DOI:
10.1039/C6RA01387B
(Paper)
RSC Adv., 2016,
6, 32454-32461
Impact of the functional group in the polyanion of single lithium-ion conducting polymer electrolytes on the stability of lithium metal electrodes†
Received
16th January 2016
, Accepted 13th March 2016
First published on 15th March 2016
Abstract
A novel single lithium-ion (Li-ion) conducting polymer electrolyte composed of lithium poly[(4-styrenesulfonyl)(fluorosulfonyl)imide] (LiPSFSI) and poly(ethylene oxide) (PEO) exhibits a high Li-ion transference number (tLi+ = 0.90) and sufficient electrochemical stability for use in Li batteries. The ionic conductivities of the LiPSFSI/PEO blended polymer electrolytes are higher than those of the lithium poly(4-styrenesulfonate) (LiPSS)/PEO electrolyte and are comparable to those of the lithium poly[(4-styrenesulfonyl)(trifluoromethanesulfonyl)imide] (LiPSTFSI)/PEO electrolyte in the temperature range of 25–90 °C. More importantly, the complex of LiPSFSI/PEO exhibits excellent interfacial compatibility with the Li metal electrode compared to both those of the LiPSS/PEO and LiPSTFSI/PEO electrolytes.
1. Introduction
Non-aqueous organic liquid electrolytes have been industrialized for applications in rechargeable lithium (Li) batteries (i.e., Li metal as anode) in 1990s.1 However, the high reactivity and flammability of the organic liquid electrolytes in the presence of Li metal causes safety problems, which finally hinders their practical applications in rechargeable Li batteries. Recently, all solid polymer electrolytes (SPEs) for rechargeable Li batteries continue to be a topic of increasing interest due to their potential advantages, including safety, good flame-resistance, excellent flexibility and low cost in design, and their functioning as a separator compared to liquid or gel electrolytes.2–8 Conventional SPEs are dual-ion conductors, which are prepared by dissolving common Li salt in a suitable polymer matrix (usually poly(ethylene oxide) (PEO)), such as LiClO4/PEO,9,10 LiBF4/PEO,11,12 LiSO3CF3/PEO,13 Li[N(SO2CF3)2] (LiTFSI)/PEO,14,15 Li[N(SO2CF2CF3)2] (LiBETI)/PEO,16,17 in which both cations and small anions are mobile similar to those in liquid electrolytes. Because Li batteries during cycling only involve the intercalation/deintercalation and plating/stripping of Li+ cations, practical cells using these conventional dual-ion conductive electrolytes result in concentration gradients of the salt and cell polarization, which ultimately cause premature cell failure.17,18
One of the best strategies to suppress the anionic mobility in conventional SPEs is to replace conventional dual-ion conductors with single Li-ion conductors (SLICs), in which the anions are immobilized by anion trapping agents or by fixing the anions to the polymeric backbones, so that the transference number (tLi+) of Li+ cations is close to one.19,20 Unfortunately, the low ionic conductivity and high interfacial resistance are the major barriers for rapidly developing SLICs for rechargeable Li batteries. This is largely due to the low negative charge distribution of anions (i.e., low Li-ion dissociation) and poor interfacial compatibility with electrodes (i.e., unstable and/or high interfacial resistance between electrodes and electrolyte membranes) in the SLICs. To break through the bottleneck of all-solid-state SLICs in conductivity, great efforts in earlier study have been made on gel-type SLICs,21–23 which are composed of all-solid-state SLICs and liquid solvents. While the ionic conductivities of SLICs is increased significantly upon the addition of low-viscosity organic solvent, the safety would still be an issue under abnormal use conditions due to the flammability of organic solvents.
As early as 1995, Armand et al.24 noted that the degree of negative charge distribution of anions plays a very important role in effectively improving the ionic conductivity of SPEs. They pointed out that the utilization of strong electron-withdrawing perfluorinated alkyl (Rf–) group to replace common hydrocarbon alkyl (R–) group in R–SO3− anion can increase the delocalized degree of anions, thus promoting the dissociation of Li+ cations. Subsequently, Watanabe et al.25 reported a new type of SLIC by dissolving lithium poly(2-oxo-1-difuluoroethylenesulfonylimide) ([Li(+) (–CO–CF2–SO2–N(−)–)n], LiPI) in polyether, in which tLi+ was found to be one. Unfortunately, the ionic conductivity was not increased as expected, due to the rigid structure of the polyanion (PI−). Recently, new SLICs comprised random and triblock copolymers, lithium poly[(4-styrenesulfonyl)(trifluoromethanesulfonyl)imide] (LiPSTFSI), and PEO, with improved ionic conductivities have been reported (e.g., σ = 10−4 at 70 °C for the Li[PSTFSI-co-MPEGA], 10−5 at 70 °C for the P(STFSILi)-b-PEO-b-P(STFSILi)).26,27 This would be due to the intrinsically flexible and delocalized nature and good plasticising effect of the –SO2–N(−)–SO2CF3 structure in the polyanion (PSTFSI−). More recently, Zhang et al. reported a sp3 boron-based solid SLIC for applications in Li/LiFePO4 half cells, which exhibits relatively high ionic conductivity and excellent cycling performance.28 Furthermore, we also reported a conventional SPEs based on the Li[N(SO2F)2] (LiFSI)/PEO (EO/Li+ = 20) for applications in Li/LiFePO4 half cells, which exhibit excellent cycling performance at 80 °C. This would be attributable to stable and low-resistance Li/electrolyte interface formed on the Li metal electrode in the LiFSI/PEO blended electrolyte, which is essentially originated from the reductions of FSI− anions,29 compared to those obtained in the classic complex of LiTFSI/PEO, though the mechanism still remains to be understood well. Encouraged by these results, we envisaged that it would be reasonable to achieve improved ionic conductivity and interfacial compatibility with Li metal electrode using the FSO2− group to replace the CF3SO2− group in the reported SLICs of LiPSTFSI.20,26
In the present study, we report a new type of single Li-ion conducting polymer electrolyte, which was prepared by blending lithium poly[(4-styrenesulfonyl)(fluorosulfonyl)imide] (LiPSFSI) (Scheme 1) with PEO. The ionic conductivity (σ), Li-ion transference number (tLi+), XRD characterization, and thermal behavior for the novel blended polymer electrolytes were investigated. Furthermore, we also demonstrated the impact of the functional group in the polyanion of SLICs on the stability of the Li metal electrode.
