DOI:
10.1039/C6RA01140C
(Paper)
RSC Adv., 2016,
6, 34574-34587
Pd cocatalyst on Sm-doped BiFeO3 nanoparticles: synergetic effect of a Pd cocatalyst and samarium doping on photocatalysis†
Received
14th January 2016
, Accepted 30th March 2016
First published on 31st March 2016
Abstract
To explore the synergetic effect of rare earth doping and noble metal cocatalyst loading on the photocatalytic activity of BiFeO3 (BFO), in this work, novel Pd cocatalyst-loaded and Sm-doped BFO (Pd/BSFO) composite photocatalysts containing different Pd loading contents were successfully prepared by using a sol–gel method followed by an impregnation process. The experimental results revealed that the rhombohedral structure of BFO was distorted by Sm substitution, and Pd cocatalyst nanoparticles were uniformly deposited on the surface of BSFO particles with the formation of a heterojunction at the interface between Pd and BSFO. Compared to the BFO, BSFO and Pd/BFO sample, the Pd/BSFO samples exhibited improved UV-vis spectral absorption ability and significantly enhanced photocatalytic activities for the photodegradation of methyl orange or a colorless compound (i.e., phenol) under visible light irradiation. When the Pd loading amount on the surface of BSFO was 1.5 wt%, the optimal photocatalytic degradation efficiency was achieved. The enhanced photocatalytic activity of the Pd/BSFO photocatalyst could be attributed to the increased optical absorption, the efficient charge transfer and separation as well as the suppressed recombination of photogenerated electron–hole pairs derived from the Sm dopant trapping level and the heterojunction formation of the Schottky barrier between BSFO and the Pd cocatalyst in Pd/BSFO, as verified by photoluminescence (PL) emission spectra, photocurrent action spectra and electrochemical impedance spectra (EIS). On the basis of the calculated energy bands and trapping experiments, the photocatalytic mechanism was also discussed.
1. Introduction
In the past few decades, there has been great interest in developing semiconductor photocatalysts with high catalytic efficiency and good stability for water pollutant treatment in environmental remediation1 and photocatalytic hydrogen production from water splitting.2,3 Bismuth ferrite (BiFeO3, BFO), as a well-known multiferroic material which possesses ferroelectric and antiferromagnetic orders at room temperature, has widely been used in data storage, spintronics devices, memory, sensors and so on.4,5 Recently, BFO has been considered as a kind of promising visible-light responsive photocatalyst for photocatalytic applications,6–8 because of its narrow band gap (∼2.2 eV), chemical stability, and low cost. In spite of its great potential, however, the reported photocatalytic activity of BFO is still not impressive owing to the poor carrier mobility and the rapid recombination of photogenerated electron–hole pairs in BFO, which will be a longstanding challenge that would greatly constrain its widespread use in the field of photocatalysis.9 Therefore, strategies are needed to be made for improvement of the photocatalytic activity of BFO.
In order to improve the photocatalytic activity of BFO photocatalyst, much effort has been devoted to controlling its structural parameters (such as morphology, size, and dimensions7,10) and varying its compositional parameters (such as composites,11,12 core–shell structuring13). For example, Li et al.7 demonstrated that compared with BFO microspheres and microcubes, the BFO submicrocubes showed better photocatalytic activity for degradation of Congo Red under visible-light illumination. Lou et al.13 reported that SrTiO3-coated BFO core/shell nanostructures could decompose water to produce H2 under visible-light irradiation. On the other hand, doping of BFO with a foreign atom at either A or B or A and B sites of the ABO3 lattice has been proven as an effective approach to improve the photocatalytic properties of BFO,14 because the dopants within the photocatalyst may act as electron or hole trapping sites which could facilitate the production and separation of photogenerated electron–hole pairs during the photocatalytic reaction.15 For example, the substitution of Bi3+ with rare-earth elements (such as Dy3+, or Gd3+) could result in remarkable improvement of the photocatalytic activity of BFO.16,17 Additionally, the loading of suitable cocatalyst nanoparticles (such as Au, Pt) has been demonstrated as another effective method for enhancing photocatalytic activity of BFO.18,19 It is supposed that the enhanced photocatalytic activities of the cocatalysts-loaded BFO could be ascribed to the enhanced separation efficiency of photogenerated electron–hole pairs due to the presence of heterojunction structure at the interface between cocatalyst and BFO. For example, Li et al.18 reported that 1.0 wt% Au nanoparticle decorated BFO nanowires exhibited ∼30 times higher photocatalytic activity for water oxidation than pure BFO nanowires. Nevertheless, it should be noted that these previous reports have studied only one effect on the photocatalysis of BFO, either loading noble metals or doping with rare-earth metals, which cannot improve the photocatalytic activity in the maximal degree. In fact, the synergetic effect of cocatalyst loading and metal doping on photocatalysis has been already observed in other photocatalysts.20–22 For instance, Yu et al. prepared a novel TiO2-based photocatalytic degradation system assisted by conventional Pd cocatalysis and Ni-doping, which exhibited remarkably improved efficient photodegradation of 4-XP under visible-light irradiation compared to those assisted by the single Pd co-catalysis or Ni-doping.22
To the best of our knowledge, however, the synergetic effect of loading noble-metal nanoparticles and doping with rare-earth metals on photocatalytic activity of BFO has not previously been investigated, and thus has received less attention. Herein, to further improve the photocatalytic activity of BFO, the synergetic effect of cocatalyst loading and rare earth doping on the photocatalysis of BFO was explored for the first time. Accordingly, BFO nanoparticles were firstly doped with Sm cations and then loaded with Pd nanoparticles, and thus a novel BFO-based composite photocatalyst (Pd/BSFO) was obtained. The as-prepared Pd/BSFO composite photocatalyst demonstrated superior photocatalytic activities for photodegradation of methyl orange or colorless compounds (i.e., phenol) compared to the pure BFO, Pd-loaded BFO (Pd/BFO) and Sm-doped BFO (BSFO), arising from the synergetic effect of Sm doping and Pd cocatalyst loading. On the basis of experimental results, the enhanced photocatalytic mechanism of Pd/BSFO nanocomposite was also proposed.
2. Experimental
2.1 Photocatalyst preparation
All chemicals were of analytical grade without further purification. Pure BFO and BSFO were prepared by using a conventional sol–gel method. In a typical procedure, appropriate amounts of Bi(NO3)3·5H2O (0.097 M), Sm(NO3)3·6H2O (0.003 M), and Fe(NO3)3·9H2O (0.1 M) with a stoichiometric ratio were dissolved in 100 ml of ethylene glycol to form a precursor solution. Tartaric acid (molar ratio of tartaric acid to nitrate was 1
:
1) was added to the above precursor solution, and the mixture was stirring until a brownish-red-colored homogeneous solution was obtained. Subsequently, the homogeneous solution was kept at 80 °C for 1.5 h to form a sol. The sol was then dried at 100 °C for 48 h to get a gel. The resultant gel was calcined at 550 °C for 2 h to obtain the pure BFO or BSFO powder.
An impregnation-deposition method was used to prepare Pd/BSFO composite photocatalysts. Typically, BSFO powder (3 g) was impregnated in an aqueous solution containing a desired amount of PdCl2 for 2 h, followed by the addition of NaBH4 solution (0.01 M). The resulting precipitate were washed with distilled water several times, and then dried in a vacuum oven at 70 °C followed by a heat treatment in a tube furnace at 300 °C for 1.5 h under a flowing mixed gas of N2/H2 (5 vol% H2). The loaded weight percentages of Pd on BSFO powder were 1.0 wt%, 1.5 wt% and 2.0 wt% (relative to the weight of BSFO), which were denoted as “1.0 wt% Pd/BSFO”, “1.5 wt% Pd/BSFO” and “2.0 wt% Pd/BSFO”, respectively. For comparison, BFO loaded with a weight percentage of 1.5 wt% Pd and BSFO loaded with a weight percentage of 1.5 wt% Ni were also prepared according to the same procedure as mentioned above except for the use of BFO or Ni(NO3)2·6H2O in replace of BSFO or PdCl2, respectively. The obtained powders were denoted as “1.5 wt% Pd/BFO” and “1.5 wt% Ni/BSFO”, respectively.
