DOI:
10.1039/C6RA01948J
(Paper)
RSC Adv., 2016,
6, 32484-32492
Novel organotin complexes derived from 2,2′-selenodiacetic acid: synthesis and biological evaluation†
Received
22nd January 2016
, Accepted 9th March 2016
First published on 11th March 2016
Abstract
The reactions of 2,2′-selenodiacetic acid and the corresponding organotin(IV) chloride with sodium ethoxide in ethanol, or via a solvothermal synthetic route, have afforded four organotin polymers, namely, [(Me3Sn)2Se(CH2COO)2]n (1), [(Me2Sn)2Se(CH2COO)2(μ3-O)]n (2), [(Bu3Sn)2Se(CH2COO)2]n (3), and [(Bu2Sn)Se(CH2COO)2]n (4). All the complexes were fully characterized by elemental analysis, FT-IR, NMR (1H, 13C, and 119Sn) spectroscopy and single crystal X-ray diffraction analysis. The structural analysis reveals that complex 1 is a 2D-polymer containing interconnected 24-membered (Sn4O8C10Se2) macrocyclic rings. Unlike complex 1, the 2D polymer structure of complex 2 is made up of the typical ladder tetraorganodistannoxane unit containing 32-membered (Sn4O8C16Se4) macrocyclic rings. Complex 3 is a 3D corrugated polymeric structure in which the deprotonated dicarboxylic acid acts as a tetradentate ligand by four oxygen atoms. Complex 4 is a 1D zigzag coordination polymer and the tin coordination geometry is described as skewed-trapezoidal bipyramidal. In particular, preliminary cytotoxic assessments of the involvement of complex 4 in the apoptotic death of MDA cells were conducted, and the results revealed that the antiproliferative and proapoptotic effect of complex 4 in MDA cells prominently contributed to the overload of intracellular ROS levels and the dysfunctional depolarization of mitochondrial membranes.
1. Introduction
Organotin derivatives have recently attracted considerable attention for their particular spatial constructions,1–4 as well as their interesting biological properties.5–8 Quite a number of tin-based complexes have been reported for their anti-proliferative properties against a variety of cell lines9–11 and it is heartening that they can exhibit attractive properties, such as lower general toxicity, better body clearance and fewer side effects, than platinum drugs.12 Most importantly, the studies have found that cancer cells do not develop resistance against organotin complexes, which is well established for cisplatin and its analogs.13 Among these complexes, organotin(IV) carboxylates have been especially investigated for their antitumor potential, owing to their better hydrolytic stability compared to organotin(IV) halides.14–16 In view of the increasing interest, it is necessary to design and synthesize novel organotin carboxylates for further biological and preclinical assessment.
Selenium is closely related to human health as a trace element. Selenium complexes, particularly organoselenium, have been shown to exhibit promising chemotherapeutic properties,17–19 which have caused them to become hot spots in the studies of tumor prevention and therapy. 2,2′-Selenodiacetic acid is an interesting ligand with a long history. It contains two terminal carboxylic acid groups analogous to dicarboxylic acids, but possesses the bio-element selenium. Furthermore, the compound is only weakly toxic as compared to many other seleno-organic compounds and it has been shown to have a curative effect for many diseases, such as Keshan and Kashin-Beck, caused by low Se in the natural environment.20
An intriguing idea is to combine some of these biologically active organoselenium ligands with organotin fragments, which may show similar or better activity and less severe side effects than that of the native drugs. We recently started a study on the reactions of 2,2′-selenodiacetic acid with organotin chloride. As a result, four novel organotin polymers were successfully prepared. In this study, the syntheses and structural characterizations of these new complexes are reported. In addition, the antiproliferative effects of complexes 1–4 in MDA cells are tested and particularly the proapoptotic induction effects of complex 4 in MDA cells are revealed.
2. Results and discussion
2.1. Syntheses of complexes 1–4
According to our designed synthetic route for the preparation of the complexes 1–4 (Scheme 1), the starting material 2,2′-selenodiacetic acid Se(CH2COOH)2 is prepared first. It can be obtained conveniently from chloroacetic acid and an in situ solution of Se2−, formed from grey selenium and a double excess of NaBH4 in a 1
:
1 THF/H2O mixture.21 Complexes 1, 3 and 4 can be prepared in good yield by treatment of 2,2′-selenodiacetic acid and the corresponding triorganotin(IV) chloride or diorganotin(IV) dichloride with sodium ethoxide in ethanol. Complex 2 is prepared via a solvothermal synthetic route involving the reaction of trimethyltin chloride and 2,2′-selenodiacetic acid with KOH in CH3OH and H2O (v/v = 1
:
5). Complexes 1–4 are stable in air and in common solvents such as chloroform, acetonitrile and tetrahydrofuran. Complex crystals suitable for X-ray analysis were obtained by slow evaporation of diethyl ether or methanol.
