DOI:
10.1039/C6RA02113A
(Paper)
RSC Adv., 2016,
6, 28055-28062
Enhanced electrochemical performance of Al–0.9Mg–1Zn–0.1Mn–0.05Bi–0.02In fabricated from commercially pure aluminum for use as the anode of alkaline batteries
Received
24th January 2016
, Accepted 8th March 2016
First published on 10th March 2016
Abstract
In this study, the electrochemical performance of new fabricated Al–0.9Mg–1Zn–0.1Mn–0.05Bi–0.02In (wt%) from commercially pure aluminum has been determined by using open circuit potential–time measurement (OCP), galvanostatic discharge, potentiodynamic polarization and electrochemical impedance spectroscopy (EIS). The results of scanning electron microscopy (SEM), energy dispersive X-ray analysis (EDX) and X-ray diffraction (XRD) reveal that the main precipitates in Al–0.9Mg–0.1Mn–0.02In and Al–0.9Mg–1Zn–0.1Mn–0.05Bi–0.02In alloys are Mg2Si and MgZn2 phases, which act as corrosion centers. The corrosion potentials of mentioned phases are more negative than that of Al. It was found that simultaneous use of alloying elements with the ability to remove the oxide film and high hydrogen over-potential could lead to activation of the alloy with lower self-corrosion rate and improved galvanic efficiency. In order to reduce the harmful effects of iron in the composition of commercially pure aluminum, manganese has been used. The results show that Al–0.9Mg–1Zn–0.1Mn–0.05Bi–0.02In is more active than the Al and Al–0.9Mg–0.1Mn–0.02In anode, and in comparison, the alloy has a lower self-corrosion rate in 4 M NaOH electrolyte. It has been observed that the galvanostatic discharge based on Al–0.9Mg–1Zn–0.1Mn–0.05Bi–0.02In offers more negative voltage and higher anodic utilization than those with Al–0.9Mg–0.1Mn–0.02In and Al.
1. Introduction
Aluminum has many interesting features such as lightness, thermal and electrical conductivity, low equivalent weight, the donating ability of three electrons per atom, and also a negative potential and high current density.1 This metal, by having a capacity per unit mass of 2.98 A h g−1, which is much higher than that of the widely used anodic material zinc 0.82 A h g−1, is a very good choice as an anode in alkaline batteries.2,3 Important points for a good anode electrode in a battery arises is as follows:4 high negative potential of OCP, minimum corrosion rate, high efficiency, battery safety and free from environmental pollution, and reasonable price compared to the other production of batteries.
However, practical applications of the Al as anode have been delayed by its low columbic efficiency arising from the high corrosion rate in alkaline media. Besides, the presence of natural oxide films on the aluminum postpones anode activation and makes the aluminum anode potential meaningfully lower than its theoretical value, thus it needs to be activated to achieve greater power density.5,6 The best way to enhance the performance of aluminum anode is to dope Al with other elements such as In, Mg, Ga, Hg and Mn, because these alloying elements can activate aluminum by eliminating or reducing the thickness of oxide films and shift the potential to a more negative value.7–11 Also, to reduce the corrosion rate of aluminum anode, high hydrogen over-potential elements such as Sn, Pb, Zn are utilized.12,13 The best mode for the alloy used in the anode is taking advantage of very high purity aluminum (>99.999% purity), because the presence of iron and silicon can lead to sharp increases in aluminum corrosion.14 However, the price of high pure aluminum is 10 to 20 times greater than the commercially pure aluminum and this prevents further use of aluminum as the anode in batteries. Two patents investigated the microstructure and electrochemical performance of Al–Mg–Mn–In alloy.15,16 Since silicon is cathodic impurity to aluminum and it can act as a center for hydrogen evolution, adding magnesium to aluminum can result in the formation of Mg2Si intermetallic compounds and minimize the harmful influence of silicon.14 It also affects on the mechanical properties of the anode by preventing the physical disintegration of the aluminum.11 The use of manganese in the composition of commercially pure aluminum anodes can reduce the corrosion rate of aluminum and reduce the harmful effects of Fe impurity by forming Al6 (Mn, Fe) in aluminum. It is noteworthy that the potential of Al6 (Mn, Fe) is almost equal to the base Al.17,18
In our pervious study, we used high pure aluminum (99.999% purity) to fabricate the alloy for using as the anode of the battery.10 The aim of this study is the feasibility and usability of commercially pure aluminum for alloying the anodes of alkaline batteries. The electrochemical and corrosion behavior of Al–Mg–Mn–In and new fabricated Al–Mg–Zn–Mn–Bi–In as anode of alkaline battery was studied in this paper.
