DOI:
10.1039/C6RA01102K
(Paper)
RSC Adv., 2016,
6, 23058-23073
Naringin ameliorates radiation-induced hepatic damage through modulation of Nrf2 and NF-κB pathways†
Received
13th January 2016
, Accepted 15th February 2016
First published on 15th February 2016
Abstract
Understanding the mechanism of ionizing radiation (IR)-induced systemic stress and its modulation by phytocomponents has great significance for the development of novel phyto-radioprotectors. The present study was intended to evaluate the radioprotective effect of naringin (NG), a citrus flavonoid on the modulation of IR-induced activation of the redox-regulated signaling system in murine liver. On the basis of survival analysis, mice were treated with 75 mg kg−1 body weight of NG for three consecutive days before irradiation (6 Gy). Pretreatment with NG significantly prevented the IR-induced generation of intracellular reactive oxygen species (ROS), along with the formation of hepatic thiobarbituric acid reactive substances (TBARS) and cellular nitrite. NG also showed significant reduction in IR-induced nuclear DNA damage through the inhibition of DNA-dependent protein kinase (DNA-PK). Further, the IR-induced cell death was arrested in the presence of NG through the inhibition of p53 mediated stress-activated protein kinase/Jun amino-terminal kinase (SAPK/JNK) pathways and modulation of other molecules of apoptosis pathways. Moreover, NG supported the intracellular defense mechanisms, by maintaining the endogenous antioxidants probably through phosphoinositide 3-kinase/protein kinase-B (PI3K/Akt) guided transcriptional activation of nuclear factor (erythroid-derived 2)-like 2 (Nrf2). In addition, NG inhibited IR-induced inflammation through suppression of NF-κB (nuclear factor kappa-light-chain-enhancer of activated B cells) followed by the alteration of pro inflammatory factors. Taken together, these results suggested that NG reversed the IR-induced redox-imbalance in murine liver, probably by the inhibition of ROS/p38-MAPK/NF-κB, along with the activation of the PI3K/Akt/Nrf2 pathway and the reduction of apoptosis by interfering with the p53/SAPK/JNK/Bax pathway.
Introduction
In recent times, ionizing radiation (IR) has been chiefly used in radiotherapy for cancer treatment to target cancer cells and to halt tumor progression. It also induces normal tissue injury as a side effect through alteration of the intracellular materials, resulting in cell death.1 It is generally accepted that IR-induced cellular damage can be caused by the direct action on DNA molecules or indirectly by the production of ROS, which ultimately damages the lipids, proteins and other molecules of cellular structure.2 Hence IR can be considered as a “two-edged sword”.3 Generation of ROS creates oxidative stress and perturbs redox homeostasis within the cells.3 Imbalance of redox homeostasis leads to the modulation of stress associated cellular signaling system that triggers the inflammation, growth arrest and apoptosis.4 DNA-PK/p53 pathway is one of the earliest sensor in IR-induced DNA damage and also responsible for the recognition and subsequent initiation of downstream protein kinase cascades leading to the apoptosis response.5 Another important redox-regulated signaling pathway involves in IR-induced cellular anomalies is NF-κB.4 NF-κB is one of the major redox-sensitive transcription factors that control the expression of hundreds of genes which are involved in regulating cell growth, differentiation, development, apoptosis and inflammation.4 To counteract oxidative stress, cells have complicated mechanisms of defense against this toxicity. One of the most important mechanism is the activation of Keap1/Nrf2 pathway which leads to the modulation of cytoprotective enzymes, such as glutathione-s-transferase (GST), manganese-dependent superoxide dismutase (Mn-SOD), NAD(P)H: quinone oxidoreductase 1 (NQO1) and heme oxygenase 1 (HO-1).6
Understanding the specific biochemical and molecular mechanisms following radiation as well as how these processes can be manipulated, holds great promise for identifying novel strategies to enhance therapeutic responses to radiation, mitigates the deleterious effects of radiation, as well as gather mechanistic information critical for radiation risk assessment.
