DOI:
10.1039/C6RA01567K
(Paper)
RSC Adv., 2016,
6, 29378-29382
A facile strategy for the synthesis of monodispersed W17O47 nanoneedles†
Received
19th January 2016
, Accepted 14th March 2016
First published on 16th March 2016
Abstract
We present a facile one-pot wet chemical strategy for the synthesis of monodispersed W17O47 nanoneedles, of which the synthetic conditions and photothermal properties are systematically investigated. By modulating experimental conditions such as the ratio of solvent, the dose of sulfur powder and the reaction time, uniform W17O47 nanoneedles with an aspect ratio larger than 10 are obtained. These hydrophobic W17O47 nanoneedles are subsequently transferred into aqueous solution by coating with amphiphilic polyvinylpyrrolidone (PVP) for photothermal imaging applications. The results suggest that these monodispersed nanoneedles have potential for photothermal detection and photothermal therapy.
Introduction
Recently, nanostructured tungsten oxide has attracted extensive attention due to its unique physical/chemical properties such as high surface areas, light absorption, electrical conduction, thereby being explored for use in photocatalysis,1–3 electrochemistry,4 photoelectricity,5–7 gas sensing,8 and phototherapy.9 To date, a lot of effort has been devoted to preparing nanostructured tungsten oxide materials10–13 with various morphologies and phases such as nanosheets,14–16 nanowires,17–20 nanorods,21 nanoparticles,22 and hollow nanospheres23 using either physical or chemical routes. As a typical solution-phase synthesis method, Xi and co-workers17,24 synthesized W18O49 nanostrucutures by solvothermal treatment of anhydrous WCl6 in ethanol. This work presents not only a possibility for the use of ultrathin W18O49 nanowires as a functional material in the conversion of carbon dioxide but also a new strategy to design oxygen-vacancy-rich nonstoichiometric simple oxides with high photochemical activity. Wang and co-workers25 utilized vapor-phase deposition method to prepare three-dimensional (3D) WO3 nanostructures on carbon cloths with tungsten powder as the precursor by adjusting the growth temperature and the rate of O2 flow. The afforded 3D WO3 nanostructures displayed strong photocurrent responses under visible light irradiation, demonstrating its potential applicability for organic compounds decomposition and wastewater treatment. Kalantar-zadeh and co-workers26 employed another important method to prepare highly ordered 3D tungsten oxide with thickness up to 2 μm on a fluoride doped tin oxide (FTO) conductive glass by using an electrochemical anodization under mild chemical dissolution conditions at a low voltage of 10 V. These highly ordered 3D WO3 nanoporous networks have excellent coloration efficiencies that greatly exceeded the general range of the crystalline WO3 films.
On the other hand, nanostructures with near-infrared (NIR) light absorption as well as high photothermal performance27,28 are highly expected in view of photothermal applications including photothermal ablation,29–33 photothermal controlled drug delivery34 and photothermal imaging35 detection. Due to the unique defect structure and LSPR effect oxygen-deficient tungsten oxides (WO3−x) display strong absorption in the NIR region, which open the possibility of using them as photothermal agents. Such properties also promote great efforts in exploring novel synthetic strategies of tungsten oxides. Recent studies indicated that high photothermal conversion efficiency could be rationally improved by modulating the composition, morphology, surface functionalization, and assembly of the nanostructure.36–38 For example, WO3−x nanowires with strong NIR photo absorption have been proved to be a promising candidate as a photothermal agent due to their excellent biocompatibility and high photothermal effect.19 Hu and co-workers38 prepared WO3−x hierarchical nanostructures, which could serve as excellent laser-cavity mirrors, and be used to promote the photothermal conversion efficiency. Notwithstanding great success in the fabrication of WO3−x nanomaterials, it is still highly desirable and challenging to prepare the monodispersed WO3−x nanocrystals with a uniform shape and small size. Herein, we explored a facile strategy to prepare the monodispersed tungsten oxide (W17O47) nanoneedles with average size of ∼90 nm and broad NIR absorption (700–3300 nm). The uniform needle morphology could be obtained by tuning the solvent ratio, the dosage of sulfur powder, and the reaction time. After being encapsulated with polyvinylpyrrolidone (PVP), these W17O47 nanoneedles were further utilized for the photothermal imaging applications.