2. Experimental
2.1. Material preparation
The nominal compositions of the alloys in this experiment are 0.9 wt% Mg–0.1 wt% Mn–0.02 wt% In and 0.9 wt% Mg–1 wt% Zn–0.1 wt% Mn–0.05 wt% Bi–0.02 wt% In and the mass balance with Al. Raw materials are magnesium and zinc ingots, indium particles (>99.9%), Al–10 wt% Mn master alloy for casting the testing alloys and commercially pure aluminum having composition in Fe 0.18, Si 0.06, Mg 0.002, Cu 0.002, Zn 0.008 (wt%) and the rest Al for casting the aforementioned anode alloys. The melting process was done in a SiC crucible using an electrical resistance furnace with a temperature range of 750–800 °C. The molten alloy was poured in a preheated cast iron mold. Manufacturing process of AgO cathode has been described in detail.10
2.2. Electrochemical measurement
The electrochemical measurements (except EIS) were carried out in a traditional three-electrode glass cell at room temperature by potentiostat/galvanostat (Autolab PGSTAT302 N). The EIS measurements were performed by a Zahner/Zennium potentiostat/galvanostat (Zahner, Germany). A saturated calomel electrode (SCE) utilized as the reference electrode and a rod of graphite was used as the counter electrode. Commercially pure aluminum and the fabricated alloys electrodes with above mentioned composition were used as a working electrode (WE). These electrodes were prepared from cubic aluminum, they were mounted in polyester, and so only their cross section (1.21, 1.70 and 1.56 cm2 for Al, Al–Mg–Mn–In and Al–Mg–Zn–Mn–Bi–In alloys, respectively) was allowed to contact the solution. The samples were ground with emery paper (400–800–1000–1500–2000 grade) and then rinsed with double distilled water. The polarization curves were measured from a cathodic potential of −2.1 V to an anodic potential of −1.0 V at a scan rate of 1 mV s−1 after OCP measurements and the EIS measurements were done at open circuit potential (OCP) in a frequency range of 100 kHz to 100 mHz with a 10 mV AC modulation amplitude. The results to the proposed equivalent circuit were fitted and evaluated using Zview electrochemical impedance spectroscopy analysis software. Galvanostatic discharge tests are performed at the current densities of 10 and 50 mA cm−2 for 1200 s. The weight of anode used up was computed from the weight loss of the anodes during galvanostatic discharge.
Anode utilization was calculated using the equation:
|
Anode utilization η = Idischargeh/(ΔWF/9.0)
| (1) |
where
η is the anode use up, %;
Idischarge is the galvanostatic discharge current of anode, (A); Δ
W is the weight loss, (g);
F is the faraday constant,
h is the time, (s).
The batteries were composed of Al, Al–Mg–Mn–In and Al–Mg–Zn–Mn–Bi–In alloys as anodes, AgO electrode as cathode and 4 M NaOH solution as electrolyte. The discharge performance of the Al–AgO batteries were investigated by means of constant current discharge tests at current densities of 50 mA cm−2 for duration of 1200 s.
2.3. Self-corrosion
The aluminum samples are insulated with epoxy resin except for the surface to be treated (1.21, 1.70 and 1.56 cm2 for Al, Al–Mg–Mn–In and Al–Mg–Zn–Mn–Bi–In alloys, respectively). The specimens were abraded with different grades of emery paper, cleaned with acetone, rinsed with double distilled water, dried by a hair blow-dryer and then immersed in 4 M NaOH solution for 60 min. The weight of the samples before and after immersion was measured accurately by a high-sensitivity balance. The sample morphology after immersion for 1 h was investigated by a XL30 ESEM PHILIPS scanning electron microscope (SEM) equipped with energy dispersive X-ray (EDX) analysis to identify various precipitates. The crystal structure of the alloy were analyzed by a Structures-APD 2000 X-ray Diffractometer using Cu Kα radiation (λ = 0.15406 nm) radiation in the 2θ range of 15–80°.
The corrosion rate was computed by the following equation:
|
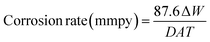 | (2) |
where Δ
W is the weight loss (mg),
D is the density (g cm
3),
A is the surface area in (cm
2),
T is the time (h) and the numeral of 87.6 is derived from the conversion of hour to year, (mg) to (g) and (cm) to (mm).