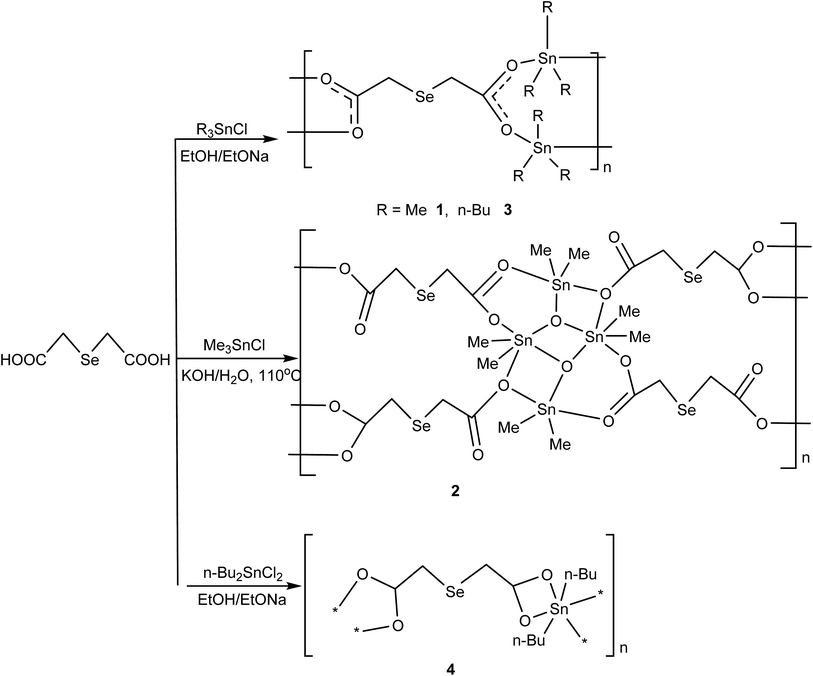 |
| Scheme 1 The synthetic procedures for obtaining complexes 1–4. | |
2.2. IR spectra
For complexes 1–4, upon metal-coordination, the disappearance of νO–H indicates the deprotonation of the OH and its subsequent coordination to the central tin atom. The strong absorptions appearing at about 452–476 cm−1 in the respective spectra of complexes 1–4, which are absent in the free ligand, are assigned to the Sn–O stretching vibration modes. Two intense bands for the asymmetric and symmetric stretching vibrations of the COO functions appear in the regions of 1583–1635 cm−1 and 1359–1429 cm−1, respectively, and the difference between the wavenumber for νas(COO) and νs(COO) allows one to distinguish the coordination modes of the ligand.4 It is greatly believed that if the carboxylic group coordinates to the tin atom in a bidentate manner, the difference between the Δ(νas − νs) is lower than for the corresponding sodium salts of the carboxylic acid ligand, and if the value of Δ(νas − νs) is much greater than the corresponding sodium salts, the carboxylic group is coordinated to the tin atom in a monodentate mode.22 Obviously, the Δν[νas(COO) − νs(COO)] values (206 cm−1 for 1, 206 cm−1 for 3, and 212 cm−1 for 4) are slightly less than for the ligand (226 cm−1), indicating bidentate coordination modes, whereas the Δν[νas(COO) − νs(COO)] values of 241, 217 cm−1 for 2, reveal monodentate and bidentate modes for the carboxylic ligand, which are consistent with a number of organotin(IV)–oxygen derivatives.4,23 For complex 2, the strong band in the 684 cm−1 region is assigned to the Sn–O–Sn stretching vibration, which is typically found for diorganotin dicarboxylates having ladder tetraorganodistannoxane geometries.24
2.3. NMR spectra
All compounds obtained satisfactory NMR spectra. In the 1H NMR spectra of 1–4, the peak for the carboxylic acid hydroxyl groups at δ = 11.5 ppm is absent due to the formation of the carboxylate group. For the CH3 groups in 1–4, the signals are observed at δ = 0.59 and 0.57 ppm for 1,2, whereas in 3,4, δ = 0.91 and 0.92 ppm. The 13C NMR spectra for complexes 1–4 obtained signals in the range of δ = 175.8–180.6 ppm for the COO− groups, which are in the region typically observed for organotin carboxylates.25 These signals are low-field shifted when compared to the starting dicarboxylic acids. Obviously, the shift is a consequence of an electron density transfer from the ligand to the tin atoms.
The 119Sn NMR spectrum of complex 1 obtained only one signal at δ = 150.2 ppm and complex 3 showed two signals at 119.5 and 158.6 ppm, typical of a four-coordinated tin atom, suggesting that the intramolecular O → Sn interaction of 1 and 3 probably does not survive in solution, which is quite common in the organotin(IV) carboxylates.26,27 Complex 2 obtained two different signals (δ = −155, 3.1), indicating the presence of different species with different tin coordination environments; they were observed in the typical range for five- and four-coordinate tin. Complex 4 showed only one signal at −137.5 ppm. These also indicate the cleavage of the O → Sn bond in solution, for 2 and 4.28
2.4. X-ray crystallography
2.4.1. X-ray crystallography of complex 1. A perspective view of the repeating unit and 2D polymeric structures of complex 1 are shown in Fig. 1. Selected bond lengths (Å) and angles (°) are listed in Table S1.† In the solid-state, [(Me3Sn)2Se(CH2COO)2]n (1) is a 2D-polymer containing interconnected 24-membered (Sn4O8C10Se2) macrocyclic rings. The tri-methyltin(IV) groups are linked by a carboxylate of each ligand in turn employing its two carboxylic groups to coordinate to four tin centers. Thus, four ligands are linked by four metal centers into a 24-membered macrocycle, which is further linked to eight nearest-neighbor tin centers by a lattice 2D network (Fig. 1b). Within each macrocyclic ring, two types of inter-tin distances are found (8.294 and 10.023 Å). All the tin atoms possess the same coordination environment. The coordination about the tin atom is only slightly distorted from the regular trigonal bipyramidal geometry. The Sn–O distances [Sn(1)–O(1) 2.414(14) Å, Sn(1)–O(4)#1 2.181(14) Å, Sn(2)–O(2)#2 2.193(14) Å, Sn(2)–O(3) 2.459(15) Å (symmetry code: #1 x, y + 1, z #2 x + 1, y, z)] all lie between the covalent bond length and the van der Waals radii.29 The O–Sn–O angles [O(4)#1–Sn(1)–O(1) 170.3(6), O(2)#2–Sn(2)–O(3) 173.1(5)] are close to linear arrangement. The sum of the angles subtended at the tin atoms in the equatorial plane is 356.8° for Sn(1) and 357.1° for Sn(2).