Consequently, in the past few decades radioprotection through dietary phytochemicals has become an increasingly active area of research as numerous synthetic and semi synthetic compounds show plethora of side effects.7 Naringin (4′,5,7-trihydroxy flavanone 7-rhamnoglucoside) is a widely known bioflavonoid derived from numerous citrus fruits and related citrus species which possess metal-chelating, antioxidant and free radical scavenging properties.4 Naringin has been evaluated for a wide spectrum of bioefficacy including anticancer, anti-inflammatory, antibacterial and cardioprotective activity.8 Recently, we have reported that naringin can prevent IR-induced inflammation and abnormal cellular growth arrest in murine splenocytes through the modulation of NF-κB and p53 pathway.4 Earlier reports showed that NG had the potential to scavenge IR-mediated free radicals and protect DNA in vitro.9 So far there are several reports delineating the molecular mechanism of action of NG on the protection against radiation toxicity using in vivo model. Therefore, the current investigation is to evaluate the cytoprotective efficacy of NG against IR-induced cellular anomalies in the murine liver with the exploration of possible mechanisms involved in this process.
Experimental
Chemicals
Naringin (purity >98%, as determined by HPLC) and other analytical grade chemicals were purchased from Sigma-Aldrich Corporation (St Louis, MO, USA). Anti-mouse/rabbit monoclonal/polyclonal primary antibodies and secondary antibodies (both alkaline phosphatase and fluorophore tagged) were purchased from Cell Signaling Technology (Danvers, MA, USA). Revert Aid M-MuLV reverse transcriptase, oligo dT and RNase inhibitor and other chemicals required for c-DNA synthesis were purchased from Thermo Fisher scientific (Waltham, Massachusetts, USA). TNF-α, IL-6 and IFN-γ sandwich enzyme-linked immunosorbent assay (ELISA) kits were obtained from eBioscience Inc (San Diego, CA, USA).
Animals
Male Swiss albino mice (4–6 weeks old and weighing 22–25 g) were obtained from animal facility unit of Chittaranjan National Cancer Institute, Kolkata, India. Animals were kept under standard conditions of temperature and humidity in the departmental animal house facility and provided with standard laboratory diet and water ad libitum. All animal experiments in this study were carried out with the prior approval of the Institutional Animal Ethics Committee (IAEC-III/proposal/SD-11/2012 dated 28.04.2012), strictly adhering to the guidelines of Committee for the Purpose of Control and Supervision of Experiments on Animals (CPCSEA) constituted by the Ministry of Environment and Forest (MoEF), Government of India.
Irradiation of mice
Mice were irradiated using 60Co source in gamma chamber GC900 (Board of Radiation and Isotope Technology, Mumbai) at Saha Institute of Nuclear Physics (SINP), Kolkata, India. Unanaesthetized mice were restrained in well-ventilated perspex boxes and exposed to whole body gamma radiation of 6 Gy, at a dose-rate of 1 Gy min−1 and a source-to-surface distance of 77.5 cm.10
Selection of optimum dose of NG for radioprotection studies
Animals were divided into 6 groups (n = 10) and treated as follows: Group I, sham control (vehicle-treated); Group II, radiation control (6 Gy IR-treated); and Group III to VI, pre-administration of NG at graded doses (25, 50, 75 and 100 mg kg−1 body weight of NG-treated) for 1 h prior to whole body IR (6 Gy). NG was suspended in 10% DMSO in PBS and administered orally (0.2 ml) in desired concentrations once in a day for consecutive three days prior to 6 Gy irradiation. After irradiation, the animals were observed daily for a period of 30 days for the assessment of radiation sickness and mortality. The dose, at which % mortality after 30 days was the least, was selected as the optimum dose for the entire study. Since 75 mg kg−1 body weight of NG exhibited maximum radioprotection, this dose was selected for further studies.
Experimental design
The mice were divided into four groups and each group was consisted of a minimum of six animals.
Control. Mice were given to 10% DMSO in PBS (pH 7.4) through oral gavages (0.2 ml) once in a day for three consecutive days.
IR group. Mice were given to 10% DMSO in PBS (pH 7.4) for three consecutive days before exposing them to a single dose of 6 Gy IR (60Co). All the irradiated animals were necropsied by cervical dislocation at 24 hours of post-irradiation.
NG group. Mice were administered with NG (75 mg kg−1 body weight) orally (0.2 ml) once a day for three consecutive days.
NG + IR group. Mice were administered with NG (75 mg kg−1 body weight) orally (0.2 ml) for three consecutive days. After one hour of the last dose, the animals were exposed to a single dose of 6 Gy IR. All the animals were necropsied by cervical dislocation at 24 hours of post-irradiation.