3. Results and discussion
3.1. Open-circuit potential (OCP) and potentiodynamic polarization (Tafel)
Fig. 1 and Table 1 present the OCP vs. time curves and self-corrosion rate of Al, Al–Mg–Mn–In and Al–Mg–Zn–Mn–Bi–In anodes in 4 M NaOH solution, respectively. Self-corrosion rates were acquired by weight loss measurements in 4 M NaOH solution after 1 h. As shown in Table 1, the self-corrosion rate increases in the following order: Al–Mg–Zn–Mn–Bi–In < Al–Mg–Mn–In < Al. It is clear that the open-circuit potential and self-corrosion rate of Al are higher than those of Al–Mg–Mn–In and Al–Mg–Zn–Mn–Bi–In in 4 M NaOH medium. According to Fig. 1, because of the increase in segregative phases in Al–Mg–Zn–Mn–Bi–In alloy by the addition of Bi, which plays the role of activation points, the open circuit potential of Al–Mg–Zn–Mn–Bi–In shifts toward more negative values.19 Fig. 2 and Table 2 indicate the tafel curves and related corrosion parameters of Al, Al–Mg–Mn–In and Al–Mg–Zn–Mn–Bi–In anodes in 4 M NaOH electrolyte, respectively. For Al–Mg–Zn–Mn–Bi–In, the corrosion potential is negative than that of Al–Mg–Mn–In and Al, and the corrosion current density (icorr) of Al–Mg–Zn–Mn–Bi–In is less than that of Al–Mg–Mn–In and Al in 4 M NaOH solution. Reducing the amount of βc in the case of Al–Mg–Zn–Mn–Bi–In alloy to others reveals that most of the active cathodic sites of base metal was replaced by zinc impurity which has the high over-potential for hydrogen evolution reaction.
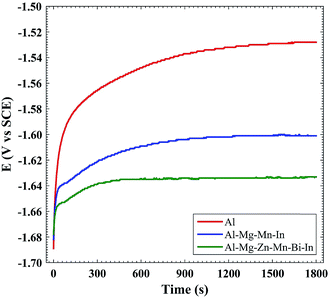 |
| Fig. 1 Open-circuit potential vs. time curves of Al, Al–Mg–Mn–In and Al–Mg–Zn–Mn–Bi–In anodes in 4 M NaOH solution. | |
Table 1 Self-corrosion rates of Al, Al–Mg–Mn–In and Al–Mg–Zn–Mn–Bi–In in 4 M NaOH solutions
Anodes |
Weight loss/mg |
Corrosion rate/mg cm−2 h−1 |
Corrosion rate/mmpy |
Al |
19.0 |
15.70 |
509.45 |
Al–Mg–Mn–In |
24.2 |
14.23 |
461.85 |
Al–Mg–Zn–Mn–Bi–In |
18.7 |
11.99 |
388.92 |
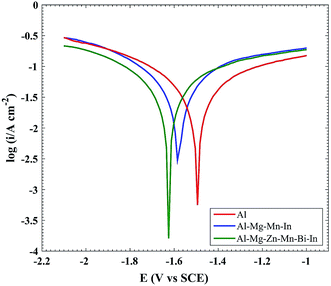 |
| Fig. 2 Potentiodynamic polarization curves for Al, Al–Mg–Mn–In and Al–Mg–Zn–Mn–Bi–In measured in 4 M NaOH solution. | |
Table 2 Corrosion parameters of Al, Al–Mg–Mn–In and Al–Mg–Zn–Mn–Bi–In in 4 M NaOH solution
Anodes |
Ecorr (V vs. SCE) |
−βc (mV dec−1) |
βa (mV dec−1) |
jcorr (mA cm−2) |
Al |
−1.501 |
794 |
1130 |
47.52 |
Al–Mg–Mn–In |
−1.598 |
681 |
906 |
37.52 |
Al–Mg–Zn–Mn–Bi–In |
−1.637 |
664 |
883 |
33.68 |
According to the Ecorr values in Table 2, the Ecorr values increase in the following order: Al–Mg–Zn–Mn–Bi–In < Al–Mg–Mn–In < Al. In addition, the corrosion current density (icorr) increases in the same order. The Ecorr of Al–Mg–Zn–Mn–Bi–In in 4 M NaOH electrolyte is negative than that of Al–Mg–Mn–In and Al, which means that Al–Mg–Zn–Mn–Bi–In in 4 M NaOH solution has the higher electrochemical activity than that of Al–Mg–Mn–In and Al. One could say that in addition to anode activation by adding Bi, presence of MgZn2 and Mg2Si particles are the main precipitates that the corrosion potential of the bulk MgZn2 is more negative than that of the others.14 The formation of Mg2Si and MgZn2 particles is demonstrated by the EDX analysis and XRD spectrum that has been followed.