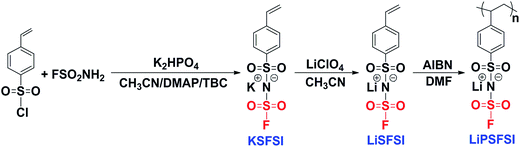 |
| Scheme 1 Synthetic routes of the monomers and polymer. TBC = 4-t-butylcatechol; DMAP = dimethylaminopyridine; AIBN = azodiisobutyronitrile. | |
2. Experimental section
2.1. Materials
Poly(ethylene oxide) (PEO, Mw = 4 × 106 g mol−1, Huagao Fine Chemicals, China) was dried at 50 °C for 10 h under vacuum conditions before use. Azodiisobutyronitrile (AIBN; Shanghai Reagent, China) was recrystallized twice from methanol before use. Lithium bis(fluorosulfonyl)imide (LiFSI, 99.5%, Suzhou Fluolyte Corp., Ltd., China) was used as received. 4-Vinylbenzenesulfonyl chloride and fluorosulfonamide were synthesized according to the reported procedures.30–32 All other reagents were purified using standard procedures before use. All the procedures related to handling moisture and oxygen sensitive reagents were performed under a dry argon atmosphere, or in a glove box (Mikrouna, H2O and O2 < 1 ppm).
2.2. Synthesis
2.2.1. Potassium [(4-styrenesulfonyl) (fluorosulfonyl)imide] (KSFSI). 4-Vinylbenzenesulfonyl chloride (44.59 g, 0.22 mol, abbreviated as PSCl), K2HPO4 (77.35 g, 0.44 mol), 4-t-butylcatechol (0.33 g, TBC, as inhibitor) and dimethylaminopyridine (0.74 g, DMAP, as catalyst) were mixed and dissolved in CH3CN (180 mL) at 0 °C under stirring conditions, then freshly distilled fluorosulfonamide (20.00 g, 0.20 mol) was added slowly under a nitrogen atmosphere. The mixture was stirred at room temperature for 72 h. The crude product was isolated by filtration and the filtrate was then evaporated under reduced pressure, washed successively with dichloromethane (3 × 200 mL) to remove impurities (e.g., unreacted raw materials, PSCl). The as-prepared product was then dried at 25 °C for 24 h under high vacuum, affording potassium [(4-styrenesulfonyl)(fluorosulfonyl)imide] (KSFSI) (24.41 g, yield 40%) as a white power. 1H NMR (399.65 MHz; CD3CN; TMS; ppm): δ = 7.83 (d, J = 8.4 Hz, 2H), 7.57 (d, J = 8.4 Hz, 2H), 6.83 (q, J = 17.7 Hz, 11.0 Hz, 1H), 5.94 (d, J = 17.7 Hz, 1H), 5.40 (d, J = 11.0 Hz, 1H). 19F NMR (376.05 MHz; CD3CN; CCl3F; ppm): δ = 54.40 (s, F). Elemental analysis calcd (%) for C8H7F1KN1O4S2: C, 31.67; H, 2.33; N, 4.62; found: C, 31.59; H, 2.51; N, 4.68. ESI-MS: ESI+ m/z 39 (100%, K+); ESI− m/z 264 (100%, [CH2 = CH–C6H4–SO2–N–SO2F]−).
2.2.2. Lithium [(4-styrenesulfonyl) (fluorosulfonyl)imide] (LiSFSI). LiSFSI was synthesized by a metathesis reaction between KSFSI and LiClO4 in CH3CN in almost quantitative yield, in a glove box (H2O and O2 < 1 ppm) specialized for preparing electrolyte salts, which is similar to the preparation of lithium (fluorosulfonyl)(pentafluoroethanesulfonyl)imide, as described in the literature.33 1H NMR (399.65 MHz; CD3CN; TMS; ppm): δ = 7.83 (d, J = 8.4 Hz, 2H), 7.58 (d, J = 8.4 Hz, 2H), 6.84 (q, J = 17.7 Hz, 11.0 Hz, 1H), 5.94 (d, J = 17.7 Hz, 1H), 5.41 (d, J = 11.0 Hz, 1H). 19F NMR (376.05 MHz; CD3CN; CCl3F; ppm): δ = 54.41 (s, F). 7Li NMR (156 MHz; CD3CN; ppm): δ = −4.03 (s, Li).