Experimental
Materials and methods
Oleylamine (80–90%, Acros Organics), 1-octadecene (90%, Sigma-Aldrich), oleic acid (90%, Alfa Aesar) and tungsten(VI) chloride (99%, Alfa Aesar) were used as obtained. Sulfur powder (CP), absolute ethyl alcohol (GR), cyclohexane (AR), tri-chloromethane (AR), n-hexane and polyvinylpyrrolidone (PVP, MW 10
000–70
000 g mol−1) were obtained from Beijing Chemical Reagent Company and were used as received without further purification.
Transmission electron microscopy (TEM) and high resolution TEM (HRTEM) images were recorded on a Hitachi H-800 transmission electron microscope operating at 100 kV and JEOL JEM-2100F transmission electron microscope operating at 200 kV, respectively. Bruker AXS D8-Advanced X-ray diffractometer with Cu Kα radiation (λ = 1.5418 Å) was employed to test powder X-ray diffraction (XRD) patterns with operation current and voltage at 40 mA and 40 kV (2θ ranging from 5° to 80°), respectively. The standard XRD pattern of W17O47 (JCPDS card no. 79-0171) was employed as a reference. UV-3600 UV-vis-NIR spectrophotometer (Shimadzu) equipped with a plotter unit was employed to measure absorption spectra. The photothermal performance was carried out with an FLIR camera.
Synthetic procedures
Synthesis of W17O47 nanoneedles. In a 100 mL three-neck flask, 2 mL of oleic acid and 8 mL of 1-octadecene were degassed for 15 min by nitrogen at room temperature. The solvent mixture was then heated up to 310 °C under nitrogen atmosphere. Meanwhile, 0.1 mmol of tungsten chloride, 0.2 mmol of sulfur powder and 2 mL of oleylamine were mixed in a centrifuge tube and subjected to sonication until full dissolution, which was then injected into the hot mixture solution quickly. Afterward, the temperature was kept at 310 °C for 1 h under nitrogen atmosphere. Then the mixture was left to cool down to room temperature naturally, followed by precipitation with ethanol. Finally, the powder nanomaterials were obtained by centrifuging and washing with ethanol and cyclohexane.
Preparation of hydrophilic W17O47@PVP nanoneedles. In a typical procedure,39 33 mg of hydrophobic W17O47 nanoneedles were dissolved in chloroform (20.0 mL) and then mixed with 125 mg of PVP (dissolved in 10.0 mL chloroform). The mixture was then sealed in beaker and stirred at room temperature for 24 h. The hydrophilic W17O47@PVP nanoneedles were collected by centrifugation (12
000 rpm, 10 min) with adding n-hexane, washed with chloroform and cyclohexane, and then redispersed in deionized water (0.1 mM) and stored for later use.
Results and discussion
As shown in the TEM images (Fig. 1a and b), the obtained needle-like nanostructure has a length around 90 nm and width about 9 nm with an aspect ratio of ∼10. The HRTEM image (Fig. 1c) showed a clear lattice fringes of 0.378 nm, consistent with the (010) lattice spacing of W17O47. It's notable that after coating with PVP, the afforded W17O47@PVP (Fig. 1d) was dispersed very well in water without obvious changes in shape and size. Moreover, X-ray diffraction (XRD) analysis was further employed to identify the phase composition and crystallographic structure of the samples. As shown in Fig. 2a, the XRD pattern of the as-obtained blue powder was well matched with the standard powder diffraction pattern of W17O47 as a reference (JCPDS card no. 79-0171). And the energy dispersive spectrum (EDS) results indicated that there was little sulfur on the surface of W17O47 (as Fig. S1†). The UV-vis-NIR absorption spectroscopy (Fig. 2b) indicated that the products have a broad absorption between 700 nm and 3300 nm, suggesting its potential applications for light absorption and photothermal conversion in a broad region, which is favorable for the convenient choice of various diode laser for photothermal tests.