3.2. Microstructure
The surface morphologies of Al, Al–Mg–Mn–In and Al–Mg–Zn–Mn–Bi–In after immersion in 4 M NaOH solution for 1 h were acquired by scanning electron microscopy (SEM) (Fig. 3). The morphology of Al–Mg–Mn–In (Fig. 3B) in 4 M NaOH solution have more pits, which is due to the presence of anodic element of magnesium and its related intermetallic particles with silicon in its structure. It is observable from Fig. 3C that the segregative phases caused by the presence of bismuth at grain boundaries have increased. The EDX analyses accompanied corresponding images of the Al–Mg–Mn–In and Al–Mg–Zn–Mn–Bi–In are shown in Fig. 4 and 5, respectively. Precipitate formed on the surface of Al–Mg–Mn–In is related to Mg2Si particles that has minimized the forming of intermetallic particles/second phase and inhibited the formation of microcells and localized attack of Al (see Fig. 4).20 The atomic ratio of these elements is about Mg
:
Si = 2
:
1. Also the EDX result and SEM image of the precipitate of Al–Mg–Zn–Mn–Bi–In are indicated in Fig. 5 which are mainly comprised of Al, Zn, and Mg elements. The atomic ratio of these elements is about Zn
:
Mg = 2
:
1. Based on the electronegativity of Zn and Mg elements, it concluded that the precipitates may be MgZn2.10,21 The mentioned intermetallic precipitates have also been confirmed by the XRD spectrum. Fig. 6 displays the XRD pattern of Al–Mg–Zn–Mn–Bi–In sample after immersion in 4 M NaOH solution for 1 h. Two phases are found indicating Mg2Si and MgZn2. Therefore, the main components in the surface of the alloy are Al, Mg2Si and MgZn2. These precipitates can act as corrosion centers that show themselves to the pitting mode. Apparently, adding alloying elements to aluminum with particular low melting point, such as In and Bi, activates surface of it by demolishing the passive layer and by distributing equally on the solid solution of aluminum or in the grain boundaries. These characteristics, accompanied by the high hydrogen evolution over-potential of the alloying elements such as Zn, make Al–Mg–Zn–Mn–Bi–In alloy appropriate for use as anode of alkaline batteries.
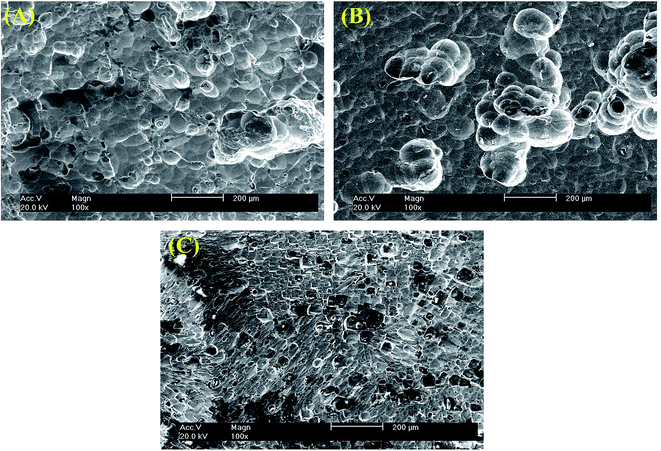 |
| Fig. 3 SEM images of anodes after 60 min immersion times in 4 M NaOH medium: (A) Al, (B) Al–Mg–Mn–In and (C) Al–Mg–Zn–Mn–Bi–In. | |
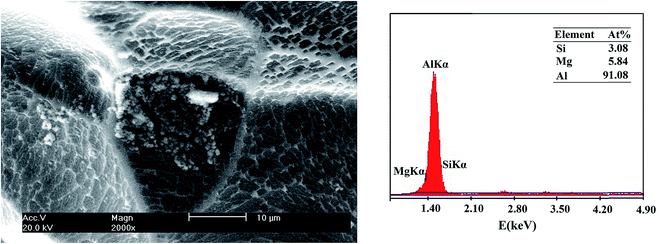 |
| Fig. 4 SEM image and corresponding EDX result of Al–Mg–Mn–In after 60 min immersion time in 4 M NaOH solution. | |
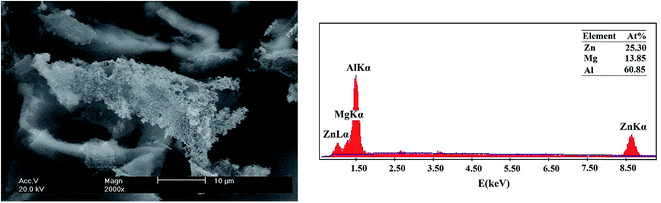 |
| Fig. 5 SEM image and corresponding EDX result of Al–Mg–Zn–Mn–Bi–In after 60 min immersion time in 4 M NaOH solution. | |
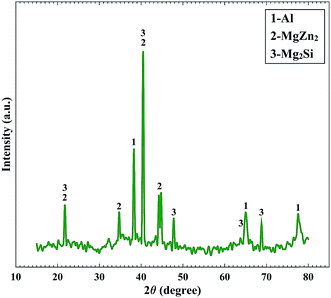 |
| Fig. 6 X-ray diffraction pattern of Al–Mg–Zn–Mn–Bi–In after 60 min immersion time in 4 M NaOH solution. | |
The improvement principle of alloying elements of Mg, Zn, Mn, Bi and In in the alloy as anode are as follows:
Mg: magnesium is an anodic impurity that leads to cathodic protection of the base aluminum and slower corrosion rate as well as preventing the silicon from forming cathodic intermetallic particles/second phase to act as hydrogen evolution centers. Also, it used to prevent physical disintegration of the aluminum anode.11
Zn: zinc is a cathodic impurity to aluminum and can decrease hydrogen evolution by increase the hydrogen evolution potential.13