2.2.3. Lithium poly[(4-styrenesulfonyl)(fluorosulfonyl)imide] (LiPSFSI). LiPSFSI was synthesized via the free radical polymerization of LiSFSI (2.78 g, 0.01 mol) in DMF (12 mL) with AIBN (0.03 g, 0.18 mmol) as an initiator. The solution was heated at 60 °C for 12 h under argon atmosphere. The raw polymer was precipitated by pouring the solutions into a large excess of ether (200 mL). The gummy residue obtained was washed with excess ether three times (3 × 100 mL) and then dried at 55 °C for 12 h under high vacuum, affording lithium poly[(4-styrenesulfonyl)(fluorosulfonyl)imide] (LiPSFSI) (2.23 g, yield 80%) as a white powder. 1H NMR (399.65 MHz; CD3OD; TMS; ppm): δ = 7.72 (broad, 2H), 6.69 (broad, 2H), 1.54 (broad, 3H). 19F NMR (376.05 MHz; CD3OD; CCl3F; ppm): δ = 53.73 (broad, F).
2.3. Fabrication of polymer electrolyte membrane
The membrane of the blended polymer electrolyte was prepared by the solution casting method. First, the blended polymer electrolytes of lithium salt (LiPSFSI)/PEO were prepared by mixing and dissolving pre-weighed amounts of PEO and LiPSFSI in purified CH3OH in a glove box (H2O and O2 < 1 ppm). The resulting viscous solution was cast onto a Teflon plate with a doctor blade and left to evaporate the solvent slowly for 12 h at room temperature. The obtained self-standing, translucent film was dried under high vacuum at 60 °C for 48 h to remove any residual solvent. The film thickness was controlled accurately in the range of 100–200 μm.
2.4. Methods
The 1H (399.65 MHz), and 19F (376.05 MHz) NMR spectra were obtained on a Bruker AV400 spectrometer. The chemical shifts are reported in ppm relative to TMS for 1H and the external references CCl3F for 19F. Elemental analyses (C, H, and N) were performed on an Elementar Vario Micro Cube elemental analyzer.
The calorimetric measurements were performed on a differential scanning calorimeter (Netzsch 200 F3). A sample of an average weight of ca. 5–10 mg was hermetically sealed in an aluminum pan and stored at room temperature for two weeks prior to the measurement in a glove box (H2O and O2 < 1 ppm). The sample was measured using successive cooling-heating scans at a rate of 10 °C min−1 in the range of −100 to 100 °C under a nitrogen flow, except for the initial cooling scan from room temperature. The solid–solid transition temperature (Ts–s) was recorded at the onset of the heat capacity change, while the melting point (Tm) was recorded at the peak of the heat capacity change at the heating scan.
Thermogravimetric analysis (TGA) was performed on a NETZSCH STA 449C thermoanalyzer. Samples with an average weight of ca. 5–10 mg were placed in an aluminum pan and heated at a ratio of 10 °C min−1 from 30 to 600 °C under a flow of argon. The temperature at which 5% weight loss occurred was defined as the decomposition temperature (Td).
The number-average molecular weight (Mn) and polydispersity indices (PDI) of the prepared polymers were determined by gel permeation chromatography (GPC) using an Agilent 1100 GPC equipped with a PLgel 10 μm MIXED-B column and an RI detector. THF was used as the eluent with a flow rate of 1.0 mL min−1 at 25 °C. Polystyrene was used as an internal standard.
X-ray powder diffraction (XRD) was performed using a Bruker D8 X-ray diffractometer. The diffraction data were collected using Cu Kα radiation in the 2θ range from 5° to 90° at 40 mA and 40 kV.
The electrochemical measurements of the ionic conductivities (σ, in S cm−1) of the blended polymer electrolytes were carried out on an Autolab PGSTAT128N workstation (Eco Chemie, Netherland) using the AC impedance spectroscopic technique. The membranes of blended polymer electrolytes were sandwiched between a pair of stainless steel blocking electrodes. Before the measurement, the cell was first heated to 70 °C for 2 h to obtain good contact between the electrolytes and electrodes, and was then allowed to stand at room temperature for two weeks prior to the measurement. Subsequently, the measurements were performed in the temperature range from 25 to 90 °C for the heating scan. The cell was thermally equilibrated at the selected temperature at least for 1 h prior to the measurement. The AC impedance spectra were obtained over the frequency range from 0.01 to 106 Hz. The ionic conductivity (σ, in S cm−1) were derived from the measured resistance using the equation of σ = l/SR, where l (cm) is the thickness of the membrane of blended polymer electrolytes, A (cm2) is the area of the membrane, and R (Ω) is the resistance determined from the Nyquist plot.
The Li-ion transference number (tLi+) of the blended polymer electrolytes was measured at 70 °C with a symmetric cell of [Li metal|polymer electrolytes|Li metal] by a combination measurement of the AC impedance and DC polarization, which were described by Bruce and Abraham.34,35 The surface of lithium metal was shaved with a scalpel before use. The cell was assembled in a glove box (H2O and O2 < 1 ppm). Before measurement, the cell was heated to 70 °C for 2 h to obtain good contact, as well as for forming a stable interface, between the electrolytes and electrodes. A DC voltage of 10 mV was then applied to the cell until a steady current was obtained (usually 2–3 hours in this study) and the initial and steady currents, which flow through the cell, were measured. Simultaneously, the same cell was also monitored over the frequency range from 0.01 to 106 Hz, by AC impedance with an oscillation voltage of 5 mV to measure the resistance of the electrolytes and the interface of the electrolytes/Li metal electrodes, before and after DC polarization. The temperature of the cell was accurately controlled at 70 ± 0.1 °C using a thermostated container.