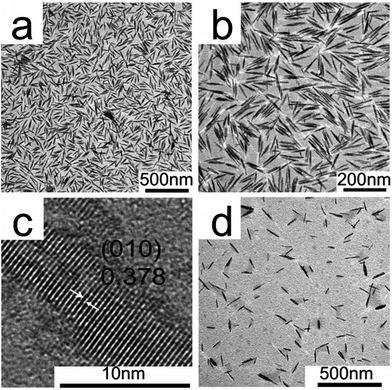 |
| Fig. 1 TEM and HRTEM images of W17O47 nanoneedles. (a) Low magnification; (b) high magnification; (c) HRTEM of hydrophobic W17O47 nanoneedles; (d) TEM image of W17O47@PVP dispersed in water. | |
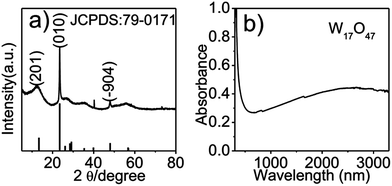 |
| Fig. 2 XRD pattern (a) and UV-vis-NIR absorption spectrum (b) of W17O47 nanoneedles. | |
To investigate the different factors for the growth of W17O47 nanoneedles, different experimental conditions such as solvent ratios, reaction time as well as material dosage were systematically studied. Initially, when fixed the total solvent volume (10 mL) while varying the volume ratios of oleic acid to 1-octadecene under identical conditions. It was found that the volume of oleic acid plays important roles on the morphology of nanomaterials. When the ratio was less than 2
:
8 (Fig. 3a and b), nanomaterials with various lengths were obtained (Table S1†). When 2 mL of oleic acid was injected into this system, nanomaterials with uniform size were acquired, as shown in Fig. 3c. However, keeping increasing the volume of oleic acid would lead to nanomaterial aggregation (Fig. 3d–g). If no 1-octadecene was used, entangled network of the nanomaterial could be obtained (Fig. 3h). To acquire the uniform size and morphology, we chose to use 2 mL of oleic acid in the following experiments.
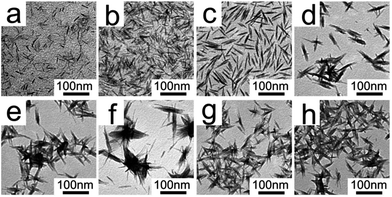 |
| Fig. 3 TEM images of W17O47 nanostructures prepared with different ratios of oleic acid to 1-octadecene. The ratios of oleic acid (mL) to 1-octadecene (mL) are (a) 0.5 : 9.5, (b) 1 : 9, (c) 2 : 8, (d) 3 : 7, (e) 5 : 5, (f) 7 : 3, (g) 8 : 2 and (h) 10 : 0. | |
Then the amount of sulfur powder was evaluated. In the absence of sulfur powder, nanowire was obtained (Fig. 4a). When 0.01 mmol sulfur powder was added into the reaction system, the sample was observed as fine nanoneedles (Fig. 4b). With the dosage of sulfur powder increasing, the morphologies of the samples stayed almost unchanged as seen in Fig. 4c–f, which showed the dosage of sulfur powder including 0.05 mmol, 0.10 mmol, 0.20 mmol and 0.30 mmol. The length, width and aspect ratio of W17O47 nanostructures with different dosage of sulfur power were shown in Table S2.† The aspect ratio was small with various length when sulfur powder under 0.20 mmol. It seems that the sulfur powder in this system has significant impact on the morphology of W17O47 by means of controlling the growth direction of W17O47 nanoneedles.
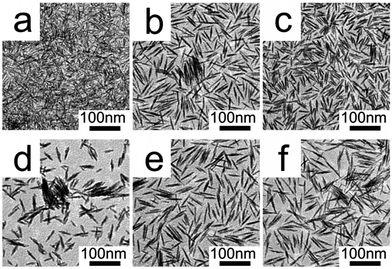 |
| Fig. 4 TEM images of W17O47 nanostructures prepared with different quantity of sulfur powder. The dosages of sulfur powder are (a) 0, (b) 0.01, (c) 0.05, (d) 0.10, (e) 0.20 and (f) 0.30 mmol. | |
As reaction time is an important factor affecting the crystallinity of W17O47, we also investigated the influence of reaction time on the nanostructures. The results revealed that W17O47 nanoneedles showed different morphology and aspect ratios under different reaction time, as shown in Fig. 5 and Table S3.† It appears that the W17O47 was formed by gradually etching bulk materials. When the reaction time was too short, still large portion of unreacted materials were left. As the reaction time increased to 1 h, the needle-like W17O47 with uniform size was observed, however, the long needles gradually fell apart as the reaction time was prolonged to 2 h, indicating that the reaction time is of crucial importance for achieving uniform W17O47 nanoneedles. As a result, 1 h was chosen as the reaction time for the synthesis of W17O47 nanoneedles.