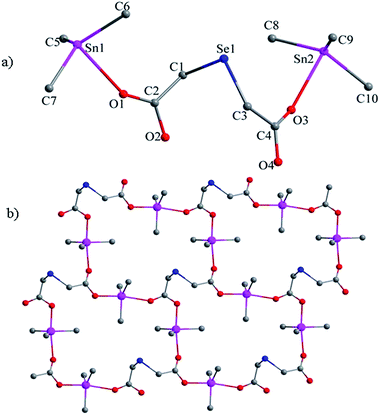 |
| Fig. 1 Fragments of the crystal structure of complex 1 showing (a) the repeating unit and (b) 2D polymeric structure. | |
2.4.2. X-ray crystallography of complex 2. Compound 2 is also a 2D coordination polymer, although its structural architecture is different from that of 1 (Fig. 2). In contrast to 1, where Sn-methyl cleavage occurs and the organotin polymers formed are made up of the typical ladder tetraorganodistannoxane unit and bridging deprotonated dicarboxylic ligand, this ladder tetraorganodistannoxane unit is a tetranuclear, centrosymmetric dimeric structure with a central planar Sn2O2 four-membered ring and two peripheral Me2Sn units. Each bridging oxygen atom in the Sn2O2 ring is attached to three μ3-O type Me2Sn units. The geometries of all the tin atoms in complex 2 can be classified into two types as follows: penta-coordinated tin Sn(1) and hexa-coordinated tin Sn(2). Each of the penta-coordinated tin atoms Sn(1) forms three short Sn–O bonds with three oxygen atoms, two from the dicarboxylate groups and the other from μ3-O. Together with the two bonds from Sn–CH3 groups, the Sn(1) atom can be viewed as a slightly distorted trigonal bipyramid with the axial site occupied by the O(1) and O(3) atoms and O(1)–Sn(1)–O(3) 169.17(13)° (see Table S2†). Unlike Sn(1), the hexa-coordinated tin atoms Sn(2), form four short Sn–O bonds with four oxygen atoms: two from the μ3-O and the other two from two different dicarboxylate groups. Together with the two Sn–CH3 groups, Sn(1) forms an octahedral geometry. Thus, each deprotonated dicarboxylic acid acts as a tridentate ligand connecting two adjacent ladders to obtain to a 2D network polymer containing a 32-membered (Sn4O8C16Se4) macrocyclic ring (Fig. 2b). Interestingly, on account of the serration of the line chain dicarboxylic ligand, a novel “stair” style of the 2D polymer structure is presented (Fig. 2c).
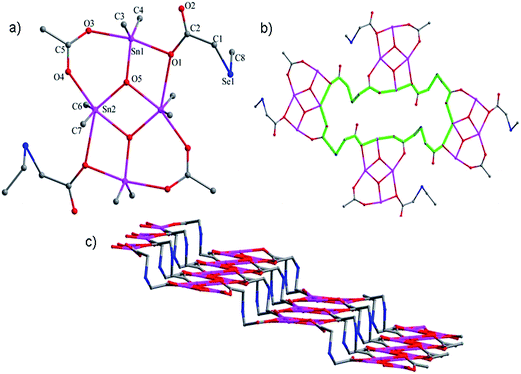 |
| Fig. 2 Fragments of the crystal structure of complex 2 showing (a) the repeating unit, (b) 32-membered macrocyclic repeating unit and (c) “stair” style of the 2D polymer structure (methyl groups have been omitted for clarity). | |
2.4.3. X-ray crystallography of complex 3. Unlike complex 1, complex 3 is a 3D corrugated polymeric structure, although the coordination modes of the central Sn atoms are the same as 1 (Fig. 3). Each dicarboxylic acid is connected to four tin atoms as a tetradentate ligand by four oxygen atoms. All the tin atoms are also five-coordinate with distorted trigonal bipyramidal geometry. Looking through the a direction, six tin atoms [Sn(1), Sn(1A), Sn(2), Sn(2A), Sn(4), Sn(4A)] together with six ligands construct a 40-membered corrugated lattice and it is further linked to six nearest-neighbor lattices to obtain to a 2D corrugated sheet. These sheets are further interlinked into forming a 3D corrugated framework by intermolecular C
O → Sn coordination interactions, thus obtaining another 48-membered corrugated channel that contains six tin atoms [Sn(1), Sn(1A), Sn(2), Sn(2A), Sn(3), and Sn(3A)]. All the Sn–O bond lengths lie between the covalent bond length and the van der Waals radii of the two atoms. The axial angles of the trigonal bipyramid [Sn(1) 175.2(4)°, Sn(2) 174.7(4)°, Sn(3) 176.0(5)°, Sn(4) 171.4(5)°] (see Table S3†) are all slightly deviated from the linear arrangement.
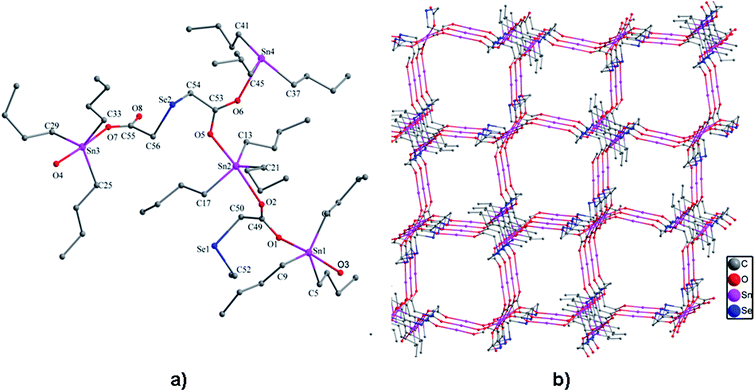 |
| Fig. 3 Fragments of the crystal structure of complex 3 showing (a) the repeating unit and (b) 3D corrugated framework (n-butyl groups have been omitted for clarity). | |
2.4.4. X-ray crystallography of complex 4. In the solid-state, [Bu2SnSe(CH2COO)2]n (4) is a 1D zigzag coordination polymer, in which the six-coordinate tin atoms are bound to four oxygen atoms from two different carboxylate groups and two carbon atoms from the n-butyl substituents (Fig. 4). The Sn coordination geometry is described as skewed-trapezoidal bipyramidal, in which the basal plane is formed by four oxygen atoms and the apical positions are occupied by the carbon atoms. The carboxylate groups chelate in an anisobidentate manner. Take Sn(1) for example, the Sn(1)–O(1) 2.146(10) Å and Sn(1)–O(5) 2.153(10) Å approach the sum of the covalent radii of Sn and O(2.13 Å). Moreover, the bond Sn(1)–O(2) 2.541(10) Å and Sn(1)–O(6) 2.504(11) Å (Table S4†) are recognized as coordinate bonds. The sum of angles subtended at the Sn(1) atom is 361.2°, showing that the atoms Sn(1), O(1), O(2), O(5) and O(6) are almost in the same plane.