3. Results and discussion
3.1. Synthesis and characterization
The polymeric salt (LiPSFSI) was prepared according to Scheme 1, wherein the corresponding monomer of Li salt (LiSFSI) was first prepared and was polymerized sequentially into the target Li polymer salt (LiPSFSI) (the procedures are detailed in the aforementioned Experimental section). The structures and compositions of the monomer (LiSFSI) and its polymer (LiPSFSI) were confirmed by 1H and 19F NMR spectroscopy (Fig. S1†). The number-average molecular weight (Mn) and polydispersity index (PDI) of the as-prepared polymer (LiPSFSI) were characterized by gel permeation chromatography (GPC, Mn = 9.85 × 104 g mol−1, PDI = 1.40, Table 1), and its complex of LiPSFSI/PEO was thermally stable up to 200 °C (Fig. S2†). Similar sulfonate-based polymer salts (lithium poly(4-styrenesulfonate) (LiPSS) and LiPSTFSI, Fig. S3†) were also synthesized using the same methods as described previously for comparison,26,36 in the hope of better understanding the impact of the functional group in the polyanion of SLICs on the interfacial compatibility with Li metal electrode reliably.
Table 1 Characterization data for GPC of the prepared polymers
Samples |
Mna (×104) |
PDIb |
Appearance |
Molecular weight, which was determined by GPC calibrated with polystyrene standards. Polydispersity indices (Mw/Mn) of the prepared polymers, which was determined by GPC calibrated with polystyrene standards. |
LiPSFSI |
9.85 |
1.40 |
White power |
LiPSS |
7.50 |
— |
Yellow power |
LiPSTFSI26 |
5.28 |
1.27 |
Yellow power |
3.2. Phase behavior
The phase transition behaviors of all the prepared LiX/PEO (X = PSFSI, PSS, PSTFSI) blended polymer electrolytes with the same molar ratio of EO/Li+ = 8 and 20, respectively, as well as neat PEO, LiSFSI and LiPSFSI, were characterized by DSC. All the measurement data are summarized in Table 2.
Table 2 Characterization data for the phase transition behaviors of the blended polymer electrolytes of the LiX/PEO (X = PSFSI, PSS, PSTFSI) with the same molar ratio of EO/Li+ = 8 and 20, as well as for neat PEO, LiSFSI and LiPSFSI
Samples |
EO/Li+ |
Ts–sa/°C |
Tmb/°C |
ΔHmc/J g−1 |
Crystallinityd/% |
Solid–Solid transition temperature. Melting point. Enthalpy of melting. The crystallinity of the blended polymer electrolytes was calculated by: crystallinity = (ΔHm/ΔHo) × 100%, where ΔHm is the melting enthalpy of per unit mass of PEO and ΔHo is the value of 203 J g−1 reported in literature40 for the melting enthalpy of 100% crystalline PEO. |
LiSFSI |
|
17 |
|
5 |
|
LiPSFSI |
|
|
|
|
|
LiPSFSI/PEO |
8 |
|
62 |
23 |
11 |
LiPSFSI/PEO |
20 |
|
70 |
78 |
38 |
LiPSS/PEO |
8 |
|
71 |
70 |
34 |
LiPSS/PEO |
20 |
|
73 |
101 |
50 |
LiPSTFSI/PEO26 |
8 |
|
62 |
56 |
28 |
LiPSTFSI/PEO26 |
20 |
|
65 |
83 |
41 |
PEO |
|
|
70 |
122 |
60 |
Fig. 1 comparatively displays the DSC traces of the LiX/PEO (X = PSFSI, PSS, PSTFSI) blended polymer electrolytes with the same molar ratio of EO/Li+ = 8 and 20 at the heating scan. As observed from Fig. 1, all the DSC runs are characterized by an endotherm peak at about 60–70 °C for the LiX/PEO (X = PSFSI, PSS, PSTFSI) blended electrolytes. This peak is related to the classic melting of crystalline PEO. For the LiX/PEO (X = PSFSI, PSS, PSTFSI) blended electrolytes, the addition of Li salts results in a considerable decrease in crystallinity (χc) of PEO compared to neat PEO (Table 2). Moreover, the values of enthalpy of melting for the LiX/PEO (X = PSFSI, PSS, PSTFSI) blended electrolytes with a lower molar ratio of EO/Li+ = 8 (i.e., ΔHm = 23 J g−1, 70 J g−1, 56 J g−1, Table 2) were lower than those of the LiX/PEO (X = PSFSI, PSS, PSTFSI) electrolytes with a higher molar ratio of EO/Li+ = 20 (i.e., ΔHm = 78 J g−1, 101 J g−1, 83 J g−1, Table 2). This would be attributed to the plasticizing effects of Li salts on their blended electrolytes. Furthermore, for the same EO/Li+ molar ratio, the crystallinity (χc) of the LiX/PEO (X = PSFSI, PSTFSI) blended electrolytes is always lower than that of the LiPSS/PEO one (Table 2), indicating a higher degree of amorphous phase in the former two (LiPSFSI/PEO and LiPSTFSI/PEO). This would be attributed to more flexible and delocalized nature of the –SO2–N(−)–SO2− structure in PSFSI− and PSTFSI− anions, compared to the –SO3− structure in the PSS− anion, which enhances polymeric segmental motions responsible for promoting Li-ion motion through the electrolyte, besides increasing the degree of dissociation of the Li salts.