2.2 Photocatalyst characterizations
The crystal structures of the as-obtained photocatalysts were recorded on a Bruker D2 X-ray diffractometer (XRD) using Cu Kα radiation. The morphologies and composition of the samples were taken using a scanning electron microscope (SEM, HitachiSU8010) attached with an energy dispersive X-ray spectrometer (EDX) and an emission transmission electron microscope (TEM) (JEOL JEM-2100). The chemical states presented in Pd/BSFO were analyzed using a PHI 5000 Versa Probe X-ray photoelectron spectrometer with Al Kα radiation. The UV-visible diffuse reflectance spectra (UV-vis DRS) of the samples were carried out by an UV-visible spectrophotometer (Shimadzu UV-3600) using BaSO4 as reference. The solid state photoluminescence (PL) spectra were recorded on a Hitachi High-Tech F-7000 fluorescence spectrophotometer with an xenon lamp as an excitation source (λ = 404 nm).
2.3 Photocatalytic measurements
The photocatalytic activities of as-prepared photocatalysts were monitored through photocatalytic degradation of methyl orange (MO) or phenol in an aqueous solution under visible light irradiation (λ ≥ 420 nm) by using a 300 W Xe lamp assembled with a cut-off filter as a light source. During the photocatalytic experiment, 0.3 g of the as-prepared photocatalyst powder was added into 100 ml of MO or phenol solution (5 mg L−1), and then magnetically stirred in the dark for 1 h to ensure adsorption/desorption equilibrium. At a given time interval, 4 ml of the suspension was collected and centrifuged at 9000 rpm for 30 min to remove the catalyst powders. The concentration of MO (or phenol) was then detected by measuring the maximum absorbance at 465 nm (or 270 nm) using a spectrophotometer (model: 722, Precision Instrument Co., Ltd. Shanghai, China). To test the stability of Pd/BSFO composite, the remaining photocatalyst particles in suspension were collected and washed to remove the residual MO and dried before another catalytic reaction. This process was recycled for five times.
2.4 Photoelectrochemical measurements
To prepare the working electrodes for the photoelectrochemical characterization, the F-doped SnO2 (FTO) conductive glass was ultrasonically cleaned in soap-suds, deionized water and acetone successively. The working electrodes were prepared by mixing a slurry containing 90% obtained sample and 10% polymer binder (polyvinylidene difluoride) on FTO and then dried in a vacuum oven at 60 °C for 24 h. The active area of electrodes was controlled to be 1.0 × 2.0 cm2, and the visible light source was a 300 W Xe lamp assembled with a cut-off filter (λ ≥ 420 nm). The photoelectrochemical measurements were recorded on a CHI660E electrochemical station using the standard three-electrode system with a saturated calomel electrode (SCE) as the reference electrode, and platinum wire as counter electrode. The photocurrent measurements were performed in 0.5 M Na2SO4 (at pH = 6.8) aqueous solution at 0.3 V potential bias vs. SCE under visible light (λ ≥ 420 nm) irradiation. Mott–Schottky analysis was carried out at an applied frequency of 1000 Hz in the dark. Electrochemical impedance spectra (EIS) were recorded in the same three-electrode configuration by applying an AC voltage with 5 mV amplitude in the frequency range from 0.01 Hz to 100 kHz under visible light (λ ≥ 420 nm) irradiation.
3. Results and discussion
3.1 Materials characterization
The crystal structures of the as-obtained photocatalysts were characterized by XRD measurements. As seen in Fig. 1(a), pure BFO showed a crystalline phase with a rhombohedral structure with the space group R3c (JCPDS no. 86-1518), indicating that pure BFO phase could be obtained by the present sol–gel process. Meanwhile, the Sm-doped BFO sample (BSFO) presented a XRD pattern similar to that of pure BFO, demonstrating good dispersion and incorporation of Sm dopant in the BFO host. From the magnified XRD patterns in the vicinity of 2θ around 32 degrees (Fig. 1(b)), however, it can be seen that the separated (104) and (110) diffraction peaks for BSFO had an obvious tendency to shift toward higher 2θ values compared to pure BSO, which was attributed to the substitution of Bi3+ (1.03 Å) with smaller sized Sm3+ (0.958 Å) ions in BFO.23 This result suggests that the rhombohedral structure was distorted by Sm substitution, which has also been observed in other rare earth doped BFO materials.24 It is also noted that the diffraction patterns for the Pd/BSFO and Pd/BFO samples were similar to that of BSFO or pure BFO, and no Pd crystalline phase was detected due to the relatively low Pd loading concentration.25 This indicates that the loading of Pd cocatalyst would not change the BFO crystal structure.
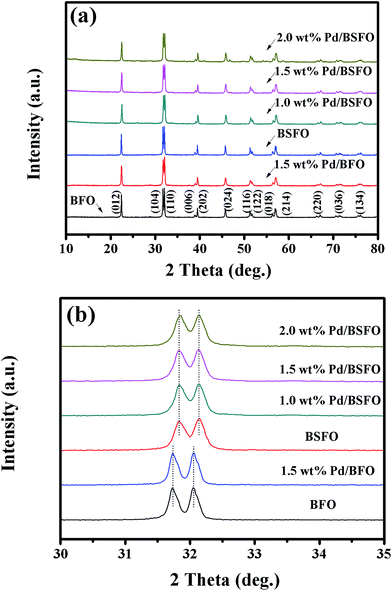 |
| Fig. 1 (a) XRD patterns of the prepared BFO, Pd/BFO, BSFO and Pd/BSFO samples. (b) The magnification of the diffraction peak from 30−35°. | |
The morphological features of as-prepared Pd/BSFO photocatalysts were characterized by SEM and TEM analysis. Fig. 2(a) shows the typical SEM image of the obtained 1.5 wt% Pd/BSFO sample. As shown, the sample was composed of irregular nanoparticles with a grain size of 100–150 nm. To reveal the distribution of Sm and Pd elements in the Pd/BSFO sample, the elemental mapping for the 1.5 wt% Pd/BSFO sample was measured (Fig. 2(b)–(d)). It can be seen that the dispersion state of green dots representing Sm element were well-proportioned, indicating the homogeneous doping of Sm in the BFO lattice, as presented in Fig. 2(c). Also, the blue dots representing Pd element were homogeneously dispersed in the map, confirming a well dispersion of Pd particles on the surface of BSFO (Fig. 2(d)). In order to analyze the chemical compositions of the Pd/BSFO samples, the EDS spectrum of the 1.5% Pd/BSFO sample was obtained, as shown in Fig. 2(e). The emergence of Bi, Fe, O, Sm and Pd characteristic peaks confirms that the prepared sample was composed of Bi, Fe, O, Sm and Pd elements. Fig. 3 shows the TEM and HRTEM images of the 1.5 wt% Pd/BSFO sample. As shown, the 1.5 wt% Pd/BSFO particles were irregular and prone to aggregate, and the average particle size was ca. 120 nm, which was in accordance with the SEM results. Meanwhile, it can be seen that Pd nanoparticles (marked by a dot circle) were dispersed on the surface of BSFO particles, and the average Pd particle size was estimated to be approximately 5 nm. The crystal lattices of Pd and BSFO particles can be clearly observed in Fig. 3(b). The measured crystal interplanar spacings for BSFO and Pd particles were 0.396 nm and 0.225 nm, which could be assigned to the (012) crystal plane of the BSFO crystal and the (111) crystal plane of Pd,26 respectively. Moreover, the HRTEM image also confirms the formation of an intimate interface between Pd and BSFO, thus leading to the formation of heterojunction structure.