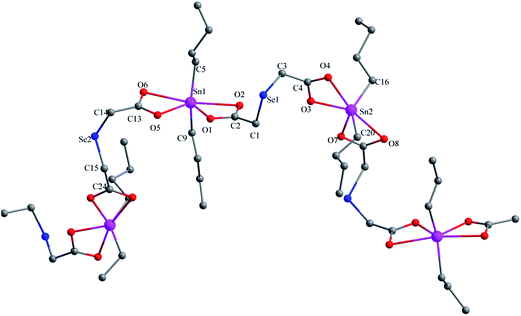 |
| Fig. 4 The 1D zigzag chain structure of 4. | |
2.5. In vitro cytotoxicity activity of complex 4
2.5.1. Complexes 1–4 inhibited proliferation of MDA cells. The in vitro antiproliferative effects of 1–4 against MDA have been evaluated by MTT-based assays. A comparison of inhibition rates for complexes 1–4 is given in Table 1. The IC50 values were calculated from the dose survival curves obtained after 24 h of drug treatment in the MTT test. As can be observed from Table 1, those complexes with R = Me (1 and 2) exhibit almost no activity against MDA, whereas complexes with R = Bu (3 and 4) exhibit strong activity, and complex 4 shows higher in vitro cytotoxic activity than 3; the IC50 values for 3 and 4 against MDA are 0.59 μmol L−1 and 0.35 μmol L−1, respectively. The inhibition effects of complex 4 on the MDA cell line at different concentrations are successively shown in Fig. 5a. It was found that there was an obvious agreement between cytotoxicity and the dosage at which complex 4 induced a dose-responsive decrease in MDA cell viability, compared to the overall cell survival rate that remained considerably controlled during a 24 hour treatment.
Table 1 IC50 (μM) of complexes 1–4 against MDA cell line
Complex |
IC50 |
MDA |
1 |
>100 |
2 |
>100 |
3 |
0.59 |
4 |
0.35 |
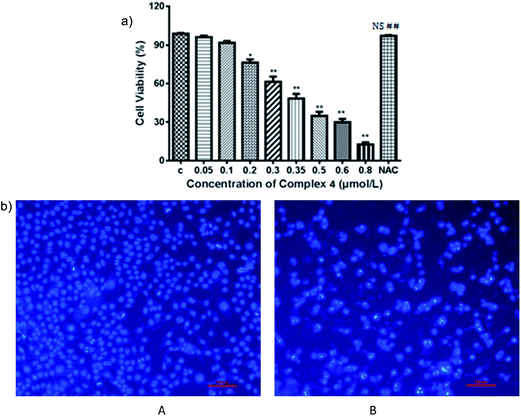 |
| Fig. 5 (a) Complex 4 reduces MDA viability. The concentration-response effect of complex treatment and treatment with 0.35 μmol L−1 of complex 4 in the presence (15 mM) or absence of NAC for 24 h on cellular viability, expressed as a percentage of MTT value. The data represent the mean ± SEM of 6 independent experiments. *p < 0.05 and **p < 0.01, as compared to the control. ##p < 0.01, as compared to 0.35 μmol L−1 of complex 4-treated cells; ns, not significant as compared to control. (b) Effect of complex 4 on chromatin condensation in MDA cells. Representative photomicrographs of DAPI stained cells observed under an inverted fluorescent microscope after incubation with 0.35 μmol L−1 of complex 4 (B) and DMSO ((A)-control). | |
2.5.2. Complex 4 induced morphologic alteration and apoptosis of MDA cells. The effect of complex 4 on nuclear morphology was evaluated by employing DAPI staining; the DNA damaging effect of complex 4 was demonstrated by the morphological inspection of the induction of nuclei condensation. As shown in Fig. 5b, the nuclei of the control group cells were slightly round and uniform; however, the nuclei of the treated cells (0.35 μmol L−1 complex 4) were smeared, fragmented and condensed. Extensive fragmentation of DNA can lead to the irreversible activation of apoptosis,30,31 although cancer cells have the capability to initiate defensive DNA damage repair mechanisms to escape lethal damage in response to chemotherapy.32,33 The observation suggests that the cells suffered from apoptotic cell death initiated by complex 4.
2.5.3. Complex 4 induced accumulation of intracellular ROS and induced depolarization of mitochondrial membranes of MDA cells. After confirming the apoptotic effect of complex 4 on MDA cells, it was necessary to determine the apoptotic inducer within cells. It is crucial to figure out the definite molecular targets for bioactive compounds in developing pharmaceutical drugs, because it provides information for the progress of highly specialized and accurate future clinical trials.34,35 A deeper investigation of the complex 4 apoptotic targets was necessary to further reveal the cytotoxic mechanism in the current study.One of the molecular mechanisms that have received close attention in the incessant combat on cancer is the relationship between the accumulation of reactive oxygen species (ROS) and mitochondrial dysfunction.35,36 ROS manipulate cancer cell survival, growth, and differentiation and findings have suggested that the overproduction of ROS can induce apoptosis in cancer cells.37 Accumulating evidence suggests that ROS-regulated apoptosis is a promising strategy for anticancer drugs. Many anticancer drugs target cells, at least in part, by generating high levels of intracellular ROS. A number of reports have indicated that agents targeting ROS metabolism can kill the cancer cells by raising the level of ROS above a toxic threshold. Inducing ROS generation is expected to increase the sensitivity of cancer cells to apoptotic stimuli in complex 4 treated cancer therapy. Therefore, to verify the fact and to uncover the regulatory mechanisms involved in complex 4-induced apoptosis by invoking ROS mediation, the levels of ROS were detected in MDA cells treated with complexes of various concentrations over indicated periods of time. Concentration- and time-dependent increases in ROS generation were monitored using the carboxy derivative of fluorescein, 2′,7′-dichlorodihydrofluorescein diacetate (DCFH-DA), a ROS-sensitive fluorescent probe; complex 4 treatment (0.35 μmol L−1) for 24 h resulted in considerable bright green fluorescent dots compared to the vehicle cell group in dark field. To clarify whether ROS generation is the primary regulator in complex 4-induced apoptosis, MDA cells were pretreated with NAC, a ROS scavenger, to suppress the intracellular oxidative stress. NAC pretreatment almost totally rescued the cells viability from a lethal case. These results indicate that blocking oxidative stress by NAC resulted in distinct MDA cell survival from apoptotic events induced by complex 4, as shown in Fig. 6. Investigation suggested that intracellular ROS production is an upstream factor for MDA cells in complex 4 chemotherapeutics, which implies that ROS mediated by complex 4 being a potential biofunction to block the survival pathways and induce the apoptotic pathways. Therefore, targeting ROS associated pathways can act as an attractive therapeutic approach of complex 4 against cancer.