Mn: manganese enhances the alloy strength by increasing the formation of fine precipitate of intermetallic phases such as Mg2Si.22 Furthermore, adding manganese to aluminum alloy not only increases tensile strength but also meaningfully improves low-cycle fatigue resistance.23
Bi: bismuth having lower melting point is enriched in the liquid of the solid–liquid interface during the solidification process, creating a segregation at the grain boundaries that activates the alloy for more negative potentials and simultaneously self-corrosion increases.24
In: indium is a cathodic impurity to aluminum and has higher over-potential to hydrogen evolution reaction. Thus, it is immediately reduced at localized sites and will be cathodically protected by the aluminum matrix and will not be dissolved during the anodic dissolution of the aluminum anode.25
3.3. Electrochemical impedance spectroscopy before and after discharge
The Nyquist plots of the anodes before and after discharge in 4 M NaOH solution and fitting of the Nyquist plots are displayed in Fig. 7. The electrical equivalent circuits for fitting the Nyquist plots are shown in Scheme 1 and the fitting values of the EIS parameters can be obtained as listed in Table 3. To achieve a satisfactory fitting results, the used capacitance element (C) shows that the double-layer capacitance is replaced by the constant phase element (CPE) Q. Acceptable description of the existence of CPE behavior and depressed loops on solid electrodes is roughly at the microscopic level, causing an inhomogeneous distribution in the double-layer capacitance. The Nyquist plots before and after galvanostatic discharge are characterized by two capacitive loops at high and low frequencies as well as two inductive loops at high and middle frequencies. Existence of inductive loop at high frequencies caused by the ohmic drop which has an imaginary region in impedance and is correlated with the potential distributions on the electrode surface.26–28 From Fig. 7A, it can be seen that charge transfer resistance (R2) decreases in the following order: Al < Al–Mg–Mn–In < Al–Mg–Zn–Mn–Bi–In. The results indicate that the resistance to corrosion increases in this order, which is well validated by potentiodynamic polarization. Compared to EIS before galvanostatic discharge, the charge transfer resistance decreases in the same order of before discharge which is beneficial for anodic efficiency of alkaline batteries. The high-frequency capacitive loop in the EIS is caused by the charge transfer reaction in the electric double layer, which in the case of Al–Mg–Zn–Mn–Bi–In after discharge, is bigger than that of Al–Mg–Mn–In and Al. One could say that the surface of Al–Mg–Zn–Mn–Bi–In is more inhibited than the others, leads to high anodic utilization during galvanostatic discharge. The middle-frequency inductive loop (L2) may be resulted from the adsorbed hydrogen of hydrogen evolution reaction. Q2 and R3,4 (R3 in case b and R4 in case a) depict corresponding parameters for the dissolution process of corrosion products. It should be noted that increasing the charge transfer resistance by adding alloying elements such as Mg and In for activating the Al and adding Mn to reduce the harmful effect of iron impurity is one side of the story, meanwhile adding Zn and Bi is the other side of the story.