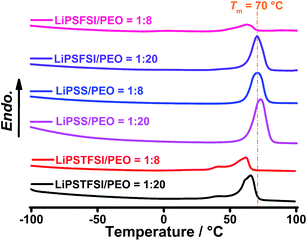 |
| Fig. 1 DSC traces of the LiX/PEO (X = PSFSI, PSS, PSTFSI) blended polymer electrolytes (EO/Li+ = 8 and 20) at the heating scan. | |
3.3. XRD characterization
Fig. 2 comparatively shows the XRD patterns of the LiX/PEO (X = PSFSI, PSS, PSTFSI) blended polymer electrolytes with the same molar ratio of EO/Li+ = 8 and 20, as well as the neat PEO for comparison. As shown in Fig. 2, two sharp diffraction peaks at 2θ = 19.2° and 23.3° for the neat PEO were observed, which is indicative of the presence of highly crystalline phase.16 However, the area and height of these two representative XRD peaks decrease rapidly upon the addition of the corresponding polymer salts (LiX, X = PSFSI, PSS, PSTFSI), indicating that blending can decrease the crystallinity (χc) of PEO. It appears that an increase in content of Li salts in the LiX/PEO (X = PSFSI, PSS, PSTFSI) blended electrolytes results in a decrease in crystallinity (χc) of PEO, as χc is lower for all the electrolytes with EO/Li+ = 8 than for those with EO/Li+ = 20. This trend in the XRD patterns is consistent with the DSC results in Fig. 1 (Table 2), wherein the enthalpy of melting is lower for the former than for the latter.
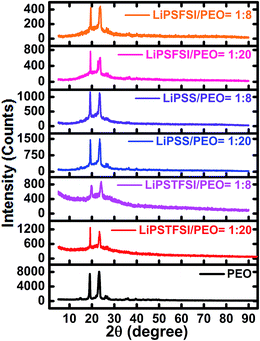 |
| Fig. 2 XRD patterns of the LiX/PEO (X = PSFSI, PSS, PSTFSI) blended polymer electrolytes (EO/Li+ = 8 and 20), as well as the neat PEO. | |
3.4. Lithium-ion transference number
Lithium-ion transference number (tLi+) plays a key role in improving the performance of SPEs, particularly the rate and interfacial performance. The values of tLi+ for the LiX/PEO (X = PSFSI, PSS, PSTFSI, and FSI) blended polymer electrolytes were measured by combining the AC impedance and DC polarization method (Fig. S4†) and are summarized in Table 3. The value of tLi+ for the classic ambipolar LiFSI/PEO (EO/Li+ = 20) blended electrolyte is 0.16 at 70 °C, which is comparable to that of 0.14 reported in the literature.29 In contrast, the value of tLi+ for the complex of LiPSFSI/PEO is as high as 0.90 at 70 °C, which is slightly higher than that of 0.85 for the LiPSS/PEO electrolyte,36 indicating that this blended polymer electrolyte based on the PSFSI− polyanion exhibits a single Li-ion conducting behavior. This is essentially due to the huge volume of the polyanion.
Table 3 Measured values for the parameters in equation and the corresponding calculated values of lithium-ion transference number (tLi+) at 70 °C
Electrolyte (EO/Li+ ratio by mole) |
Io (μA) |
Is (μA) |
Ri (Ω) |
Rf (Ω) |
Rol (Ω) |
Rsl (Ω) |
ΔV (mV) |
tLi+ |
LiPSFSI/PEO (8) |
0.548 |
0.398 |
446.6 |
469.0 |
14 013.4 |
20 151.0 |
10 |
0.90 |
LiPSFSI/PEO (20) |
1.140 |
0.523 |
630.8 |
581.1 |
9419.2 |
19 748.9 |
10 |
0.89 |
LiPSS/PEO (8)36 |
|
|
|
|
|
|
|
0.85 |
LiPSTFSI/PEO (8)26 |
|
|
|
|
|
|
|
0.92 |
LiFSI/PEO (20) |
195.6 |
635.4 |
15.89 |
15.81 |
31.05 |
33.38 |
10 |
0.16 |
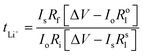 |
Where ΔV is the dc potential applied across the cell; Is and Io are the steady-state and the initial current determined by the dc polarization, respectively; Rol and Rsl are the interface resistance measured by the AC impedance method before and after polarization, respectively; Ri and Rf are the initial and final resistances of the electrolytes, respectively. |
3.5. Ionic conductivities
Fig. 3 compares the plots of ionic conductivities (σ) as a function of temperature for the LiX/PEO (X = PSFSI, PSS, PSTFSI, FSI, Fig. S5†) blended polymer electrolytes with a molar ratio of EO/Li+ = 8 and/or 20. As shown in Fig. 3, the ionic conductivities of the dual-ion conducting blended electrolyte, LiFSI/PEO (EO/Li+ = 20), measured in this study match those reported in the literature,29 indicating that these measurements are reliable. It can be noted that the ionic conductivities for these four types of blended electrolytes increase in the order of LiPSS/PEO (EO/Li+ = 20) < LiPSFSI/PEO (EO/Li+ = 20) = LiPSTFSI/PEO (EO/Li+ = 20) < LiPSFSI/PEO (EO/Li+ = 8) < LiFSI/PEO (EO/Li+ = 20) over the entire temperature range. The ionic conductivities of these blended electrolytes show typical behavior associated with polymer crystallinity and the concentration of charge carrier. The ionic conductivities of the SLICs, LiX/PEO (X = PSFSI, PSTFSI, EO/Li+ = 20) blended electrolytes, were about 1–2 orders in magnitude higher than those of the LiPSS/PEO (EO/Li+ = 20) electrolyte over the entire temperature range, which would be attributed to more flexible and delocalized nature of the –SO2–N(−)–SO2− structure in PSFSI− and PSTFSI− anions, compared to the –SO3− structure in PSS− anion. The ionic conductivities are about half order in magnitude higher for the LiPSFSI/PEO blended electrolyte with EO/Li+ = 8 than the corresponding one with EO/Li+ = 20 over the entire temperature range. This would be expected from the higher concentration of charge carriers (i.e., Li salt concentration) for the former with smaller ratio of EO/Li+ = 8 vs. the latter with EO/Li+ = 20.