Tissue homogenate preparation
Liver samples were quickly excised, washed with saline, blotted with a piece of filter paper and homogenized in appropriate radioimmunoprecipitation assay (RIPA) lysis buffer using a sonicator. The homogenates were centrifuged at 800 × g for 5 min at 4 °C to separate the nuclear debris. The supernatant so obtained was centrifuged at 10
500 × g for 20 min at 4 °C to get the post-mitochondrial supernatant which was used for biochemical assays.11
Isolation of hepatocytes
A fresh hepatocytes suspension was obtained by in situ liver perfusion with collagenase (0.05%, p/v) through a two-step perfusion technique.12 Cell viability (80%) was determined by the trypan blue exclusion test and the viable cells were used for the further experiments.
Measurement of serum ALT, AST, ALP and tissue LDH activity
Blood samples were drawn from the orbital venous plexus at 24 hours of post irradiation. Serum was separated by centrifugation for 5 min at 3000 × g. Activities of serum alanine aminotransferase (ALT), aspartate aminotransferase (AST), alkaline phosphatase (ALP) and tissue lactate dehydrogenase (LDH) activity were measured at 24 hours of post irradiation according to the instructions supplied with the commercial assay kits.
Histological assessment
For the light microscopic investigation, liver tissues from each experimental group were fixed in 10% buffered formalin. Then the fixed liver tissues were embedded in paraffin, sectioned, deparaffinized and rehydrated using the standard techniques followed by staining with hematoxylin and eosin (H and E). The extent of liver damage was assessed and scored according to the standard method by examining the magnitude of the hepatic necrosis and infiltration of inflammatory cells in a high power field (objective lens: 20×; magnification: 200×) under a Zeiss Axiovert microscope using Axiovision software (Jena, Germany).11
Estimation of free form of NG in plasma and liver using high performance liquid chromatography (HPLC)
Mice were administered with NG (75 mg kg−1 body weight) orally for three consecutive days. Animals were sacrificed after 1, 6 and 24 hours of last dose of NG administration (as in this study design, mice were exposed to IR after 1 h of last dose of NG administration and sacrificed after 24 hours of irradiation). Plasma and liver tissues were collected at respective time points. Liver was homogenized and used for HPLC according to the previous protocol.13 The retention time for NG was about 13 minutes at 320 nm both in case of plasma and liver.10
Measurement of intercellular reactive oxygen species (ROS)
The harvested hepatocytes (2 × 106) from each group of mice were resuspended in 0.5 ml of complete medium containing oxidation sensitive fluorescent dye, H2DCFDA (10 mM), incubated at 37 °C for an additional 30 min, washed with PBS and analyzed immediately by flow cytometer.14
Evaluation of lipid peroxidation and antioxidant enzyme activities
The tissue homogenate was subjected to further biochemical measurements and the protein content of the tissue homogenate was measured by the method of Lowry et al. using crystalline BSA as standard. Lipid peroxidation was determined by measuring the thiobarbituric acid reactive substance (TBARS) levels in the tissue homogenate using previously standardized method.13 The level of reduced glutathione (GSH), activity of superoxide dismutase (SOD) and catalase (CAT) were also determined according to the standardized laboratory protocol.13
Determination of heme oxygenase-1 (HO-1) and NAD(P)H quinone oxidoreductase 1 (NQO1) activity
HO-1 activity was assessed using the method of Carraway et al. (2000) by monitoring the biliverdin reduction to bilirubin by biliverdin reductase by using the difference in optical density at 464 nm and 530 nm with extinction coefficient of 40 mM−1 cm−1, and the enzymatic activity was expressed as pmol mg−1 of protein per hour.15 NQO1 activity was measured as the dicoumarol-inhibitable fraction of DCPIP reduction according to the method of De Haan et al. (2002) by observing the difference of two readings at 600 nm at room temperature between a 60 s interval and was expressed as the dicoumarol-inhibitable part of DCPIP reduction (nmol DCPIP reduced per min per μg of protein).16
Determination of cellular nitrate formation
Cellular nitrite formation was determined using the Griess reagent following the standardized method described earlier.17
Analysis of protein expression by flow cytometry