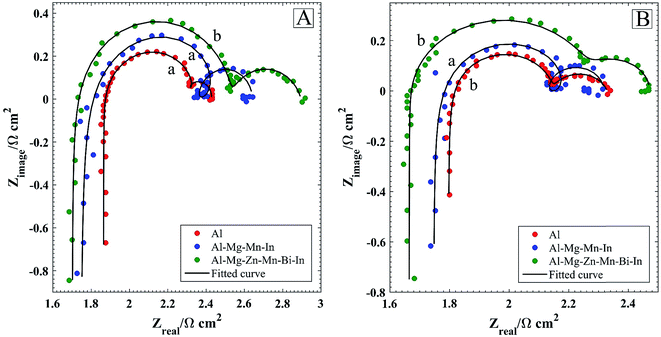 |
| Fig. 7 Nyquist plots of the Al, Al–Mg–Mn–In and Al–Mg–Zn–Mn–Bi–In before (A) and after (B) galvanostatic discharge at current densities of 50 mA cm−2 in 4 M NaOH solution and corresponding fitted curves. Lower case letters as mentioned in Scheme 1 determine corresponding compatible equivalent circuit for each Nyquist plot. | |
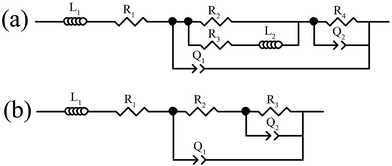 |
| Scheme 1 Equivalent circuits compatible with the Nyquist plots in Fig. 7. | |
Table 3 The area-normalized values of the elements in equivalent circuits of Scheme 1 fitted in the Nyquist plots of Fig. 7
Electrolyte |
4 M NaOH |
Al |
Al–Mg–Mn–In |
Al–Mg–Zn–Mn–Bi–In |
Before discharge |
After discharge |
Before discharge |
After discharge |
Before discharge |
After discharge |
L1 (H cm2) |
1.08 μ |
0.67 μ |
1.38 μ |
1.0 μ |
1.38 μ |
1.19 μ |
R1 (Ω cm2) |
1.86 |
1.79 |
1.74 |
1.75 |
1.70 |
1.66 |
Q1 (F cm−2) |
1.29 m |
0.28 m |
0.42 m |
0.096 m |
0.125 m |
1.05 m |
n1 (0 < n < 1) |
0.90 |
0.93 |
0.81 |
0.97 |
0.94 |
0.94 |
R2 (Ω cm2) |
0.54 |
0.35 |
0.72 |
0.45 |
0.84 |
0.62 |
L2 (H cm2) |
2.9 m |
— |
0.6 m |
0.88 m |
— |
— |
R3 (Ω cm2) |
2.30 |
0.12 |
2.37 |
2.47 |
0.36 |
0.18 |
Q2 (F cm−2) |
0.055 |
0.343 |
0.055 |
0.054 |
0.178 |
0.067 |
n2 (0 < n < 1) |
1 |
0.79 |
1 |
1 |
0.83 |
1 |
R4 (Ω cm2) |
0.13 |
— |
0.27 |
0.19 |
— |
— |
3.4. Galvanostatic and battery discharge studies
Fig. 8 displays the galvanostatic discharge curves of the Al anodes at a current densities of 10 and 50 mA cm−2. It can be seen that commercially pure Al anode has the higher extent of anodic polarization relative to others. Al–Mg–Mn–In having indium impurity to activate the anode surface, manganese to minimize the effect of iron impurity and magnesium to combine with silicon and reduce the harmful effects of it, has been able to partially overcome the loss of columbic efficiency. In the case of Al–Mg–Zn–Mn–Bi–In, the improving of the columbic efficiency is more evident especially at anodic discharge current of 10 mA cm−2. Element of zinc can control the active cathodic sites on the aluminum surface. Also, presence of bismuth results in the increase of segregative phases to more activity. Therefore, the Al–Mg–Zn–Mn–Bi–In has been able to perform better as a battery anode compared to commercially Al and Al–Mg–Mn–In in 4 M NaOH electrolyte. It is noteworthy that the potential fluctuation takes place on the galvanostatic discharge curve of Al–Mg–Zn–Mn–Bi–In may be attributed to the formation and peeling off the zincate coating over the aluminum electrode.29 Fig. 9 shows the discharge behavior of Al–AgO battery at current densities of 50 mA cm−2. Battery internal resistance causes a rapid drop in cell potential. According to reaction (3) and (4), anodic dissolution of aluminum generates Al(OH)4− ions, which activates the anode surface. The anodic process of aluminum oxidation is joined with the cathodic process of water reduction based on the reaction (5), which acts as a subsidiary cathode and reduces the columbic efficiency of the anode.10 |