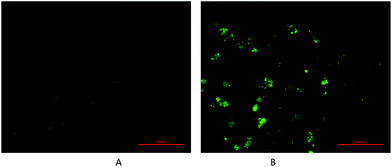 |
| Fig. 6 Assessment of the level of intracellular ROS generation. Cells were treated with 0.35 μmol L−1 of complex 4 (B) and vehicle (A) for 24 h and then were incubated with 10 μmol L−1 of DCFH-DA, an oxidation-sensitive fluorescent probe, for 30 min in the dark after indicated treatment with complex 4. Fluorescence images of ROS generation in living cells after complex 4 treatment. The levels of intracellular ROS were monitored by inverted microscope. | |
ROS are tightly coupled with mitochondria, which are not only the origin of ROS but also the targets of ROS.38 Excessive intracellular ROS production has been shown to induce apoptosis by depolarization of the mitochondrial membrane. Sustained oxidative stress facilitates mitochondrial permeability transition pore opening, which interrupts glycolysis and oxidative phosphorylation for ATP production.39 Mitochondrial dysfunction is associated with ROS-mediated cell death induced by chemotherapeutic agents.40–42 Apoptosis through a mitochondrial-mediated pathway can be assessed by performing MMP assay using the mitochondria-sensitive probe, JC-1. To further explore the mechanism of complex 4-induced apoptosis and to investigate whether mitochondria disruption is involved in apoptotic events induced by complex 4, MMP in response to complex 4 treatment was monitored by the aggregate status of the JC-1 probe under fluorescence microscopy. The results showed that complex 4 caused a highly visible alteration of merged fluorescence of treated cells, compared to intact cells. As shown in Fig. 7. These findings support the fact that complex 4 instigate early apoptotic death by inducing the collapse of MMP.
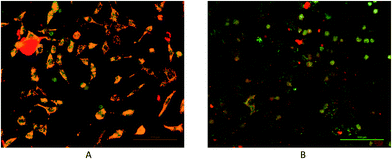 |
| Fig. 7 Effects of complex 4 on mitochondrial membrane potential. Cells were pre-treated with 0.35 μmol L−1 of complex 4 (B) and vehicle (A) for 24 h. Merge photomicrographs of JC-1 staining in MDA cells treated with or without apoptotic concentrations of complex 4. | |
3. Conclusions
In summary, four new organotin polymers based on 2,2′-selenodiacetic acid have been synthesized and fully characterized. X-ray crystal diffraction analysis shows that the structural diversities are greatly affected by the reaction conditions and the organo-ligand R bound to tin atoms. Most remarkably, preliminarily in vitro cytotoxic assessments of complex 4 demonstrate that it possesses good potential biological activity. Further studies on antitumor mechanisms are in progress in this laboratory.
4. Experimental details
4.1. Materials and measurements
Trimethyltin chloride, tri-n-butyltin chloride, and dibutyltin dichloride were commercially available and they were used without purification. 2,2′-Selenodiacetic acid was prepared according to previously reported methods.20,21 The melting points were obtained with an X-4 digital micro melting-point apparatus and were uncorrected. Infrared-spectra were obtained on a Nicolet-5700 spectrophotometer using KBr discs. 1H, 13C and 119Sn NMR spectra were obtained on a Varian mercury Plus400 spectrometer operating at 400, 100.6 and 149.2 MHz, respectively. The spectra were obtained at room temperature (298 K) unless otherwise specified; the 13C spectra were broadband proton decoupled. The chemical shifts are reported in ppm with respect to the references and are stated relative to external tetramethylsilane (TMS) for 1H and 13C NMR and Me4Sn for 119Sn NMR. Elemental analyses (C, H) were performed with a PE-2400II apparatus.
4.2. Syntheses of complexes 1–4
4.2.1. Synthesis of complex 1. The reaction was carried out under nitrogen atmosphere. 2,2′-Selenodiacetic acid (0.197 g, 1 mmol) and sodium ethoxide (0.136 g, 2 mmol) were added to a solution of ethanol (30 mL) in a Schlenk flask and stirred for 30 min. Trimethyltin chloride (0.398 g, 2 mmol) was added and the reaction mixture was stirred for 12 h at room temperature. The solution was filtered and the solvent of the filtrate was gradually removed by evaporation under vacuum, until a solid product was obtained. The solid was recrystallized from methanol and a transparent colorless crystal was formed. Yield: 75%. M.P.: 116–117 °C. Anal. calc. for C10H22O4SeSn2: C, 22.98; H, 4.24%. Found: C, 23.21; H, 4.37%. IR (KBr, cm−1): νas(COO), 1635; νs(COO), 1429; ν(Sn–C), 568; ν(Sn–O), 442. 1H NMR (CDCl3, ppm): δ = 3.40 (s, SeCH2), 0.59 (t, CH3, 2JSn–H = 57.2 Hz). 13C NMR (CDCl3, ppm): δ = 175.8 (COO); 24.5 (SeCH2); −2.3 (Sn–CH3, 1JSn–C = 207 Hz). 119Sn NMR (CDCl3, ppm): 150.2.