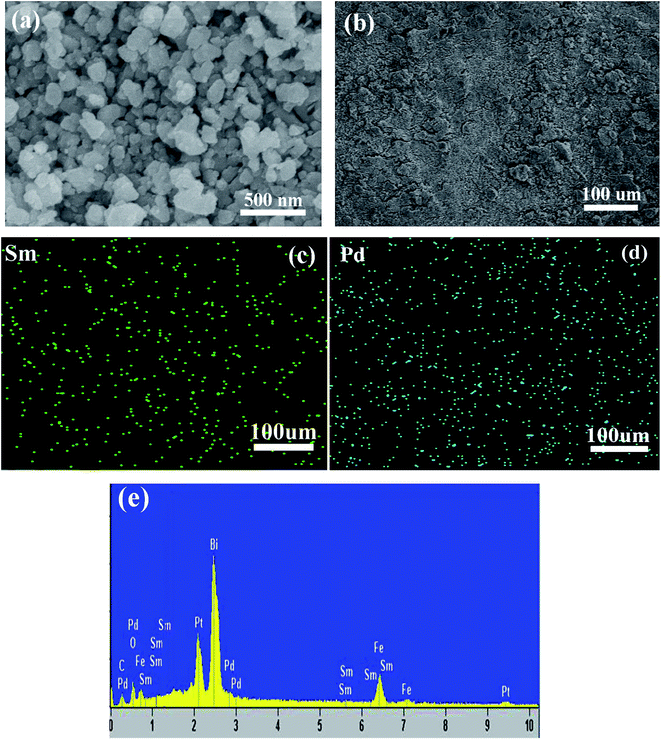 |
| Fig. 2 (a) SEM micrograph of the 1.5 wt% Pd/BSFO sample; (b) SEM micrograph of the 1.5 wt% Pd/BSFO corresponding EDX distribution maps of Sm element (c) and EDX distribution maps of Pd element (d); (e) EDS pattern of the 1.5 wt% Pd/BSFO. (Note: the labeled elements of carbon (C) and platinum (Pt) in the EDS pattern were detected from the conductive tape and sprayed metal during the specimen preparation for SEM measurement, respectively.) | |
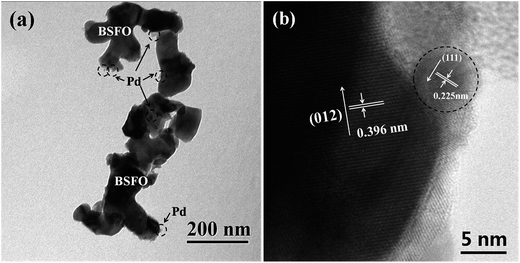 |
| Fig. 3 (a) TEM and (b) HRTEM micrograph of the 1.5 wt% Pd/BSFO sample. | |
X-ray photoelectron spectroscopy was employed to determine the chemical states of the Bi, Fe, O, Sm and Pd elements in the 1.5 wt% Pd/BSFO sample. Fig. 4 presents the XPS core level spectra of Bi 4f, Fe 2p, O 1s, Sm 3d, and Pd 3d in the 1.5 wt% Pd/BSFO sample. As shown in Fig. 4(a), the binding energies of 158.3 and 163.9 eV could be assigned to the Bi 4f7/2 and Bi 4f5/2 peaks, implying that bismuth was in the form of Bi3+.27 The XPS spectrum of the Fe 2p orbital (Fig. 4(b)) reveals that the doublet peaks located at 709.8 and 723.6 eV were Fe 2p3/2 and Fe 2p1/2, respectively, arising from spin-orbital interaction. The observation of the satellite peak at 717.8 eV (≈8 eV above the Fe 2p3/2 peak) indicates that the Fe element was in the Fe3+ valence state in the Pd/BSFO sample.28 The O 1s XPS spectrum (Fig. 4(c)) shows that the two different peaks centered at 528.9 and 531.0 could be ascribed to lattice oxygen and chemisorbed oxygen, respectively.29 The XPS spectrum for Sm 3d shown in Fig. 4(d) reveals that the Sm 3d states were split into Sm 3d5/2 and Sm 3d3/2 arising from spin–orbit interaction, and the doublet peaks for Sm 3d5/2 and Sm 3d3/2 were centered at around 1083.5 and 1110.8 eV,30 indicating the existence of trivalent oxidation state of Sm in the Pd/BSFO sample. As depicted in Fig. 4(e), the two characteristic Pd 3d peaks appearing at 335.7 eV and 341.0 eV could be assigned to Pd 3d5/2 and Pd 3d3/2 respectively, which confirms the presence of metallic Pd in the sample.31 From the above XPS results, it can be concluded that Bi, Sm and Fe existed in a form of Bi3+, Sm3+ and Fe3+ in the obtained Pd/BSFO particles, respectively, and that the loading Pd cocatalyst was in a metal state though no crystalline phase was detected by XRD.
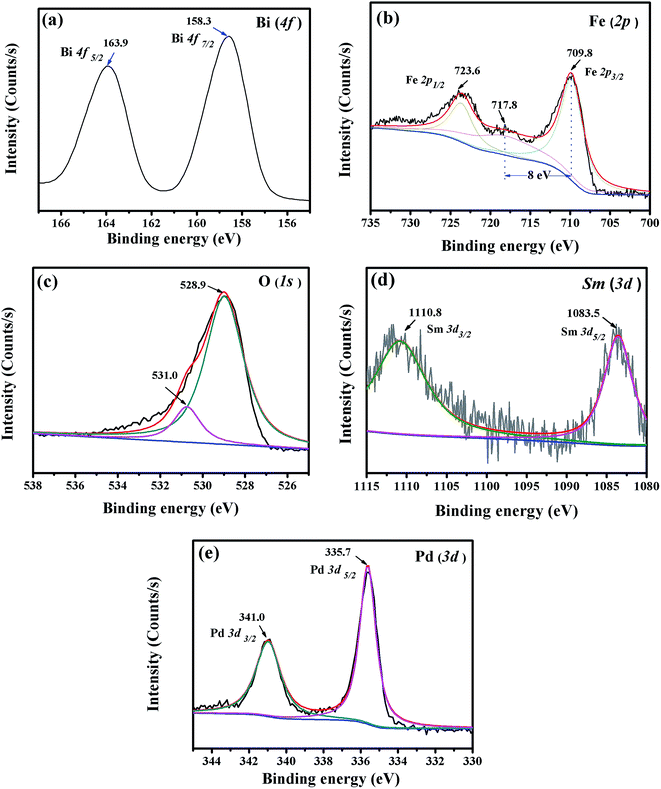 |
| Fig. 4 XPS spectra of (a) Bi, (b) Fe, (c) O, (d) Sm and (e) Pd elements for the 1.5 wt% Pd/BSFO sample. | |
Prior to the photocatalytic measurements, the optical properties of the as-prepared photocatalyst samples were examined. Fig. 5(a) shows the UV-vis diffuse reflectance spectra of BFO, Pd/BFO, BSFO and Pd/BSFO samples. The absorption edge of the as-prepared BFO sample was about 563 nm, and all the other samples exhibited a little red-shifted absorption edge. This indicates that all the as-prepared photocatalysts could respond to visible light for photocatalytic reaction. On the basis of the Tauc plot equation (αhν = A(hν − Eg)n/2), the energy bandgaps of the obtained photocatalysts could be calculated.32 As shown in Fig. 5(b), the band gap values for BFO, 1.5 wt% Pd/BFO, BSFO, 1.0 wt% Pd/BSFO, 1.5 wt% Pd/BSFO and 2.0 wt% Pd/BSFO were estimated to be 2.20, 2.18, 2.14, 2.12, 2.08 and 2.00 eV, respectively. Compared to BFO, the BSFO sample exhibited a little stronger visible light absorption accompanying with a slightly lower band gap. The doping of Sm in BFO may cause an increase in density of states in the valance band,33 thus leading to a narrowed band gap as well as a shift in the absorption edge towards lower photon energy. Besides, the Pd/BFO and Pd/BSFO samples showed enhanced visible-light absorption compared to the BFO or BSFO photocatalyst sample, suggesting that the loading of Pd cocatalyst could utilize more visible light for photocatalyic reaction. What is more, the band gap values of the Pd/BSFO samples were gradually decreased with the increase of the Pd loading content. The influence of Pd cocatalyst on the band gap of BFO or BSFO could be explained by the following two reasons. On one hand, the deposited Pd cocatalyst nanoparticles could modify the original energy equilibrium, and produce some lower energy levels arising from the heterojunction of Schottky barrier formed at the interface between Pd and semiconductor, thus leading to the facile charge–transfer transition between noble metals and semiconductors.19,34 On the other hand, the deposited Pd nanoparticles could absorb visible light owing to their surface plasmon resonance (SPR) effect. With the increase of the loading content of Pd cocatalyst, a slight red-shift in the SPR would occur due to the increased electromagnetic retardation of Pd/BFO and Pd/BSFO particles.35 Thus, the enhanced optical property of Pd/BSFO could be attributed to the synergetic effect of Sm doping and Pd cocatalyst loading, which would probably improve the photocatalytic activity of BFO.