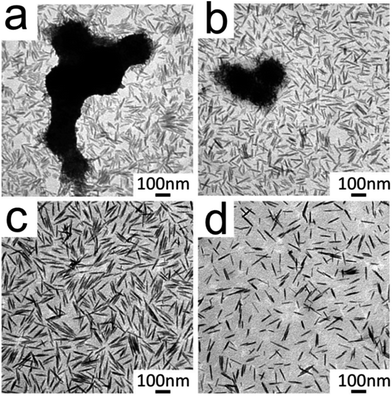 |
| Fig. 5 TEM images of W17O47 nanoneedles with different reaction time intervals. The reaction time is (a) 10 min, (b) 30 min, (c) 1 h and (d) 2 h, respectively. | |
As mentioned before, the W17O47 nanoneedles had a broad UV-vis-NIR absorption from 700 nm to 3300 nm, which is favorable for the photothermal tests via different diode laser. Prior to further investigation, the photothermal performance of W17O47 nanoneedles was carefully performed. The 808 nm irradiation light was frequently adopted in photothermal imaging and photothermal therapy because of its good dodging of absorption by water, blood and tissues. As a result, an excellent penetration and good photothermal efficacy is highly desirable via 808 nm irradiation. Under continuous irradiation (808 nm) with a power density of 1.0 W cm−2, the photothermal conversion profile shows the temperature changes of PVP coated W17O47 nanoneedles with different irradiation time (Fig. 6a). In addition, the temperature enhancement increased gradually along with the increment of W17O47 nanoneedle concentration and could reach 25 °C within 6 min when the concentration of W17O47 nanoneedles was 6.6 mg mL−1. As a control, the temperature change of pure water was increased only 0.7 °C under 808 nm light irradiation (1.0 W cm−2) within 6 min. This result suggests that the 808 nm light can efficiently dodge the absorption of water, which is highly preferable for in vivo/in vitro photothermal imaging and photothermal therapy because this weak absorption will cause low side effects to normal biosamples. In another aspect, the increase of power densities would enhance the temperature increments when with fixed amount of W17O47 nanoneedles (6.6 mg mL−1), as shown in Fig. 6b. We also tested the cytotoxicity of W17O47@PVP nanoneedles in Fig. S2,† indicating high biocompatability of the as-prepared W17O47@PVP nanoneedles and great potential to be used in biomedical applications such as photothermal imaging and photothermal therapy.
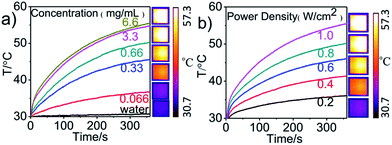 |
| Fig. 6 Photothermal conversion profiles. (a) Temperature evolution of W17O47@PVP colloids with different concentration (0.066–6.6 mg mL−1) versus irradiation time under irradiation of 808 nm NIR light (1.0 W cm−2). (b) Temperature evolution of W17O47 nanoneedle colloids (6.6 mg mL−1) versus irradiation time with different irradiation power densities under 808 nm NIR light. | |
Conclusions
In summary, we developed a facile one-pot wet chemical strategy for the synthesis of monodispersed W17O47 nanoneedles with uniform shape and size. After systematically investigating different experimental factors such as solvent ratios, sulfur powder dosage and reaction time, we found that sulfur powder and oleic acid played an important role in the controlled synthesis of W17O47 nanostructures with nanoneedle morphology. After being transferred into aqueous solution by coating with PVP, the W17O47@PVP was explored for the photothermal imaging, indicating its potential applications for photothermal detection and photothermal therapy.
Acknowledgements
This research was supported in part by the National Natural Science Foundation of China (Grant no. 21475007, 21275015 and 21505003). We also thank the support from the “Innovation and Promotion Project of Beijing University of Chemical Technology”, the “Public Hatching Platform for Recruited Talents of Beijing University of Chemical Technology, and the High-Level Faculty Program of Beijing University of Chemical Technology (buctrc201507), and BUCT Fund for Disciplines Construction and Development (Project No. XK1526)”.
Notes and references
- D. Chandra, K. Saito, T. Yui and M. Yagi, Angew. Chem., Int. Ed., 2013, 52, 12606–12609 CrossRef CAS PubMed.
- Q. X. Mi, Y. Ping, Y. Li, B. F. Cao, B. S. Brunschwig, P. G. Khalifah, G. A. Galli, H. B. Gray and N. S. Lewis, J. Am. Chem. Soc., 2012, 134, 18318–18324 CrossRef CAS PubMed.
- P. M. Rao, L. L. Cai, C. Liu, I. S. Cho, C. H. Lee, J. M. Weisse, P. D. Yang and X. L. Zheng, Nano Lett., 2014, 14, 1099–1105 CrossRef CAS PubMed.