4.2.2. Synthesis of complex 2. The reaction was carried out in a sealed Teflon-lined stainless steel autoclave. A mixture of 2,2′-selenodiacetic acid (0.197 g, 1 mmol), KOH (0.112 g, 2 mmol) and trimethyltin chloride (0.398 g, 2 mmol) in a mixture of H2O and CH3OH (v/v = 5
:
1) was heated under solvothermal conditions (110 °C) for three days. After cooling to room temperature, colorless crystals were collected and washed with hexane. Yield: 62%. M.P.: 196–197 °C. Anal. calc. for C8H16O5SeSn2: C, 18.89; H, 3.17%. Found: C, 19.11; H, 3.37%. IR (KBr, cm−1): νas(COO), 1585; νs(COO), 1368; ν(Sn–O–Sn), 684, ν(Sn–C), 552; ν(Sn–O), 449. 1H NMR (CDCl3, ppm): δ = 3.40 (s, SeCH2), 0.57 (t, CH3, 2JSn–H = 56.8 Hz). 13C NMR (CDCl3, ppm): δ = 176.1 (COO); 24.8 (SeCH2); −2.4 (Sn–CH3, 1JSn–C = 210 Hz). 119Sn NMR (CDCl3, ppm): −155, 3.1.
4.2.3. Synthesis of complex 3. The procedure is similar to that of complex 1. The 2,2′-selenodiacetic acid (0.197 g, 1 mmol), sodium ethoxide (0.136 g, 2 mmol) and tri-n-butyltin chloride (0.651 g, 2 mmol) were reacted for 12 h at room temperature and recrystallized from diethyl ether; a transparent colorless crystal was formed. Yield: 65%. M.P.: 53–54 °C. Anal. calc. for C56H116O8Se2Sn4: C, 43.39; H, 7.54%. Found: C, 43.55; H, 7.81%. IR (KBr, cm−1): νas(COO), 1583; νs(COO), 1377; ν(Sn–C), 546; ν(Sn–O), 456. 1H NMR (CDCl3, ppm): δ = 3.39 (s, SeCH2), 1.24–1.60 (m, CH2CH2CH2), 0.91 (t, 2JSn–H = 14.5 Hz, CH3). 13C NMR (CDCl3, ppm): δ = 176.3 (COO); 24.8 (SeCH2); 27.7 [αCH2, 1J(119Sn–13C) 417 Hz], 26.8 [βCH2, 2J(119Sn–13C) 19 Hz], 17.3 [γCH2, 3J(119Sn–13C) 98 Hz], 13.6 (CH3). 119Sn NMR (CDCl3, ppm): 119.5, 158.6.
4.2.4. Synthesis of complex 4. The procedure is similar to that of complex 1. The 2,2′-selenodiacetic acid (0.197 g, 1 mmol), sodium ethoxide (0.136 g, 2 mmol) and dibutyltin dichloride (0.303 g, 1 mmol) were reacted for 12 h at room temperature and recrystallized from diethyl ether; a transparent colorless crystal was formed. Yield: 80%. M.P.: 108–109 °C. Anal. calc. for C24H44O8Se2Sn2: C, 33.68; H, 5.18%. Found: C, 33.57; H, 5.46%. IR (KBr, cm−1): νas(COO), 1636, 1571; νs(COO), 1394, 1359; ν(Sn–C), 544; ν(Sn–O), 456. 1H NMR (CDCl3, ppm): δ = 3.45 (s, SeCH2), 1.36–1.73 (m, CH2CH2CH2), 0.92 (t, 2JSn–H = 14.4 Hz, CH3). 13C NMR (CDCl3, ppm): δ = 180.6 (COO); 23.7 (SeCH2); 26.5(αCH2, 1J(119Sn–13C) 606 Hz), 25.3 (βCH2), 16.8 (γCH2), 13.5(CH3). 119Sn NMR (CDCl3, ppm): −137.5.
4.3. X-ray crystallographic studies
Single-crystal X-ray diffraction data were collected on a Bruker SMART-1000 CCD diffractometer with graphite-monochromated Mo Kα (λ = 0.71073 Å) radiation at room temperature. The structures were solved by direct methods and refined by the full-matrix least-squares methods on F2 using the SHELX-97 program.43 All non-hydrogen atoms were refined with anisotropic displacement parameters. The hydrogen atoms attached to carbon atoms were generated geometrically and refined using riding mode. Crystal data and experimental details of the structure determinations are listed in Table 2.
Table 2 Crystal data and structure refinement parameters for complexes 1–4
Complex |
1 |
2 |
3 |
4 |
Empirical formula |
C10H22O4SeSn2 |
C8H16O5SeSn2 |
C56H116O8Se2Sn4 |
C24H44O8Se2Sn4 |
Formula weight |
522.62 |
508.55 |
1550.17 |
855.89 |
Wavelength (Å) |
0.71073 |
0.71073 |
0.71073 |
0.71073 |
Crystal system |
Triclinic |
Monoclinic |
Orthorhombic |
Monoclinic |
Space group |
P![[1 with combining macron]](https://www.rsc.org/images/entities/char_0031_0304.gif) |
P2(1)/n |
Pbca |
P2(1)/c |
a (Å) |
8.2944(6) |
7.5627(6) |
16.7533(18) |
14.2606(12) |
b (Å) |
10.0429(7) |
11.8111(11) |
29.152(3) |
25.221(2) |
c (Å) |
11.4961(9) |
16.3455(15) |
31.755(3) |
10.0413(8) |
α (°) |
99.026(2) |
90 |
90 |
90 |
β (°) |
100.060(2) |
96.024(2) |
90 |
105.678(2) |
γ (°) |
111.211(3) |
90 |
90 |
90 |
V (Å3) |
853.38(11) |
1452.0(2) |
15 509(3) |
3477.2(5) |
Z |
2 |
5 |
8 |
4 |
Dcalc (Mg m−3) |
2.034 |
2.326 |
1.328 |
1.635 |
μ (mm−1) |
5.060 |
5.949 |
2.251 |
3.566 |
F (000) |
496 |
952 |
6272 |
1680 |
Crystal size (mm) |
0.40 × 0.16 × 0.10 |
0.22 × 0.21 × 0.11 |
0.45 × 0.41 × 0.40 |
0.20 × 0.10 × 0.05 |
Reflections collected |
4347 |
7139 |
75 079 |
5875 |
Data/restraints/parameters |
2960/0/154 |
2551/0/145 |
13 458/1379/642 |
5875/736/330 |
Goodness-of-fit on F2 |
1.152 |
1.020 |
1.132 |
0.870 |
Final R indices [I > 2σ (I)] |
R1 = 0.0826, wR2 = 0.2723 |
R1 = 0.0260, wR2 = 0.0627 |
R1 = 0.0910, wR2 = 0.1939 |
R1 = 0.0995, wR2 = 0.1428 |
R indices (all data) |
R1 = 0.0976, wR2 = 0.2780 |
R1 = 0.0343, wR2 = 0.0664 |
R1 = 0.2797, wR2 = 0.2243 |
R1 = 0.2796, wR2 = 0.1780 |
4.4. Antitumor assays
4.4.1 The antiproliferation of MDA cells assay for complex 4. MDA cells were provided by the American Type Culture Collection (ATCC) and were cultured in DMEM (Gibco) medium supplemented with 10% FBS and 100 U mL−1 penicillin–streptomycin at 37 °C and 5% CO2. Complex 4 was dissolved in DMSO then the solution was diluted with a medium in serial dilution. The dilutions of solutions were exposed to cells for 24 h. To investigate the effects of complex 4 on cell viability, the cells (3 × 105 cells per well) were exposed to various concentrations of complex 4 or treated with complex 4 at apoptotic concentrations in the presence or absence of NAC (15 mmol L−1) for 24 h. MTT solution (5 mg mL−1 in PBS) was added (50 μL per well) and the plates were further incubated for 4 h at 37 °C. Precipitated formazan crystals were dissolved by adding DMSO. Absorption was measured using a spectrometer (Bio-Rad iMark, CA, USA) at 490 nm.