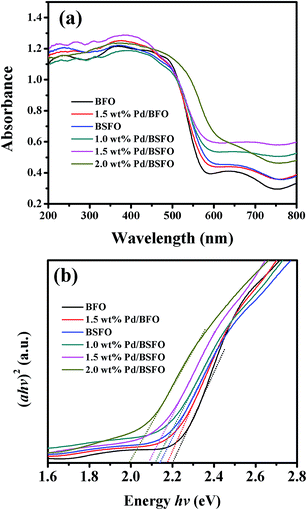 |
| Fig. 5 (a) UV-vis diffuse reflectance spectra of the prepared BFO, Pd/BFO, BSFO and Pd/BSFO samples; (b) plots of (αhν)2 vs. hν derived from the UV-visible spectra for the prepared samples. | |
3.2 Photocatalytic measurements
The photocatalytic performances of the as-prepared photocatalyst samples were evaluated by photocatalytic degradation of MO aqueous solution as displayed in Fig. 6. Fig. 6(a) shows the photodegradation efficiencies of MO as a function of illumination time with different photocatalysts under visible light (λ ≥ 420 nm) irradiation. A blank experiment without any catalyst was taken under the same condition. It is found that the self degradation of MO pollutant was inappreciable. When the as-prepared photocatalyst sample was added, the degradation of MO took place under visible light irradiation. After 120 min irradiation time, 36%, 42%, 47%, 60%, 80%, 87% and 76% of MO were decomposed for the BFO, Pd/BFO, BSFO, 1.0 wt% Pd/BSFO, 1.5 wt% Pd/BSFO and 2.0 wt% Pd/BSFO samples, respectively. Among them, the 1.5 wt% Pd/BSFO sample yielded the highest MO degradation efficiency, which was about 2.4 times that of the pure BFO. It can be seen that a trend in the photocatalytic activity was in the following order: 1.5 wt% Pd/BSFO > 1.0 wt% Pd/BSFO > 2.0 wt% Pd/BSFO > BSFO > 1.5 wt% Pd/BFO > BFO. Compared to the BFO sample, the photodegradation efficiency of the BSFO or 1.5 wt% Pd/BFO sample was slightly enhanced, while a noticeable increase of the MO photodegradation efficiency was observed for all the Pd/BFSO samples. This confirms that either Sm doping or Pd cocatalyst loading could not improve the photocatalytic activity of BFO in the maximal degree, and the optimum photocatalytic activity of BFO could be obtained by combining Sm doping and Pd cocatalyst loading. To quantitatively analyze the reaction kinetics of MO degradation, the pseudo-first-order model according to Langmuir–Hinshelwood model36 was applied, as expressed by the following equation (eqn (1)): |
 | (1) |
where C0 and C are the concentration of MO (mg L−1) at different irradiation time of t0 and t, respectively, and k is the pseudo-first-order rate constant of photodegradation (min−1). From Fig. 6(b), the linear fitting curves of ln(C0/C) versus irradiation time (t) shows a good correlation to pseudo-first-order reaction kinetics for as-prepared photocatalysts. The corresponding k values (Fig. 6(c)) were calculated to be 353 × 10−5, 448 × 10−5, 735 × 10−5, 1302 × 10−5, 1682 × 10−5, and 1025 × 10−5 min−1 for the BFO, 1.5 wt% Pd/BFO, BSFO, 1.0 wt% Pd/BSFO, 1.5 wt% Pd/BSFO and 2.0 wt% Pd/BSFO sample, respectively. The k values of the Pd/BFO and BSFO sample were about 1.3 times and 2.1 times that of pure BFO sample, respectively. Nevertheless, the 1.5 wt% Pd/BSFO exhibited the highest k value, which was 4.8 times higher than that of pure BFO sample.
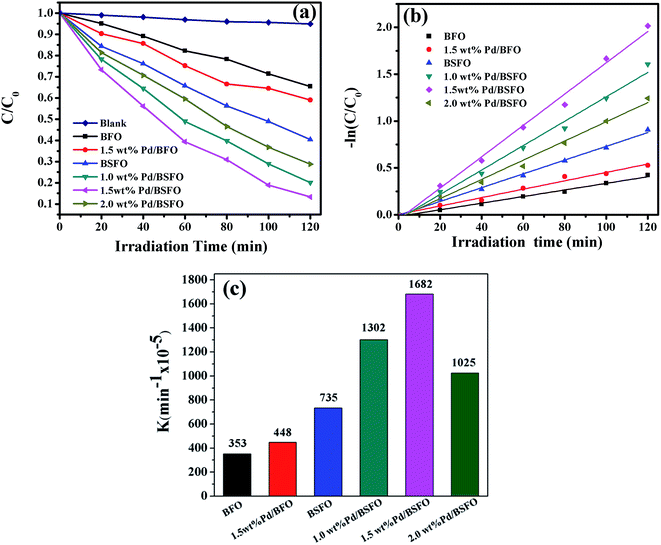 |
| Fig. 6 (a) Photocatalytic degradation of MO as a function of the irradiation time under visible light (λ ≥ 420 nm) irradiation for obtained samples; (b) pseudo-first order kinetics fitting data for the photodegradation of MO under visible light (λ ≥ 420 nm) irradiation; (c) the kinetic constants of MO degradation on various photocatalysts. | |
As known, the stability and reusability of a photocatalyst is crucial to its practical applications. Fig. 7(a) shows the MO photodegradation results of the 1.5 wt% Pd/BSFO photocatalyst for five cycles under visible light irradiation. As shown, the photocatalytic activity was largely maintained after five successive runs, indicating a good stability. In addition, the XRD patterns of 1.5 wt% Pd/BSFO sample before and after photocatalytic degradation reaction (Fig. 7(b)) reveal that no crystal structure change occurred during the photocatalytic reaction, further indicating a good stability of the as-prepared Pd/BSFO photocatalyst.
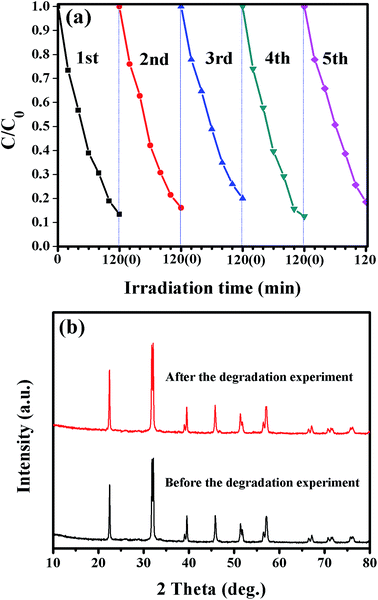 |
| Fig. 7 (a) Photocatalytic degradation of MO with the 1.5 wt% Pd/BSFO sample for five cycles under visible light (λ ≥ 420 nm) irradiation; (b) XRD patterns of the 1.5 wt% Pd/BSFO before and after the photocatalytic degradation experiment. | |
As pointed out clearly by Ohtani,37 a colored organic dye is not suitable for evaluating the visible light photocatalytic activity due to its dye-sensitization effect, which complicates the photocatalytic mechanism of dye degradation. To eliminate the dye-sensitization effect on photocatalysis, the photocatalytic activities of as-prepared photocatalyst samples were also evaluated by degradation of a colorless compound (i.e., phenol) under visible light (λ ≥ 420 nm) irradiation. The photocatalytic results are shown in Fig. S1.† For the blank sample without addition of any photocatalyst, the concentration of phenol was nearly unchanged during the whole visible light irradiation time, implying that phenol is a stable pollutant. When the as-prepared photocatalyst was added, phenol consequently was decomposed under visible light irradiation. The phenol photodegradation efficiencies of as-prepared photocatalysts were increased successively in the order of BFO, 1.5 wt% Pd/BFO, BSFO, 2.0 wt% Pd/BSFO, 1.0 wt% Pd/BSFO and 1.5 wt% Pd/BSFO, and the 1.5 wt% Pd/BSFO sample exhibited the highest photocatalytic activity for phenol degradation. Apparently, the trend in the photocatalytic activities of as-prepared photocatalysts for phenol degradation was the same as that for MO degradation. This means that the “dye-sensitized” photoinduced oxidative decomposition had a little effect on the photocatalytic activities of as-prepared photocatalyts.
In addition, as Pd is a noble metal, it is not economical to use Pd cocatalyst for photocatalytic degradation of organic pollutants. It is commonly accepted that a non-noble metal cocatalyst would be more desirable. To date, some transition metals (such as Ni) have been employed as cocatalysts for enhancing photocatalytic activity of the photocatalyst.38,39 For comparison, we also prepared 1.5 wt% Ni/BSFO photocatalysts, and evaluated their photocatalytic activities by photodegradation of phenol under visible light (λ ≥ 420 nm) irradiation. The successful preparation of 1.5 wt% Ni/BSFO photocatalysts was verified by various characterization techniques including XRD, XPS and SEM, as presented in Fig. S2 and S3.† The photocatalytic results in Fig. S4† show that the phenol photodegradation efficiencies for the BFO, BSFO, 1.5 wt% Ni/BSFO and 1.5 wt% Pd/BSFO samples were 9.8%, 21.7%, 28.8% and 50.1%, respectively. As seen, the photodegradation efficiency of BSFO was enhanced when Pd or Ni cocatalyst was loaded on the surface of BSFO, and the 1.5 wt% Pd/BSFO exhibited the highest photodegradation efficiency, which was 1.7 times that of 1.5 wt% Ni/BSFO. Thus, the loading of noble metal Pd cocatalyst would enhance the photocatalytic activity of BSFO more efficiently than the loading of transition metal Ni cocatalyst.