Al + 3OH− → Al(OH)3↓ + 3e−
| (3) |
|
Al(OH)3 + OH− → Al(OH)4−
| (4) |
|
2H2O + 2e− → H2 + 2OH−
| (5) |
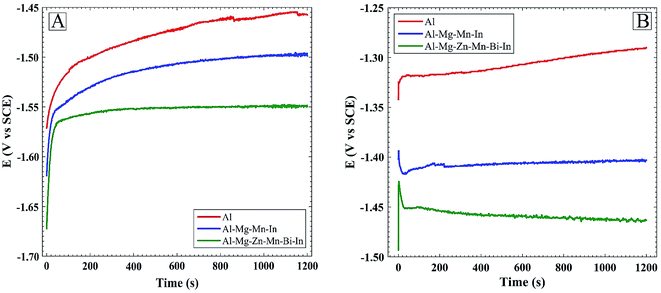 |
| Fig. 8 Galvanostatic discharge curves of Al, Al–Mg–Mn–In and Al–Mg–Zn–Mn–Bi–In anodes at current densities of 10 (A) and 50 (B) mA cm−2 in 4 M NaOH electrolyte. | |
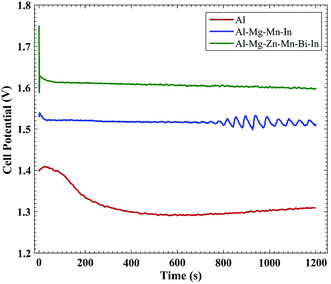 |
| Fig. 9 Discharge behavior of the Al–AgO battery at current densities of 50 mA cm−2. | |
Table 4 offers the anodic performance of the above mentioned anodes at 10 and 50 mA cm−2. As present in Table 4, the Al–Mg–Zn–Mn–Bi–In anode in 4 M NaOH electrolyte displays the highest anodic utilization compared with the others in both the anodic discharge currents.
Table 4 The galvanostatic discharge performance of Al, Al–Mg–Mn–In and Al–Mg–Zn–Mn–Bi–In at current densities of 10 and 50 mA cm−2 for 20 min in 4 M NaOH electrolyte
Current density (mA cm−2) |
Potential (V) |
Anodic utilization (%) |
Al |
Al–Mg–Mn–In |
Al–Mg–Zn–Mn–Bi–In |
Al |
Al–Mg–Mn–In |
Al–Mg–Zn–Mn–Bi–In |
10 |
−1.46 |
−1.49 |
−1.55 |
9.35 |
16.34 |
25.85 |
50 |
−1.29 |
−1.40 |
−1.46 |
55.74 |
57.12 |
60.05 |
4. Conclusions
In conclusion, we investigated the electrochemical performance of Al, Al–Mg–Mn–In and new fabricated Al–Mg–Zn–Mn–Bi–In anodes in 4 M NaOH electrolyte. The results show that compared with Al and Al–Mg–Mn–In, the Al–Mg–Zn–Mn–Bi–In anode has the higher electrochemical performance and a lower self-corrosion rate. The main precipitates in Al–Mg–Mn–In and Al–Mg–Zn–Mn–Bi–In are Mg2Si and MgZn2 phases which act as corrosion centers and their corrosion potentials are negative than that of Al which is validated by SEM images, EDX analyses and XRD spectrum. It was found that single use of alloying elements with the removal ability of the oxide film and activating capability of the anode surface is not enough and in addition, alloying elements having high hydrogen over-potential should be used. The purpose of adding manganese is to reduce the harmful effects of iron in the composition of commercially pure aluminum. Al–Mg–Zn–Mn–Bi–In anode exhibited higher electrochemical performance, less corrosion rate, improved anodic efficiency and more negative galvanostatic discharge potential than those with Al–Mg–Mn–In and Al in alkaline media.
Acknowledgements
Bu Ali Sina University are gratefully acknowledged for financial support of this work.
References
- Z. Ma and X. Li, J. Solid State Electrochem., 2011, 15, 2601–2610 CrossRef CAS.
- Q. Li and N. J. Bjerrum, J. Power Sources, 2002, 110, 1–10 CrossRef CAS.
- A. Z. Zhuk, A. E. Sheindlin, B. V. Kleymenov, E. I. Shkolnikov and M. Y. Lopatin, J. Power Sources, 2006, 157, 921–926 CrossRef CAS.