4.4.2. Nuclei alteration assay for complex 4. Cells were seeded on a 96-well plate with or without complex treatment for 24 h. After the incubation period, the cells were loaded with DAPI by incubation (1 μmol L−1 for 5 min at 37 °C) in methanol solution, according to the Cell Staining kit manual (Biopec). The cells were then washed twice with HBSS solution and fluorescence was observed using a 350 nm excitation filter and 450 nm emission filter with an inverted microscope (NikonTi Japan); phase contrast images were captured by a CCD camera with commercial software.
4.4.3. Measurement of ROS assay for complex 4. For measurement of intracellular ROS levels by flow cytometry analysis, the oxidation-sensitive probe DCFH-DA was used. In brief, cells were detached by trypsinization, washed twice in warm DMEM and incubated in 10 μmol L−1 of H2DCF-DA at 37 °C for 30 min and then washed twice with PBS. Green fluorescence was detected by an inverted fluorescence microscope (Nikon Ti) according to protocol.
4.4.4. Mitochondrial membrane potential assay for complex 4. Cells (1 × 106) were washed with PBS and re-suspended in mitochondrial incubation buffer after being treated with complex 4 for 24 hours. JC-1 (molecular probe) was then added to the cells at a final concentration of 5 μmol L−1 and incubated at 37 °C in the dark for 30 minutes and mitochondrial membrane potential (the merge of red and green fluorescence) was detected by an inverted fluorescence microscope (Nikon Ti) according to protocol.
Acknowledgements
We are grateful to the National Natural Science Foundation of China (21371087) and the Natural Science Foundation of Shandong Province (ZR2015BL001) for financial support.
References
- V. Chandrasekhar, K. Gopal, P. Sasikumar and R. Thirumoorthi, Coord. Chem. Rev., 2005, 249, 1745–1765 CrossRef CAS.
- C. P. Sindlinger and L. Wesemann, Chem. Commun., 2015, 51, 11421–11424 RSC.
- Y.-B. Dong, H.-Y. Shi, J. Yang, Y.-Y. Liu and J.-F. Ma, Cryst. Growth Des., 2015, 15, 1546–1551 CAS.
- I. F. Hernandez-Ahuactzi, J. Cruz-Huerta, H. Tlahuext, V. Barba, J. Guerrero-Alvarez and H. Hopfl, Cryst. Growth Des., 2015, 15, 829–847 CAS.
- S. K. Hadjikakou and N. Hadjiliadis, Coord. Chem. Rev., 2009, 253, 235–249 CrossRef CAS.
- A. Gianguzza, O. Giuffre, D. Piazzese and S. Sammartano, Coord. Chem. Rev., 2012, 256, 222–239 CrossRef CAS.
- M. Hong, H. Geng, M. Niu, F. Wang, D. Li, J. Liu and H. Yin, Eur. J. Med. Chem., 2014, 86, 550–561 CrossRef CAS PubMed.
- I. Rojas-Leon, J. A. Guerrero-Alvarez, J. Hernandez-Paredes and H. Hopfl, Chem. Commun., 2012, 48, 401–403 RSC.
- B. Wrackmeyer, T. Chivers, D. J. Eisler, F. Ribot, V. Chandrasekhar, P. Singh, K. Gopal, H. Höpfl, S. C. Sevov, J. B. Lambert, A. V. Zabula, F. E. Hahn, Y. Mizuhata, N. Tokitoh, K. Jurkschat, M. Moya-Cabrera, V. Jancik, R. Cea-Olivares, M. Mazhar, D. Imtiazud, S. R. Whittleton, R. J. Boyd and T. B. Grindley, in Tin Chemistry, John Wiley & Sons, Ltd, 2008, pp. 17–283, DOI:10.1002/9780470758090.ch2.
- M. Nath, Appl. Organomet. Chem., 2008, 22, 598–612 CrossRef CAS.
- X. Wu, W. Kang, D. Zhu, C. Zhu and S. Liu, J. Organomet. Chem., 2009, 694, 2981–2986 CrossRef CAS.
- S. Tabassum, S. Mathur, F. Arjmand, K. Mishra and K. Banerjee, Metallomics, 2012, 4, 205–217 RSC.
- N. Gerasimchuk, T. Maher, P. Durham, K. V. Domasevitch, J. Wilking and A. Mokhir, Inorg. Chem., 2007, 46, 7268–7284 CrossRef CAS PubMed.