3.3 Photocatalytic mechanism
It is known that dyes and organic pollutants can be photodegraded through photocatalytic oxidation by some reactive species, such as h+, ˙OH and O2˙−.12 To clarify the reactive species involved in the photocatalytic degradation of phenol by the obtained Pd/BSFO catalyst, the trapping experiments were performed. The phenol photodegradation results of the 1.5 wt% Pd/BSFO photocatalyst with different types of active species scavengers under visible light irradiation (λ ≥ 420 nm) are presented in Fig. 8. As seen, the phenol degradation efficiency was accelerated with the addition of AgNO3 (an electron scavenger,40 2 mM) in comparison to that without addition of quencher. The enhancement of photodegradation rate might arise from the promotion of separation of electron–hole pairs caused by the consumption of photogenerated electrons. When KI (a hole-scavenger,41 2 mM) was added to the phenol solution, the phenol degradation rate was reduced to 23.4%, indicating that the consumption of excited holes could play an important role in the photodegradation rate. When tert-butyl alcohol (TBA, a hydroxyl radicals scavenger,42 2 mM) was added, the phenol degradation rate was dramatically decreased, implying that hydroxyl radicals (˙OH) could significantly influence the photocatalytic activity of Pd/BSFO. In addition, the addition of benzoquinone (BQ, 0.5 mM) scavenger for superoxide radicals O2˙− (ref. 43) resulted in a slight decrease in phenol photodegradation activity, indicating that superoxide radical (O2˙−) did not dominate the photodegradation process. The above trapping experiments clearly show that the predominant active species for phenol photodegradation by the Pd/BSFO photocatalyst were hydroxyl radicals (˙OH) and photogenerated holes (h+) rather than superoxide radicals (O2˙−) or photogenerated electrons.
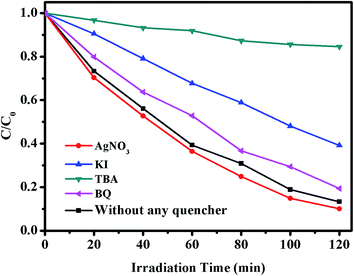 |
| Fig. 8 Photocatalytic degradation of phenol over the 1.5 wt% Pd/BSFO sample alone, and with the addition of different types of active species scavengers (i.e., AgNO3, KI, TBA and BQ) under visible light (λ ≥ 420 nm) irradiation. | |
To elucidate the electronic structure of as-prepared photocatalysts, electrochemical flat potential measurements were carried out, and the data were plotted in Mott–Schottky (MS) spectra, as shown in Fig. 9(a). The positive slopes of the MS plots confirm that the as-prepared BFO, Pd/BFO, BSFO and Pd/BSFO were n-type semiconductors. Meanwhile, the flat-band potential values are generally determined using the Mott–Schottky equation (eqn (2)):44
|
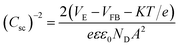 | (2) |
where
Csc is the space charge layer capacitance of the dielectric electrode,
e is the electron charge,
ε is the dielectric constant of the semiconductor,
ε0 is the vacuum permittivity,
ND is the donor density,
A is the photoactive area,
VE is the applied bias potential,
VFB is the flat band potential,
K is the Boltzmann constant,
T is the temperature. The
VFB value can be determined from the extrapolation to 1/
C2 = 0. As
Fig. 9(a) illustrates, the flat band potentials for BFO, 1.5 wt% Pd/BFO, BSFO and 1.5 wt% Pd/BSFO were calculated to be −0.27 V, −0.28 V, −0.35 V and −0.37 V
versus saturated calomel electrode (SCE), respectively. According to the following equation (
eqn (3)),
45 |
E(NHE) = E(SCE) + (0.059 × pH) + 0.241
| (3) |
these potentials could be converted to +0.37, +0.36, +0.29 and +0.27 V
versus NHE (at pH = 0). Assuming the gap between flat band potential and bottom edge of conduction band (CB) is negligible for n-type semiconductors,
46,47 the CB positions of BFO, 1.5 wt% Pd/BFO, BSFO and 1.5 wt% Pd/BSFO could be estimated as +0.37, +0.36, +0.29 and +0.27 eV (
vs. NHE). By taking into account the results of UV-vis DRS absorption, the valence band (VB) positions for BFO, 1.5 wt% Pd/BFO, BSFO and 1.5 wt% Pd/BSFO were calculated to be +2.57, +2.54, +2.43 and +2.35 eV, respectively.
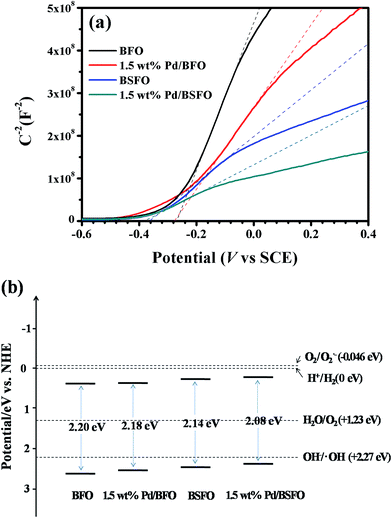 |
| Fig. 9 (a) The flat band potential measurement based on Mott–Schottky analysis; (b) schematic diagram for specific band positions of the obtained photocatalysts. | |
From the above calculated band edge positions, we can depict a possible energy level diagram for the as-prepared photocatalysts as shown in Fig. 9(b). It can be seen that the CB potentials of BFO, Pd/BFO, BSFO and Pd/BSFO were less negative than the potential E0(O2/O2˙−) (−0.046 V vs. NHE48) (eqn (4)), suggesting that the generated CB electrons on the photocatalyst surface could not reduce O2 to yield O2˙−. When combined with two protons (H+), however, the generated electrons might take part in the O2 reduction to form H2O2, which would subsequently convert to hydroxyl radicals,49 since the CB potential of as-prepared photocatalyst was negative than the potential of E0(O2/H2O2) (+0.68 V vs. NHE50) (eqn (5)). On the other hand, the separated photo-generated holes when moving to the surface of the photocatalyst could react with H2O to form ˙OH (eqn (6)), as the VB potential of photocatalysts were positive enough compared to the standard reaction potential of OH−/˙OH (+2.27 V vs. NHE48). Consequently, it could be inferred that ˙OH and h+ could be responsible for the decomposition of organic pollutant (eqn (7) and (8)), which was in consistent with the above trapping experiments. Eventually, the organic pollutants could be mineralized into CO2, H2O, or inorganic ions.
|
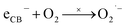 | (4) |
|
O2 + 2e− + 2H+ → H2O2 → 2˙OH
| (5) |
|
˙OH + organic pollutant → degradation product
| (7) |
|
hVB+ + organic pollutant → degradation product
| (8) |
On the basis of the above discussion, a possible mechanism for the enhanced photocatalytic activities of Pd/BSFO hybrid photocatalysts was proposed and schematically illustrated in Fig. 10(a). As shown, the Pd/BSFO particles could be excited by visible light (λ ≥ 420 nm) to produce photogenerated electron–hole pairs. Due to the easy recombination of photoexcited electron–hole pairs and the difficulty in charge transport within BFO particles, only few electrons and holes could reach the BFO particle surface to participate in the degradation reactions, leading to a poor degradation activity of MO or phenol, as seen in Fig. 6 and S1.† For the Pd/BSFO photocatalyst, nevertheless, the rare earth (Sm) dopant in Pd/BSFO would serve as electron traps51 which could capture excited electrons and support the separation of electron–hole pairs, thus facilitating the charge transfer from the bulk BFO to the surface of the photocatalyst. This factor could probably contribute to the improved photocatalytic activity of Pd/BSFO. Furthermore, when the nanosized Pd cocatalyst particles were deposited on the surface of BFO or BSFO particles, the Schottky barrier heterojunction52 could be constructed between metallic Pd nanoparticles and semiconductor BFO or BSFO particles, as shown in Fig. 10(b). Under this circumstance, the photogenerated electrons could be effectively transferred from BFO or BSFO to the Pd particle surface, which could further facilitate the separation of photogenerated electron–hole pairs. This could make a major contribution to the enhanced photocatalytic activity of Pd/BSFO. Additionally, the deposited noble metal cocatalyst on the photocatalyst surface might also be excited by the localized SPR with visible light absorption, and subsequently these excited electrons would act as redox centres to initiate photocatalytic reactions.53,54 Therefore, the SPR effect of the deposited Pd might also to some extent enhance the photocatalytic activity of Pd/BSFO photocatalyst.