Harvested hepatocytes were fixed in 4% paraformaldehyde in PBS (pH 7.4) and permeabilized in 0.1% Triton X-100 in PBS for 5 min. After washing twice in PBS with 3% FBS, permeabilized cells were incubated with primary antibody on ice for 2 h and washed twice in PBS. Cells were then incubated with FITC and APC-conjugated goat anti-rabbit IgG as a secondary antibody for 30 min on ice and washed twice in PBS. The stained cells were acquired and analyzed using BD FACSAria III™ (Becton Dickinson, Franklin Lakes, NJ, USA) equipped with Flow Jo software.4
Detection of apoptosis and necrosis using Annexin V-FITC/DAPI
Briefly, a total of 2 × 106 harvested hepatocytes were suspended in 500 μl binding buffer. Subsequently, 5 μl of Annexin V-FITC and 5 μl of DAPI were added followed by mixing at room temperature in the dark for 15 min. Within 1 h, the percentage of live, apoptotic and necrotic cells were detected by BD FACSAria III™ flow cytometer (Becton Dickinson, Franklin Lakes, NJ, USA).18
Analysis of mitochondrial transmembrane potential (MMP) using JC-1
Briefly, isolated hepatocytes (2 × 106) were incubated with JC-1 (2.5 μM) for 15 min at 37 °C in the dark. Subsequently, stained cells were washed with PBS followed by flow cytometric evaluation (BD FACSAria III™, Becton Dickinson, Franklin Lakes, NJ, USA).18
Immunoblot analysis
Immunoblot experiments were performed using the standard protocol as described earlier.14 Equal amounts of denatured protein fractions (25 μg) were separated by SDS-PAGE on a 12.5% polyacrylamide gel, and then transferred overnight onto polyvinylidene difluoride membranes (PVDF). After appropriate blocking, the membranes were incubated with the primary antibodies overnight and secondary antibodies (alkaline phosphatase-conjugated goat anti-rabbit or anti-mouse secondary antibody) for 2 h. The blots were detected using the NBT-BCIP chromogenic substrate and the photographs were taken Gel Doc™ EZ Imager (Bio-Rad Laboratories, Hercules, CA, USA).14
Determination of caspase-3 and caspase-9 activity
Caspase-3 and 9 activities in the liver tissue homogenate was measured using fluorimetric assay kit according to the manufacture's instruction.
Determination of TNF-α, IL-6 and IFN-γ level
TNF-α, IL-6 and IFN-γ concentrations in the hepatic tissue samples were analyzed using commercially available kits according to the manufacture's protocol.
RNA extraction and reverse transcriptase PCR (RT-PCR)
RNA isolation was performed using the TRIzol® reagent (Invitrogen, Carlsbad, CA, USA) according to the standard protocol. Briefly, through the reverse transcription, the resulting cDNA was used for RT-PCR using the gene specific primers (Table 1). PCR amplification was conducted using a 2720-Thermal Cycler (Applied Biosystem, UK) and 0.5 unit of Taq polymerase was set for 30 cycles (denaturation: at 94 °C, annealing: at 58 °C for 30 seconds and extension: at 72 °C for 1 min and final extension at 72 °C for 7 min).19 GAPDH expression was used as an internal control. Electrophoresis was carried out using agarose gel electrophoresis and stained with ethidium bromide. The stained gels were photographed using Gel Doc EZ Imager (Bio-Rad Laboratories, Hercules, CA, USA) and the resultant gel photographs were analyzed by Image Lab 4.1 Software (Bio-Rad Laboratories, Hercules, CA, USA).4
Table 1 Oligonucleotides for RT-PCR
Target mRNA |
Sequence (5′-3′) |
Tm (°C) |
Product size (bp) |
iNOS |
Forward: GGAGATCAATGTGGCTGT GC |
55 |
496 |
Reverse: AAGGCCAAACACAGCATACC |
MCP1 |
Forward: CCCCAAGAAGGAATGGGTCC |
52 |
155 |
Reverse: TGCTTGAGGTGGTTGTGGAA |
GAPDH |
Forward: CAAGGCTGTGGGCAAGGTCA |
59 |
232 |
Reverse: AGGTGGAAGAGTGGGAGTTGCTG |
Immunohistochemical assessment
Paraffin-embedded blocks of liver tissues were cut into 5 μm sections and mounted onto slides. The sections were deparaffinized with xylene and dehydrated over several series of alcohol. Antigen retrieval was performed by heat-induced antigen retrieval procedure using standard procedure and blocking was performed using 5% BSA in TBS for 4 h at room temperature. Finally the sections were incubated with primary antibody in dilution 1
:
300 at 4 °C overnight in a humidified chamber. The tissue sections were washed with TBST and incubated with FITC and APC conjugated secondary antibody (Santa Cruz Biotechnology, USA) solution (1
:
500) for 2 h at room temperature, and the nucleus was visualized by DAPI (Invitrogen). The images were observed in BD Pathway™ fluorescence (Becton Dickinson, San Jose, CA, USA) and Olympus FV1000 MPE SIM Laser Scanning confocal microscope (Olympus, Tokyo, Japan).11
Statistical analysis
Results were expressed as mean ± standard errors (SEM) of data obtained from at least three replicate experiments. Statistical significance and differences among groups were assessed with one-way analysis of variance using OriginPro 8.0 software (San Diego, CA, USA). p < 0.05 value was considered as significant.