- J. W. Liu, J. Zheng, J. L. Wang, J. Xu, H. H. Li and S. H. Yu, Nano Lett., 2013, 13, 3589–3593 CrossRef PubMed.
- J. Lee, C. Jo, B. Park, W. Hwang, H. I. Lee and S. Yoon, Nanoscale, 2014, 6, 10147–10155 RSC.
- W. T. Wu, J. J. Wu and J. S. Chen, ACS Appl. Mater. Interfaces, 2011, 3, 2616–2621 CAS.
- L. Liang, K. Li, C. Xiao, S. J. Fan, J. Liu, W. S. Zhang, W. H. Xu, W. Tong, J. Y. Liao, Y. Y. Zhou, B. J. Ye and Y. Xie, J. Am. Chem. Soc., 2015, 137, 3102–3108 CrossRef CAS PubMed.
- M. Horprathum, T. Srichaiyaperk, B. Samransuksamer, A. Wisitsoraat, P. Eiamchai, S. Limwichean, C. Chananonnawathorn, K. Aiempanakit, N. Nuntawong, V. Patthanasettakul, C. Oros, S. Porntheeraphat, P. Songsiriritthigul, H. Nakajima, A. Tuantranont and P. Chindaudom, ACS Appl. Mater. Interfaces, 2014, 6, 22051–22060 CAS.
- P. Kalluru, R. Vankayala, C. S. Chiang and K. C. Hwang, Angew. Chem., Int. Ed., 2013, 52, 12332–12336 CrossRef CAS PubMed.
- J. F. Al-Sharab, R. K. Sadangi, V. Shukla, S. D. Tse and B. H. Kear, Cryst. Growth Des., 2009, 9, 4680–4684 CAS.
- M. Breedon, P. Spizzirri, M. Taylor, J. du Plessis, D. McCulloch, J. M. Zhu, L. S. Yu, Z. Hu, C. Rix, W. Wlodarski and K. Kalantar-Zadeh, Cryst. Growth Des., 2010, 10, 430–439 CAS.
- C. Ng, C. H. Ye, Y. H. Ng and R. Amal, Cryst. Growth Des., 2010, 10, 3794–3801 CAS.
- Y. M. Solonin, O. Y. Khyzhun and E. A. Graivoronskaya, Cryst. Growth Des., 2001, 1, 473–477 CAS.
- W. J. Li, P. M. Da, Y. Y. Zhang, Y. C. Wang, X. Lin, X. G. Gong and G. F. Zheng, ACS Nano, 2014, 8, 11770–11777 CrossRef CAS PubMed.
- Y. P. He and Y. P. Zhao, J. Phys. Chem. C, 2008, 112, 61–68 CAS.
- J. Q. Yan, T. Wang, G. J. Wu, W. L. Dai, N. J. Guan, L. D. Li and J. L. Gong, Adv. Mater., 2015, 27, 1580–1586 CrossRef CAS PubMed.
- G. C. Xi, S. X. Ouyang, P. Li, J. H. Ye, Q. Ma, N. Su, H. Bai and C. Wang, Angew. Chem., Int. Ed., 2012, 51, 2395–2399 CrossRef CAS PubMed.
- J. C. Liu, O. Margeat, W. Dachraoui, X. J. Liu, M. Fahlman and J. Ackermann, Adv. Funct. Mater., 2014, 24, 6029–6037 CrossRef CAS.
- Z. G. Chen, Q. Wang, H. L. Wang, L. S. Zhang, G. S. Song, L. L. Song, J. Q. Hu, H. Z. Wang, J. S. Liu, M. F. Zhu and D. Y. Zhao, Adv. Mater., 2013, 25, 2095–2100 CrossRef CAS PubMed.
- S. W. Lin, Y. X. Guo, X. Li and Y. P. Liu, Mater. Lett., 2015, 152, 102–104 CrossRef CAS.
- K. Lee, W. S. Seo and J. T. Park, J. Am. Chem. Soc., 2003, 125, 3408–3409 CrossRef CAS PubMed.
- A. K. Baytak, S. Duzmen, T. Teker and M. Aslanoglu, Mater. Sci. Eng., C, 2015, 57, 164–170 CrossRef CAS PubMed.
- Z. F. Huang, J. J. Song, L. Pan, F. L. Lv, Q. F. Wang, J. J. Zou, X. W. Zhang and L. Wang, Chem. Commun., 2014, 50, 10959–10962 RSC.