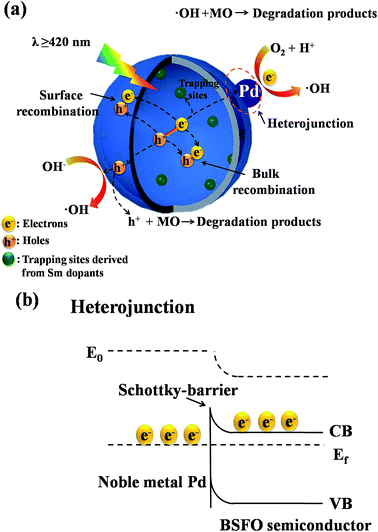 |
| Fig. 10 (a) Schematic diagram for the proposed visible light photocatalytic mechanism of Pd/BSFO samples; (b) schematic illustration of Schottky barrier formed at the interface between Pd and BSFO. | |
The effective separation of photogenerated electron–hole pairs as well as suppression of charge recombination in Pd/BSFO was confirmed by photoluminescence (PL) emission spectra, photocurrent action spectra and electrochemical impedance spectra. As known, the PL emission intensity is related to the recombination of excited electrons and holes, and the lower emission intensity is indicative of a decrease in recombination probability.12,55 Fig. 11(a) shows the PL spectra of as-prepared photocatalysts at an excitation wavelength of 407 nm. It can be seen that the emission band for the photocatalysts was centered at about 668 nm, originating from the radiative recombination process of self-trapped excitations.56 The PL intensities decreased successively in the order of BFO, 1.5 wt% Pd/BFO, BSFO, 2.0 wt% Pd/BSFO, 1.0 wt% Pd/BSFO, and 1.5 wt% Pd/BSFO, indicating that the Pd/BSFO sample exhibited an enhanced ability in capturing photoinduced electrons compared to pure BFO, Pd/BFO or BSFO. The lower PL intensity of Pd/BSFO samples implied a delay in recombination rate and, thus, higher photocatalytic activity. Among all the prepared photocatalysts, the 1.5 wt% Pd/BSFO sample had the lowest PL intensity, indicating the highest photocatalytic activity in agreement with the photocatalytic degradation results. It should be noted that the 2.0 wt% Pd/BSFO sample exhibited a higher PL emission intensity than the 1.5 wt% Pd/BSFO sample. This could be ascribed to the fact that the excess amount of Pd in Pd/BSFO could produce much more surface defects, which would capture the photoinduced electrons to further generate excitons,57 thus leading to the enhancement of PL emission intensity. The transient photocurrent response of a photocatalyst may directly correlate to the separation and transport efficiency of the photogenerated carriers.58 Fig. 11(b) shows the photocurrent responses measured for the prepared photocatalysts as a function of time with light-on and light–off cycles under visible light irradiation (λ ≥ 420 nm). It can be seen that Pd/BSFO samples exhibited a much higher photocurrent than pure BFO, Pd/BFO and BSFO, indicating a much more efficient photoinduced charge separation and transfer process in the Pd/BSFO sample. Also, the 1.5 wt% Pd/BSFO sample displayed the highest photocurrent intensity, which was 4.5 times that of the BFO sample, 3.6 times that of the 1.5 wt% Pd/BFO sample, or 2.8 times that of the BSFO sample. As expected, the trends in the photocurrent response of the prepared samples were in agreement with the photocatalytic degradation results. The enhanced photocurrent response of the Pd/BSFO sample could be ascribed to the much more efficient charge transfer and separation of the photogenerated electron–hole pairs arising from the synergetic effect of Sm doping and Pd cocatalyst loading. To further reveal the synergetic effect of Sm doping and Pd cocatalyst loading on the photoinduced charge separation efficiency of BFO, the typical electrochemical impedance spectra (EIS) of as-prepared photocatalyst samples were also performed. Fig. 11(c) shows the typical EIS spectra for as-prepared photocatalysts under visible light irradiation (λ ≥ 420 nm). As is well-known, the arc radius in the impedance spectrum can reflect the reaction rate on the photocatalyst surface, and the smaller arc radius implies a more efficient separation of photogenerated electron–hole pairs and a higher efficiency of charge transfer across the electrode/electrolyte interface.59 As shown, the arc radii of the prepared Pd/BFO and BSFO sample electrodes were smaller than that of pure BFO electrode, suggesting that either the Pd cocatalyst loading or the Sm doping in BFO could facilitate the separation and migration of photogenerated electron–hole pairs in comparison with pure BFO. Moreover, a further decrease in the arc radius of the Nyquist plot was observed for the Pd/BSFO samples, confirming the much more efficient separation of photogenerated electron–hole pairs in Pd/BSFO. Therefore, the PL spectra, photocurrent action spectra and EIS measurements demonstrate that the combination of Pd cocatalyst loading and Sm doping could significantly promote the separation of photogenerated electron–hole pairs and suppress the recombination of photogenerated electron–hole pairs in the Pd/BSFO samples, thus improving the photocatalytic activities.
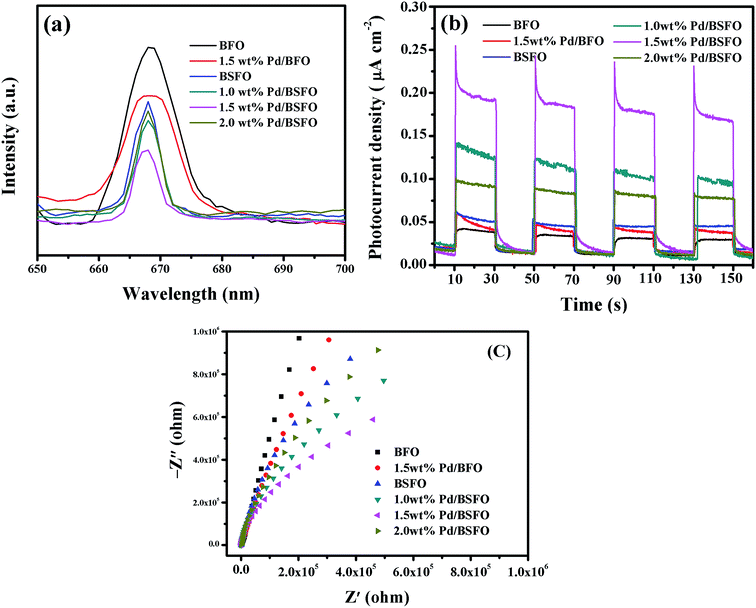 |
| Fig. 11 (a) PL emission spectra, (b) photocurrent action spectra and (c) EIS Nyquist plots of the prepared photocatalyst samples. | |
4. Conclusions
In summary, the synergetic effect of Sm doping and Pd cocatalyst loading on the photocatalytic activity of BFO was investigated for the first time. A series of novel Pd cocatalyst-loaded and Sm-doped BFO (Pd/BSFO) composite photocatalysts containing different Pd loading contents were successfully prepared by using a sol–gel method followed by an impregnation process. The obtained products were characterized by a variety of techniques including XRD, SEM, TEM and XPS. It was found that the rhombohedral structure of BFO was distorted by Sm substitution, and Pd cocatalyst nanoparticles were well distributed on the surface of BSFO. Compared to the BFO sample, the Pd/BSFO samples displayed significantly enhanced visible-light absorption accompanying with a much lower band gap, which could be ascribed to the rearrangement of the molecular orbital and distortion in FeO6 octahedral induced by Sm doping as well as the surface plasmon resonance (SPR) effect of Pd cocatalyst. More importantly, the Pd/BSFO composite photocatalysts exhibited much higher photocatalytic activities for photodegradation of MO or phenol compared to pure BFO, BSFO and Pd/BFO sample. When the Pd loading content was 1.5 wt%, the optimal photocatalytic degradation efficiency was achieved. The enhanced photocatalytic activity of the Pd/BSFO photocatalyst could be attributed to the increased optical absorption, the efficient charge transfer and separation as well as the suppressed recombination of photogenerated electron–hole pairs derived from the Sm dopant trapping level and the heterojunction formation of the Schottky barrier between BSFO and Pd cocatalyst in Pd/BSFO, as confirmed by PL emission spectra, photocurrent action spectra, and EIS measurements. In addition, on the basis of the calculated band edge positions and trapping experiments, the enhanced photocatalytic mechanism of Pd/BSFO was also discussed.