- T. Basu Baul, A. Paul, L. Pellerito, M. Scopelliti, P. Singh, P. Verma and D. Vos, Invest. New Drugs, 2010, 28, 587–599 CrossRef CAS PubMed.
- M. Vafaee, M. M. Amini, H. R. Khavasi, S. W. Ng and E. R. T. Tiekink, Appl. Organomet. Chem., 2012, 26, 471–477 CrossRef CAS.
- M. Sirajuddin, S. Ali, V. McKee, M. Sohail and H. Pasha, Eur. J. Med. Chem., 2014, 84, 343–363 CrossRef CAS PubMed.
- J. He, D. Li, K. Xiong, Y. Ge, H. Jin, G. Zhang, M. Hong, Y. Tian, J. Yin and H. Zeng, Bioorg. Med. Chem., 2012, 20, 3816–3827 CrossRef CAS PubMed.
- Z. Luo, B. Chen, S. He, Y. Shi, Y. Liu and C. Li, Bioorg. Med. Chem. Lett., 2012, 22, 3191–3193 CrossRef CAS PubMed.
- S.-C. Yu, H. Kuhn, C.-G. Daniliuc, I. Ivanov, P. G. Jones and W.-W. du Mont, Org. Biomol. Chem., 2010, 8, 828–834 CAS.
- S. M. Chopade, P. P. Phadnis, A. Wadawale, A. S. Hodage and V. K. Jain, Inorg. Chim. Acta, 2012, 385, 185–189 CrossRef CAS.
- K. I. Doudin, R. K. Berge, K. J. Børve, J. Songstad and K. W. Törnroos, J. Mol. Struct., 2000, 554, 149–161 CrossRef CAS.
- G. B. Deacon and R. J. Phillips, Coord. Chem. Rev., 1980, 33, 227–250 CrossRef CAS.
- K. Chandra, R. K. Sharma, B. S. Garg and R. P. Singh, J. Inorg. Nucl. Chem., 1980, 42, 187–193 CrossRef CAS.
- R.-H. Wang, M.-C. Hong, J.-H. Luo, R. Cao and J.-B. Weng, Eur. J. Inorg. Chem., 2002, 2002, 2082–2085 CrossRef.
- Q. Li, F. Wang, R. Zhang, J. Cui and C. Ma, Polyhedron, 2015, 85, 361–368 CrossRef CAS.
- Q.-F. Wang, C.-L. Ma, G.-F. He and Z. Li, Polyhedron, 2013, 49, 177–182 CrossRef CAS.
- T. Sedaghat, M. Aminian, H. A. Rudbari and G. Bruno, J. Organomet. Chem., 2014, 754, 26–31 CrossRef CAS.
- J. Holeček, M. Nádvorník, K. Handlíř and A. Lyčka, J. Organomet. Chem., 1986, 315, 299–308 CrossRef.
- A. Bondi, J. Phys. Chem., 1964, 68, 441–451 CrossRef CAS.
- M. D. Linnik, Restor. Neurol. Neurosci., 1996, 9, 219–225 CAS.
- V. Baille, P. G. H. Clarke, G. Brochier, F. Dorandeu, J. M. Verna, E. Four, G. Lallement and P. Carpentier, Toxicology, 2005, 215, 1–24 CrossRef CAS PubMed.
- N. Tuteja, M. B. Singh, M. K. Misra, P. L. Bhalla and R. Tuteja, Crit. Rev. Biochem. Mol. Biol., 2001, 36, 337–397 CrossRef CAS PubMed.
- M. Dizdaroglu, Cancer Lett., 2012, 327, 26–47 CrossRef CAS PubMed.
- F. Wolbers, P. Buijtenhuijs, C. Haanen and I. Vermes, Apoptosis, 2004, 9, 385–392 CrossRef CAS PubMed.
- J. Pi, J. Zeng, J. J. Luo, P. H. Yang and J. Y. Cai, Bioorg. Med. Chem. Lett., 2013, 23, 2902–2908 CrossRef CAS PubMed.
- K. Tsaggeos, N. Masiera, A. Niwicka, V. Dokorou, M. G. Siskos, S. Skoulika and A. Michaelides, Cryst. Growth Des., 2012, 12, 2187–2194 CAS.
- I. Afanaśev, Aging and Disease, 2011, 2, 219–230 Search PubMed.
- I. A. Mueller, J. M. Grim, J. M. Beers, E. L. Crockett and K. M. O'Brien, J. Exp. Biol., 2011, 214, 3732–3741 CrossRef CAS PubMed.
- L. Formentini, M. P. Pereira, L. Sanchez-Cenizo, F. Santacatterina, J. J. Lucas, C. Navarro, A. Martinez-Serrano and J. M. Cuezva, EMBO J., 2014, 33, 762–778 CrossRef CAS PubMed.
- Y. Ma, J. Zhang, Q. Zhang, P. Chen, J. Song, S. Yu, H. Liu, F. Liu, C. Song, D. Yang and J. Liu, Biochem. Biophys. Res. Commun., 2014, 448, 8–14 CrossRef CAS PubMed.
- K. Qu, N. Y. Shen, X. S. Xu, H. B. Su, J. C. Wei, M. H. Tai, F. D. Meng, L. Zhou, Y. L. Zhang and C. Liu, Acta Pharmacol. Sin., 2013, 34, 1217–1228 CrossRef CAS PubMed.
- Y. Guo, W. Zhang, Y. Y. Yan, C. G. Ma, X. Wang, C. Wang and J. L. Zhao, J. BUON, 2013, 18, 477–485 Search PubMed.
- G. M. Sheldrick, SHELXL-97, Program for Structure Solution, University of Göttingen, Germany, 1997 Search PubMed.
Footnote |
† Electronic supplementary information (ESI) available. CCDC 1444984 (for 1), 1444985 (for 2), 1444986 (for 3), 1444987 (for 4). For ESI and crystallographic data in CIF or other electronic format see DOI: 10.1039/c6ra01948j |
|
This journal is © The Royal Society of Chemistry 2016 |