- G. C. Xi, J. H. Ye, Q. Ma, N. Su, H. Bai and C. Wang, J. Am. Chem. Soc., 2012, 134, 6508–6511 CrossRef CAS PubMed.
- X. H. Zhang, X. H. Lu, Y. Q. Shen, J. B. Han, L. Y. Yuan, L. Gong, Z. Xu, X. D. Bai, M. Wei, Y. X. Tong, Y. H. Gao, J. Chen, J. Zhou and Z. L. Wang, Chem. Commun., 2011, 47, 5804–5806 RSC.
- J. Z. Ou, S. Balendhran, M. R. Field, D. G. McCulloch, A. S. Zoolfakar, R. A. Rani, S. Zhuiykov, A. P. O'Mullane and K. Kalantar-zadeh, Nanoscale, 2012, 4, 5980–5988 RSC.
- F. Hu, Y. Zhang, G. C. Chen, C. Y. Li and Q. B. Wang, Small, 2015, 11, 985–993 CrossRef CAS PubMed.
- X. G. Ding, C. H. Liow, M. X. Zhang, R. J. Huang, C. Y. Li, H. Shen, M. Y. Liu, Y. Zou, N. Gao, Z. J. Zhang, Y. G. Li, Q. B. Wang, S. Z. Li and J. Jiang, J. Am. Chem. Soc., 2014, 136, 15684–15693 CrossRef CAS PubMed.
- L. Cheng, K. Yang, Q. Chen and Z. Liu, ACS Nano, 2012, 6, 5605–5613 CrossRef CAS PubMed.
- Q. W. Tian, F. R. Jiang, R. J. Zou, Q. Liu, Z. G. Chen, M. F. Zhu, S. P. Yang, J. L. Wang, J. H. Wang and J. Q. Hu, ACS Nano, 2011, 5, 9761–9771 CrossRef CAS PubMed.
- X. J. Song, H. Gong, S. N. Yin, L. Cheng, C. Wang, Z. W. Li, Y. G. Li, X. Y. Wang, G. Liu and Z. Liu, Adv. Funct. Mater., 2014, 24, 1194–1201 CrossRef CAS.
- Z. J. Zhang, J. Wang, X. Nie, T. Wen, Y. L. Ji, X. C. Wu, Y. L. Zhao and C. Y. Chen, J. Am. Chem. Soc., 2014, 136, 7317–7326 CrossRef CAS PubMed.
- C. Liang, S. Diao, C. Wang, H. Gong, T. Liu, G. S. Hong, X. Z. Shi, H. J. Dai and Z. Liu, Adv. Mater., 2014, 26, 5646–5652 CrossRef CAS PubMed.
- S. Huang, J. Liu, Q. He, H. L. Chen, J. B. Cui, S. Y. Xu, Y. L. Zhao, C. Y. Chen and L. Y. Wang, Nano Res., 2015, 8, 4038–4047 CrossRef CAS.
- J. B. Cui, S. Y. Xu, C. Guo, R. Jiang, T. D. James and L. Y. Wang, Anal. Chem., 2015, 87, 11592–11598 CrossRef CAS PubMed.
- X. Wang, J. Zhuang, Q. Peng and Y. D. Li, Nature, 2005, 437, 121–124 CrossRef CAS PubMed.
- J. B. Cui, R. Jiang, S. Y. Xu, G. F. Hu and L. Y. Wang, Small, 2015, 11, 4183–4190 CrossRef CAS PubMed.
- B. Li, Y. X. Zhang, R. J. Zou, Q. Wang, B. J. Zhang, L. An, F. Yin, Y. Q. Hua and J. Q. Hu, Dalton Trans., 2014, 43, 6244–6250 RSC.
- G. Lu, S. Z. Li, Z. Guo, O. K. Farha, B. G. Hauser, X. Y. Qi, Y. Wang, X. Wang, S. Y. Han, X. G. Liu, J. S. DuChene, H. Zhang, Q. C. Zhang, X. D. Chen, J. Ma, S. C. J. Loo, W. D. Wei, Y. H. Yang, J. T. Hupp and F. W. Huo, Nat. Chem., 2012, 4, 310–316 CrossRef CAS PubMed.
Footnote |
† Electronic supplementary information (ESI) available. See DOI: 10.1039/c6ra01567k |
|
This journal is © The Royal Society of Chemistry 2016 |