Acknowledgements
This work is financially supported by the National Natural Science Foundation of China (No. 51372237, 51572250), International S&T Cooperation Program of China (No. 2013DFG52490), Public Welfare Project of Science & Technology Department of Zhejiang Province (No. 2014C31026), Research Project of Public Welfare Quality Testing Industry of China (No. 201510072) and Zhejiang Provincial Higher School Talent Project (No. PD2013183).
References
- M. M. Khin, A. S. Nair, V. J. Babu, R. Murugan and S. Ramakrishna, A review on nanomaterials for environmental remediation, Energy Environ. Sci., 2012, 5, 8075–8109 CAS.
- A. Kudo and Y. Miseki, Heterogeneous photocatalyst materials for water splitting, Chem. Soc. Rev., 2009, 38, 253–278 RSC.
- X. Li, J. G. Yu, J. X. Low, Y. P. Fang, J. Xiao and X. B. Chen, Engineering heterogeneous semiconductors for solar water splitting, J. Mater. Chem. A, 2015, 3, 2485–2534 CAS.
- G. Catalan and J. F. Scott, Physics and applications of bismuth ferrite, Adv. Mater., 2009, 21, 2463–2485 CrossRef CAS.
- T. Rojac, A. Bencan, B. Malic, G. Tutuncu, J. L. Jones, J. E. Daniels and D. Damjanovic, BiFeO3 ceramics: processing, electrical, and electromechanical properties, J. Am. Ceram. Soc., 2014, 97, 1993–2011 CrossRef CAS.
- F. Gao, X. Y. Chen, K. B. Yin, S. Dong, Z. F. Ren, F. Yuan, T. Yu, Z. G. Zou and J. M. Liu, Visible-light photocatalytic properties of weak magnetic BiFeO3 nanoparticles, Adv. Mater., 2007, 19, 2889–2892 CrossRef CAS.
- S. Li, Y. H. Lin, B. P. Zhang, Y. Wang and C. W. Nan, Controlled fabrication of BiFeO3 uniform microcrystals and their magnetic and photocatalytic behaviors, J. Phys. Chem. C, 2010, 114, 2903–2908 CAS.
- T. Gao, Z. Chen, Y. X. Zhu, F. Niu, Q. L. Huang, L. S. Qin, X. G. Sun and Y. X. Huang, Synthesis of BiFeO3 nanoparticles for the visible-light induced photocatalytic property, Mater. Res. Bull., 2014, 59, 6–12 CrossRef CAS.
- C. M. Cho, J. H. Noh, I. S. Cho, J. S. An and K. S. Hong, Low-temperature hydrothermal synthesis of pure BiFeO3 nanopowders using triethanolamine and their applications as visible-light photocatalysts, J. Am. Ceram. Soc., 2008, 91, 3753–3755 CrossRef CAS.
- I. Papadas, J. A. Christodoulides, G. Kioseogloua and G. S. Armatas, A high surface area ordered mesoporous BiFeO3 semiconductor with efficient water oxidation activity, J. Mater. Chem. A, 2015, 3, 1587–1593 CAS.
- R. Q. Guo, L. Fang, W. Dong, F. G. Zheng and M. R. Shen, Magnetically separable BiFeO3 nanoparticles with a gamma-Fe2O3 parasitic phase: controlled fabrication and enhanced visible-light photocatalytic activity, J. Mater. Chem., 2011, 21, 18645–18652 RSC.
- F. Niu, D. Chen, L. S. Qin, N. Zhang, J. Y. Wang, Z. Chen and Y. X. Huang, Facile synthesis of highly efficient p–n heterojunction CuO/BiFeO3 composite photocatalysts with enhanced visible-light photocatalytic activity, ChemCatChem, 2015, 7, 3279–3289 CrossRef CAS.
- J. Lou and P. A. Maggard, Hydrothermal synthesis and photocatalytic activities of SrTiO3-coated Fe2O3 and BiFeO3, Adv. Mater., 2006, 18, 514–517 CrossRef.
- R. Q. Guo, L. Fang, W. Dong, F. G. Zheng and M. G. Shen, Enhanced photocatalytic activity and ferromagnetism in Gd doped BiFeO3 nanoparticles, J. Phys. Chem. C, 2010, 114, 21390–21396 CAS.
- S. X. Wu, J. Z. Fang, X. X. Xu, Z. Liu, X. M. Zhu and W. C. Xu, Microemulsion synthesis, characterization of highly visible light responsive rare earth-doped Bi2O3, Photochem. Photobiol., 2012, 88, 1205–1210 CrossRef CAS PubMed.
- S. Mohan, B. Subramanian, I. Bhaumik, P. K. Gupta and S. N. Jaisankar, Nanostructured Bi(1−x)Gd(x)FeO3 – a multiferroic photocatalyst on its sunlight driven photocatalytic activity, RSC Adv., 2014, 4, 16871–16878 RSC.
- M. Sakar, S. Balakumar, P. Saravanan and S. Bharathkumar, Compliments of confinements: substitution and dimension induced magnetic origin and band-bending mediated photocatalytic enhancements in Bi1−xDyxFeO3 particulate and fiber nanostructures, Nanoscale, 2015, 7, 10667–10679 RSC.
- S. Li, J. M. Zhang, M. G. Kibria, Z. T. Mi, M. Chaker, D. L. Ma, R. Nechache and F. Rosei, Remarkably enhanced photocatalytic activity of laser ablated Au nanoparticle decorated BiFeO3 nanowires under visible-light, Chem. Commun., 2013, 5856–5858 RSC.
- F. Niu, D. Chen, L. S. Qin, T. Gao, N. Zhang, S. Wang, Z. Chen, J. Y. Wang, X. G. Sun and Y. X. Huang, Synthesis of Pt/BiFeO3 heterostructured photocatalysts for highly efficient visible-light photocatalytic performances, Sol. Energy Mater. Sol. Cells, 2015, 143, 386–396 CrossRef CAS.
- R. Li, H. Han, F. Zhang, D. Wang and C. Li, Highly efficient photocatalysts constructed by rational assembly of dual-cocatalysts separately on different facets of BiVO4, Energy Environ. Sci., 2014, 7, 1369–1376 CAS.
- F. Q. Zhou, Y. L. Min, J. C. Fan and Q. J. Xu, Reduced graphene oxide-grafted cylindrical like W doped BiVO4 hybrids with enhanced performances for photocatalytic applications, Chem. Eng. J., 2015, 266, 48–55 CrossRef CAS.
- Y. L. Yu, T. He, L. J. Guo, Y. J. Yang, L. M. Guo, Y. Tang and Y. Cao, Efficient visible-light photocatalytic degradation system assisted by conventional Pd catalysis, Sci. Rep., 2015, 5, 9561 CrossRef CAS PubMed.
- S. Pattanayak, R. N. P. Choudhary and P. R. Das, Effect of Sm-substitution on structural, electrical and magnetic properties of BiFeO3, Electron. Mater. Lett., 2014, 10, 165–172 CrossRef CAS.
- J. Liu, L. Fang, F. G. Zheng, S. Ju and M. R. Shen, Enhancement of magnetization in Eu doped BiFeO3 nanoparticles, Appl. Phys. Lett., 2009, 95, 022511 CrossRef.
- Z. H. N. Al-Azri, W. T. Chen, A. Chan, V. Jovic, T. Ina, H. Idriss and G. I. N. Waterhouse, The roles of metal co-catalysts and reaction media in photocatalytic hydrogen production: Performance evaluation of M/TiO2 photocatalysts (M = Pd, Pt, Au) in different alcohol-water mixtures, J. Catal., 2015, 329, 355–367 CrossRef CAS.
- H. Y. Li, Z. X. Cai, J. C. Ding and X. Guo, Gigantically enhanced NO sensing properties of WO3/SnO2 double layer sensors with Pd decoration, Sens. Actuators, B, 2015, 220, 398–405 CrossRef CAS.
- X. Zhu, Q. Hang, Z. Xing, Y. Yang, J. Zhu, Z. Liu, N. Ming, P. Zhou, Y. Song, Z. Li, T. Yu and Z. Zou, Microwave hydrothermal synthesis, structural characterization, and visible-light photocatalytic activities of single-crystalline bismuth ferric nanocrystals, J. Am. Ceram. Soc., 2011, 94, 2688–2693 CrossRef CAS.
- W. B. Luo, J. Zhu, Y. R. Li, X. P. Wang, D. Zhao, J. Xiong and Y. Zhang, Effects of chemical fluctuations on microstructures and properties of multiferroic BiFeO3 thin films, Appl. Phys. Lett., 2007, 91, 082501 CrossRef.
- Y. B. Ding, F. Yang, L. H. Zhu, N. Wang and H. Q. Tang, Bi3+ self doped NaBiO3 nanosheets: facile controlled synthesis and enhanced visible light photocatalytic activity, Appl. Catal., B, 2015, 164, 151–158 CrossRef CAS.