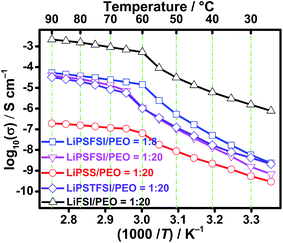 |
| Fig. 3 Temperature dependence of the ionic conductivities for the LiX/PEO (X = PSFSI, PSS, PSTFSI, FSI) blended polymer electrolytes (EO/Li+ = 8 and/or 20). | |
As shown in Fig. 3, the maximum ionic conductivities for the LiFSI/PEO blended electrolyte were observed over the entire temperature range, compared to these three types of SLICs (LiX/PEO, X = PSFSI, PSS, PSTFSI, Table S1†). This is expected from the dual-ion conducting nature of the complex of LiFSI/PEO, in which both cations and anions are mobile. By contrast, only cations are mobile and tLi+ approaches one in these three types of SLICs (LiX/PEO, X = PSFSI, PSS, PSTFSI, Table 3). All these blended electrolytes show an abrupt decrease in ionic conductivity at around 60–70 °C, which is related to the crystallization of PEO, as observed in the DSC traces in Fig. 1. In addition, it can be observed that the rate of increase in ionic conductivity becomes slower above 70 °C, suggesting that these blended electrolytes enter into the viscous flow state from their elastomeric state and the activation energies of their conductions decrease (Table S2†). Among these SLICs, the highest conductivities for the LiPSFSI/PEO (EO/Li+ = 8) blended electrolyte were obtained over the testing temperature range. The maximum in ionic conductivity would be a consequence of a trade-off between the chain flexibility and concentration of effective charge carrier. Some of representative values for the ionic conductivities of these blended electrolytes are summarized in Table S1† (e.g., σ = 2.32 × 10−9 at 25 °C, 1.43 × 10−5 at 60 °C, 5.30 × 10−5 at 90 °C for the LiPSFSI/PEO, EO/Li+ = 8). It is noticeable that the ionic conductivities below 70 °C for the LiX/PEO (X = PSFSI, PSS, PSTFSI, Table S1†) blended electrolytes are mainly due to the degree of dissociation of the Li salts, namely, Li salt concentration.
3.6. Electrochemical stability
The anodic electrochemical stability of the LiPSFSI/PEO (EO/Li+ = 20) blended polymer electrolyte was investigated by linear sweep voltammetry. Fig. 4 shows the polarization curves for the LiPSFSI/PEO (EO/Li+ = 20) blended electrolyte on [Li|SPEs|SS] coin type cells over a wide range of potentials at a sweep rate of 1 mV S−1 at 70 °C. As shown in Fig. 4, the LiPSFSI/PEO blended electrolyte displays an oxidation potential at ca. 4.5 V vs. Li+/Li, wherein the potential limit is set at a current density of 10 μA cm−2. This indicates that the anodic stability of LiPSFSI/PEO blended electrolyte would satisfy the requirements for application in 4 V class-Li batteries.
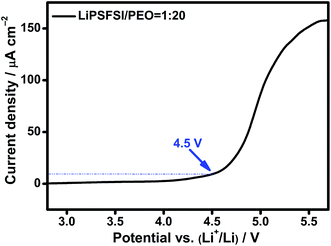 |
| Fig. 4 Linear sweep voltammogram of the complex of LiPSFSI/PEO (EO/Li+ = 20) on [Li|SPEs|SS] coin type cells, measured over a wide range of potentials from 2.7 to 6.0 V vs. Li+/Li. | |
3.7. Cycling performance
A symmetric [Li|SPEs|Li] cell was used for investigating the cycling performance of the Li electrode in the LiPSFSI/PEO (EO/Li+ = 20) blended polymer electrolyte at 80 °C at a low current density of 10 μA cm−2, as well as for the LiPSS/PEO (EO/Li+ = 20) and LiPSTFSI/PEO (EO/Li+ = 20) blended electrolytes, for comparison. Fig. 5 compares the cycling performance for the symmetric cells based on these three LiX/PEO (X = PSFSI, PSS, PSTFSI) blended electrolytes. As shown in Fig. 5a, an interesting note is that the cell polarization potential decreases rapidly in the first few cycles and then becomes relatively stable within less than 500 mV, even though small variations in potential are still observed, presumably due to surface roughening during the Li electrodeposition process.37 By contrast, in the LiPSS/PEO and LiPSTFSI/PEO blended electrolytes (Fig. 5b and c), the cell polarization potential changes randomly to lesser or greater extents during cycling and increases monotonically as a whole trend with continuous cycling until battery failure. This suggests that the dissolutions and regrowth of interphases occurred significantly and caused Li dendrite growth on Li metal electrode. This indicates that relatively stable interphases between these two blended electrolytes and Li metal electrodes cannot be formed. The abovementioned results suggest that the cycling stability of the symmetric cell based on the LiPSFSI/PEO blended electrolyte is superior to those based on both the LiPSS/PEO and LiPSTFSI/PEO electrolytes. This would be attributed to the better capability of FSO2− group in LiPSFSI in forming stable interphases with the Li metal electrode, compared to those of SO3− group in LiPSS and CF3SO2− group in LiPSTFSI. This result indicates that functional group in the polyanion of SLICs plays a key role in tailoring the properties of the interphases of Li metal electrode with SPEs, even though the clear mechanism still remains to be understood. A similar phenomenon was also observed in the FSI− anions-based SPEs and liquid electrolytes.29,38,39