- V. Kapali, S. Venkatakrishna Iyer, V. Balaramachandran, K. B. Sarangapani, M. Ganesan, M. Anbu Kulandainathan and A. Sheik Mideen, J. Power Sources, 1992, 39, 263–269 CrossRef CAS.
- S. Gudić, J. Radošević, I. Smoljko and M. Kliškić, Electrochim. Acta, 2005, 50, 5624–5632 CrossRef.
- M. A. Amin, S. S. Abd El-Rehim, E. E. F. El-Sherbini, S. R. Mahmoud and M. N. Abbas, Electrochim. Acta, 2009, 54, 4288–4296 CrossRef CAS.
- J. Ma, J. Wen, J. Gao and Q. Li, Electrochim. Acta, 2014, 129, 69–75 CrossRef CAS.
- S. Z. El Abedin and A. O. Saleh, J. Appl. Electrochem., 2004, 34, 331–335 CrossRef.
- J. Ma, J. Wen, J. Gao and Q. Li, J. Power Sources, 2014, 253, 419–423 CrossRef CAS.
- H. Moghanni-Bavil-Olyaei and J. Arjomandi, RSC Adv., 2015, 5, 91273–91279 RSC.
- M. Nestoridi, D. Pletcher, R. J. K. Wood, S. Wang, R. L. Jones, K. R. Stokes and I. Wilcock, J. Power Sources, 2008, 178, 445–455 CrossRef CAS.
- A. Sheik Mideen, M. Ganesan, M. Anbukulandainathan, K. B. Sarangapani, V. Balaramachandran, V. Kapali and S. Venkatakrishna Iyer, J. Power Sources, 1989, 27, 235–244 CrossRef.
- Y. Tang, L. Lu, H. W. Roesky, L. Wang and B. Huang, J. Power Sources, 2004, 138, 313–318 CrossRef CAS.
- J. Wen, J. He and X. Lu, Corros. Sci., 2011, 53, 3861–3865 CrossRef CAS.
- P. W. Jeffrey, W. Halliop and F. N. Smith, Aluminium anode alloy, US Pat. 4,751,086, 1988.
- P. W. Jeffrey, W. Halliop and F. N. Smith, Aluminium anode alloy, EP 0,209,402, 1987.
- J. Ma and J. Wen, J. Alloys Compd., 2010, 496, 110–115 CrossRef CAS.
- Z. Sun, H. Lu, L. Fan, Q. Hong, J. Leng and C. Chen, J. Electrochem. Soc., 2015, 162, A2116–A2122 CrossRef CAS.
- J. W. Gao, J. B. Wen and J. G. He, The research of electrochemical properties of Al-Mg-Sn-Ga-Bi alloys in alkaline electrolyte Advanced Materials Research, 2013, vol. 800, pp 488–491 Search PubMed.
- S. Khireche, D. Boughrara, A. Kadri, L. Hamadou and N. Benbrahim, Corros. Sci., 2014, 87, 504–516 CrossRef CAS.
- M. A. Jingling, W. Jiuba, L. I. Gengxin and X. V. Chunhua, Corros. Sci., 2010, 52, 534–539 CrossRef.
- G. Mrówka-Nowotnik, J. Sieniawski and A. Nowotnik, J. Achiev. Mater. Manuf. Eng., 2009, 32, 162–170 Search PubMed.
- S. W. Nam and D. H. Lee, Met. Mater. Int., 2000, 6, 13–16 CrossRef CAS.
- J.-g. He, J.-b. Wen, X.-d. Li, G.-w. Wang and C.-h. Xu, Trans. Nonferrous Met. Soc. China, 2011, 21, 1580–1586 CrossRef CAS.
- M. Reboul, P. Gimenez and J. Rameau, Corrosion, 1984, 40, 366–371 CrossRef CAS.
- V. M.-W. Huang, V. Vivier, I. Frateur, M. E. Orazem and B. Tribollet, J. Electrochem. Soc., 2007, 154, C89–C98 CrossRef CAS.
- V. M.-W. Huang, V. Vivier, M. E. Orazem, N. Pébère and B. Tribollet, J. Electrochem. Soc., 2007, 154, C81–C88 CrossRef CAS.
- V. M.-W. Huang, V. Vivier, M. E. Orazem, N. Pébère and B. Tribollet, J. Electrochem. Soc., 2007, 154, C99–C107 CrossRef CAS.
- J.-B. Wang, J.-M. Wang, H.-B. Shao, J.-Q. Zhang and C.-N. Cao, J. Appl. Electrochem., 2007, 37, 753–758 CrossRef CAS.
|
This journal is © The Royal Society of Chemistry 2016 |