- T. D. Nguyen, D. Mrabet and T. O. Do, Controlled self-assembly of Sm2O3 nanoparticles into nanorods: simple and large scale synthesis using bulk Sm2O3 powders, J. Phys. Chem. C, 2008, 112, 15226–15235 CAS.
- C. Bigey, L. Hilaire and G. Maire, Catalysis
on Pd/WO3 and Pd/WO2: effect of the modifications of the surface states due to redox treatments on the skeletal rearrangement of hydrocarbons: Part I. physical and chemical characterizations of catalysts by BET, TPR, XRD, XAS, and XPS, J. Catal., 1999, 184, 406–420 CrossRef CAS.
- L. F. Fei, J. K. Yuan, Y. M. Hu, C. Z. Wu, J. L. Wang and Y. Wang, Visible light responsive perovskite BiFeO3 pills and rods with dominant {111}c facets, Cryst. Growth Des., 2011, 11, 1049–1053 CAS.
- N. H. Hong, N. T. Huong, T. Y. Kim, S. Goumri-Said and M. B. Kanoun, Tuning magnetic properties of BiFeO3 thin films by controlling rare-earth doping: experimental and first-principles studies, J. Phys. Chem. C, 2015, 119, 14351–14357 CAS.
- K. Sayama, A. Nomura, T. Arai, T. Sugita, R. Abe, M. Yanagida, T. Oi, Y. Iwasaki, Y. Abe and H. Sugihara, Photoelectrochemical decomposition of water into H2 and O2 on porous BiVO4 thin-film electrodes under visible light and significant effect of Ag ion treatment, J. Phys. Chem. B, 2006, 110, 11352–11360 CrossRef CAS PubMed.
- M. Chen, B. H. Wu, J. Yang and N. F. Zheng, Small adsorbate-assisted shape control of Pd and Pt nanocrystals, Adv. Mater., 2012, 24, 862–879 CrossRef CAS PubMed.
- J. C. Yu, J. G. Yu, W. K. Ho, Z. T. Jiang and L. Z. Zhang, Effects of F-doping on the photocatalytic activity and microstructures of nanocrystalline TiO2 powders, Chem. Mater., 2002, 14, 3808–3816 CrossRef CAS.
- B. Ohtani, Photocatalysis A to Z—What we know and what we do not know in a scientific sense, J. Photochem. Photobiol., C, 2010, 11, 157–178 CrossRef CAS.
- A. B. Ahmed, B. Jibril, S. Danwittayakul and J. Dutta, Microwave-enhanced degradation of phenol over Ni-loaded ZnO nanorods catalyst, Appl. Catal., B, 2014, 156–157, 456–465 CrossRef CAS.
- J. Levec and A. Pintar, Catalytic wet-air oxidation processes: A review, Catal. Today, 2007, 124, 172–184 CrossRef CAS.
- W. J. Li, D. Z. Li, Y. M. Lin, P. X. Wang, W. Chen, X. Z. Fu and Y. Shao, Evidence for the active species involved in the photodegradation process of methyl orange on TiO2, J. Phys. Chem. C, 2012, 116, 3552–3560 CAS.
- R. Palominos, J. Freer, M. A. Mondaca and H. D. Mansilla, Evidence for hole participation during the photocatalytic oxidation of the antibiotic flumequine, J. Photochem. Photobiol., A, 2008, 193, 139–145 CrossRef CAS.
- K. L. Lv and Y. M. Xu, Effects of polyoxometalate and fluoride on adsorption and photocatalytic degradation of organic dye X3B on TiO2: the difference in the production of reactive species, J. Phys. Chem. B, 2006, 110, 6204–6212 CrossRef CAS PubMed.
- Y. C. Huang, H. B. Li, M. S. Balogun, W. Y. Liu, Y. X. Tong, X. H. Lu and H. B. Ji, Oxygen vacancy induced bismuth oxyiodide with remarkably increased visible-light absorption and superior photocatalytic performance, ACS Appl. Mater. Interfaces, 2014, 6, 22920–22927 CAS.
- K. P. S. Parmar, H. J. Kang, A. Bist, P. Dua, J. S. Jang and J. S. Lee, Photocatalytic and photoelectrochemical water oxidation over metal-doped monoclinic BiVO4 photoanodes, ChemSusChem, 2012, 5, 1926–1934 CrossRef CAS PubMed.
- S. J. Hong, S. Lee, J. Suk Jang and J. S. Lee, Heterojunction BiVO4/WO3 electrodes for enhanced photoactivity of water oxidation, Energy Environ. Sci., 2011, 4, 1781–1787 CAS.
- S. Cho, J. W. Jang, K. J. Kong, E. S. Kim, K. H. Lee and J. S. Lee, Anion-doped mixed metal oxide nanostructures derived from layered double hydroxide as visible light photocatalysts, Adv. Funct. Mater., 2013, 23, 2348–2356 CrossRef CAS.
- D. Wang, T. Kako and J. Ye, Efficient photocatalytic decomposition of acetaldehyde over a solid-solution perovskite (Ag0.75Sr0.25)(Nb0.75Ti0.25) O3 under visible-light irradiation, J. Am. Chem. Soc., 2008, 130, 2724–2725 CrossRef CAS PubMed.
- H. F. Cheng, B. B. Huang, Y. Dai, X. Y. Qin and X. Y. Zhang, One-step synthesis of the nanostructured AgI/BiOI composites with highly enhanced visible-light photocatalytic performances, Langmuir, 2010, 26, 6618–6624 CrossRef CAS PubMed.
- C. L. Muhich, Y. Zhou, A. M. Holder, A. W. Weimer and C. B. Musgrave, Effect of surface deposited Pt on the photoactivity of TiO2, J. Phys. Chem. C, 2012, 116, 10138–10149 CAS.
- A. J. Bard, R. Parsons and J. Jordan, Standard potentials in aqueous solution, CRC press, 1985 Search PubMed.
- M. Machida, S. Murakami and T. Kijima, Photocatalytic property and electronic structure of lanthanide tantalates, LnTaO4 (Ln = La, Ce, Pr, Nd, and Sm), J. Phys. Chem. B, 2001, 105, 3289–3294 CrossRef CAS.
- J. H. Yang, D. G. Wang, H. X. Han and C. Li, Roles of cocatalysts in photocatalysis and photoelectrocatalysis, Acc. Chem. Res., 2013, 46, 1900–1909 CrossRef CAS PubMed.
- J. Q. Yan, G. J. Wu, N. J. Guan and L. D. Li, Synergetic promotion of the photocatalytic activity of TiO2 by gold deposition under UV-visible light irradiation, Chem. Commun., 2013, 49, 11767–11769 RSC.
- J. A. Scholl, A. L. Koh and J. A. Dionne, Quantum plasmon resonances of individual metallic nanoparticles, Nature, 2012, 483, 421–427 CrossRef CAS PubMed.
- V. Etacheri, M. K. Seery, S. J. Hinder and S. C. Pillai, Highly visible light active TiO2−xNx heterojunction photocatalysts, Chem. Mater., 2010, 22, 3843–3853 CrossRef CAS.
- A. Zhu, Q. Zhao, X. Li and Y. Shi, BiFeO3/TiO2 nanotube arrays composite electrode: construction, characterization, and enhanced photoelectrochemical properties, ACS Appl. Mater. Interfaces, 2014, 6, 671–679 CAS.
- L. Q. Jing, Y. C. Qu, B. Q. Wang, S. D. Li, B. J. Jiang, L. B. Yang, W. Fu, H. G. Fu and J. Z. Sun, Review of photoluminescence performance of nano-sized semiconductor materials and its relationships with photocatalytic activity, Sol. Energy Mater. Sol. Cells, 2006, 90, 1773–1787 CrossRef CAS.
- D. M. Chen, K. W. Wang, W. Z. Hong, R. L. Zong, W. Q. Yao and Y. F. Zhu, Visible light photoactivity enhancement via CuTCPP hybridized g-C3N4 nanocomposite, Appl. Catal., B, 2015, 166–167, 366–373 CrossRef CAS.
- Y. H. Lv, Y. Y. Zhu and Y. F. Zhu, Enhanced photocatalytic performance for the BiPO4−x nanorod induced by surface oxygen vacancy, J. Phys. Chem. C, 2013, 117, 18520–18528 CAS.
Footnote |
† Electronic supplementary information (ESI) available. See DOI: 10.1039/c6ra01140c |
|
This journal is © The Royal Society of Chemistry 2016 |