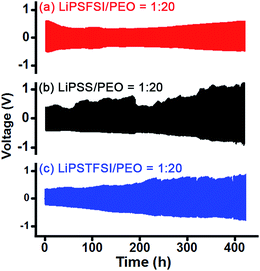 |
| Fig. 5 Cycling performance for the symmetric [Li|SPEs|Li] cells with LiX/PEO (X = PSFSI, PSS, PSTFSI) blended polymer electrolytes (EO/Li+ = 20) at a current density of 10 μA cm−2 at 80 °C. | |
4. Conclusions
A very convenient method for the preparation of lithium poly[(4-styrenesulfonyl)(fluorosulfonyl)imide] (LiPSFSI) was described. Novel single Li-ion conducting polymer electrolytes composed of LiPSFSI and high molecular weight poly(ethylene oxide) (PEO, Mw = 4 × 106 g mol−1) were prepared and characterized by DSC, TGA, XRD, ionic conductivity, Li-ion transference number (tLi+), and electrochemical stability, with a Li metal electrode. The complex of LiPSFSI/PEO indeed exhibited a high Li-ion transference number (tLi+ = 0.90) and an anodic electrochemical stability as high as 4.5 V vs. Li+/Li and its decomposition temperature was up to 200 °C. The ionic conductivities of the LiX/PEO (X = PSFSI, PSTFSI, EO/Li+ = 20) blended polymer electrolytes were about 1–2 orders of magnitude higher than those of the LiPSS/PEO (EO/Li+ = 20) electrolyte over the entire temperature range because of more flexible and delocalized nature of the –SO2–N(−)–SO2− structure in PSFSI− and PSTFSI− anions, compared to the –SO3− structure in PSS− anion. More importantly, the complex of LiPSFSI/PEO exhibited excellent interfacial compatibility with the Li metal electrode compared to both those of LiPSS/PEO and LiPSTFSI/PEO electrolytes. This would essentially be due to the FSO2− group in the polyanion of LiPSFSI. These preliminary results suggest that the LiPSFSI would be a promising salt of a polymer electrolyte, and would open a new and promising avenue for the future development of single Li-ion conductors in Li batteries.
Acknowledgements
This study was supported by the National Natural Science Foundation of China (No. 51172083, 51222210, 51472268).
Notes and references
- T. Tanaka, K. Ohta and N. Arai, J. Power Sources, 2001, 97–98, 2–6 CrossRef CAS
. - J.-M. Tarascon and M. Armand, Nature, 2001, 414, 359–367 CrossRef CAS PubMed
. - D. T. Hallinan and N. P. Balsara, Annu. Rev. Mater. Res., 2013, 43, 503–525 CrossRef CAS
. - D. Zhou, Y.-B. He, R. L. Liu, M. Liu, H. D. Du, B. H. Li, Q. Cai, Q.-H. Yang and F. Y. Kang, Adv. Energy Mater., 2015, 5, 1500353 Search PubMed
. - Y. B. Liu, Z. J. Cai, L. Tan and L. Li, Energy Environ. Sci., 2012, 5, 9007–9013 CAS
. - Y. Lin, J. Li, Y. Q. Lai, C. F. Yuan, Y. Cheng and J. Liu, RSC Adv., 2013, 3, 10722–10730 RSC
. - Q. Ma, H. Zhang, C. W. Zhou, L. P. Zheng, P. F. Cheng, J. Nie, W. F. Feng, Y.-S. Hu, H. Li, X. J. Huang, L. Q. Chen, M. Armand and Z. B. Zhou, Angew. Chem., Int. Ed., 2016, 55, 2521–2525 CrossRef CAS PubMed
. - Y. Wang and W.-H. Zhong, ChemElectroChem, 2015, 2, 22–36 CrossRef CAS
. - W. A. Henderson and S. Passerini, Electrochem. Commun., 2003, 5, 575–578 CrossRef CAS
. - S. Das and A. Ghosh, Electrochim. Acta, 2015, 171, 59–65 CrossRef CAS
. - E. M. Fahmi, A. Ahmad, N. N. M. Nazeri, H. Hamzah, H. Razali and M. Y. A. Rahman, Int. J. Electrochem. Sci., 2012, 7, 5798–5804 CAS
. - M. Kalita, M. Bukat, M. Ciosek, M. Siekierski, S. H. Chung, T. Rodrĺguez, S. G. Greenbaum, R. Kovarsky, D. Golodnitsky, E. Peled, D. Zane, B. Scrosati and W. Wieczorek, Electrochim. Acta, 2005, 50, 3942–3948 CrossRef CAS
. - L. R. A. K. Bandara, M. A. K. L. Dissanayake and B.-E. Mellander, Electrochim. Acta, 1998, 43, 1447–1451 CrossRef CAS
. - W. Gorecki, M. Jeannin, E. Belorizky, C. Roux and M. Armand, J. Phys.: Condens. Matter, 1995, 7, 6823–6832 CrossRef CAS
. - Y. Zhao, R. Y. Tao and T. Fujinami, Electrochim. Acta, 2006, 51, 6451–6455 CrossRef CAS
. - G. B. Appetecchi, W. Henderson, P. Villano, M. Berrettoni and S. Passerini, J. Electrochem. Soc., 2001, 148, A1171–A1178 CrossRef CAS
. - J.-H. Shin and S. Passerini, Electrochim. Acta, 2004, 49, 1605–1612 CrossRef CAS
. - J. B. Kerr, S. E. Sloop, G. Liu, Y. B. Han, J. Hou and S. Wang, J. Power Sources, 2002, 110, 389–400 CrossRef CAS
. - J. Rolland, E. Poggi, A. Vlad and J.-F. Gohy, Polymer, 2015, 68, 344–352 CrossRef CAS
. - R. Meziane, J.-P. Bonnet, M. Courty, K. Djellab and M. Armand, Electrochim. Acta, 2011, 57, 14–19 CrossRef CAS
. - X. J. Wang, Z. H. Liu, Q. S. Kong, W. Jiang, J. H. Yao, C.
J. Zhang and G. L. Cui, Solid State Ionics, 2014, 262, 747–753 CrossRef CAS
. - Y. F. Zhang, R. Rohan, Y. B. Sun, W. W. Cai, G. D. Xu, A. Lin and H. S. Cheng, RSC Adv., 2014, 4, 21163–21170 RSC
. - Y. S. Zhu, S. Y. Xiao, Y. Shi, Y. Q. Yang and Y. P. Wu, J. Mater. Chem. A, 2013, 1, 7790–7797 CAS
. - D. Benrabah, S. Sylla, F. Alloin, J.-Y. Sanchez and M. Armand, Electrochim. Acta, 1995, 40, 2259–2264 CrossRef CAS
. - M. Watanabe, Y. Suzuki and A. Nishimoto, Electrochim. Acta, 2000, 45, 1187–1192 CrossRef CAS
. - S. W. Feng, D. Y. Shi, F. Liu, L. P. Zheng, J. Nie, W. F. Feng, X. J. Huang, M. Armand and Z. B. Zhou, Electrochim. Acta, 2013, 93, 254–263 CrossRef CAS
. - R. Bouchet, S. Maria, R. Meziane, A. Aboulaich, L. Lienafa, J. P. Bonnet, T. N. T. Phan, D. Bertin, D. Gigmes, D. Devaux, R. Denoye and M. Armand, Nat. Mater., 2013, 12, 452–457 CrossRef CAS PubMed
. - Y. F. Zhang, W. W. Cai, R. Rupesh, M. Z. Pan, Y. Liu, X. P. Liu, C. C. Li, Y. B. Sun and H. S. Cheng, J. Power Sources, 2016, 306, 152–161 CrossRef CAS
. - H. Zhang, C. Y. Liu, L. P. Zheng, F. Xu, W. F. Feng, H. Li, X. J. Huang, M. Armand, J. Nie and Z. B. Zhou, Electrochim. Acta, 2014, 133, 529–538 CrossRef CAS
. - H. Kamogawa, A. Kanzawa, M. Kadoya, T. Naito and M. Nanasawa, Bull. Agric. Chem. Soc. Jpn., 1983, 56, 762–765 CrossRef CAS
. - J. Cortés, M. M. Mañas and R. Pleixats, Eur. J. Org. Chem., 2000, 239–243 CrossRef
. - V. P. Doz, R. Appel and D. C. W. Senkpiel, Eingegangen, 1958, Z 660.
- H.-B. Han, Y.-X. Zhou, K. Liu, J. Nie, X.-J. Huang, M. Armand and Z.-B. Zhou, Chem. Lett., 2010, 39, 472–474 CrossRef CAS
. - J. Evans, C. A. Vincent and P. G. Bruce, Polymer, 1987, 28, 2324–2328 CrossRef CAS
. - K. M. Abraham, Z. Jiang and B. Carroll, Chem. Mater., 1997, 9, 1978–1988 CrossRef CAS
. - C. H. Park, Y.-K. Sun and D.-W. Kim, Electrochim. Acta, 2004, 50, 375–378 CrossRef CAS
. - X.-G. Sun, J. Hou and J. B. Kerr, Electrochim. Acta, 2005, 50, 1139–1147 CrossRef CAS
. - R. R. Miao, J. Yang, X. J. Feng, H. Jia, J. L. Wang and Y. Nuli, J. Power Sources, 2014, 271, 291–297 CrossRef CAS
. - Q. Ma, Z. Fang, P. Liu, J. Ma, X. G. Qi, W. F. Feng, J. Nie, Y.-S. Hu, H. Li, X. J. Huang, L. Q. Chen and Z. B. Zhou, ChemElectroChem, 2016 DOI:10.1002/celc.201500520
. - B. Wunderlich, Macromolecular Physics, Academic Press, New York, 1980, pp. 66–67 Search PubMed
.
Footnote |
† Electronic supplementary information (ESI) available. See DOI: 10.1039/c6ra01387b |
|
This journal is © The Royal Society of Chemistry 2016 |