Results
NG increased survival of animal exposed to sub-lethal dose (6 Gy) of IR
The results corresponding to the optimum protective dose of NG on the survival rate of mice estimated after whole-body exposure to a sub-lethal dose of 6 Gy IR is illustrated in Fig. 1A. Oral administration of freshly prepared NG of 25, 50, 75 and 100 mg kg−1 did not induce any death during the 30 days of the observation period. All irradiated animals without NG treatment showed 100% mortality within 17 days and exhibited signs of radiation sickness within 2–4 days after exposure to 6 Gy IR. The main symptoms included a reduction in the food and water intake, irritability, weight loss, emaciation, lethargy, diarrhea and ruffling of hair. The first death in 6 Gy IR-exposed group was observed on day 6 and 50% of the animals were died within 10 days after irradiation. Pretreatment with various concentrations of NG (25, 50, 75 and 100 mg kg−1) showed 30, 40, 70 and 60% survival respectively against 6 Gy IR. This delay was longest for 75 mg kg−1 body weight of NG, where the first death was reported by the day 22 post-irradiation. The shortest delay in mortality was observed for 25 mg kg−1 body weight, where the first death occurred on day 17 post-irradiation. NG of 75 mg kg−1 body weight was found to be the most effective dose maintaining 70% survival up to 30 days. Therefore, the optimum protective dose of NG has been considered to be 75 mg kg−1 body weight, which maintained the survival of mice approximately by 70% when compared to the irradiated group.
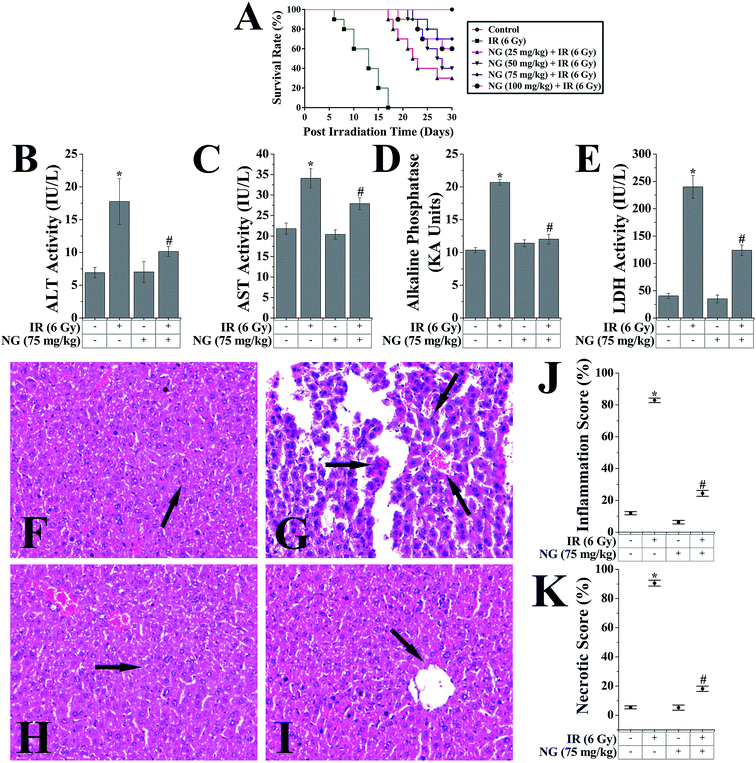 |
| Fig. 1 (A) Kaplan–Meier analysis of 30 days survival of whole body γ-irradiated (6 Gy) mice pre-administered with NG (25, 50, 75 and 100 mg kg−1). Results are presented as mean ± SEM (n = 10). Assessment of liver toxicity markers in 6 Gy irradiated and NG pretreated mice. Bar graph represented as (B) ALT activity, (C) AST activity, (D) ALP activity and (E) LDH activity. Representative images of H&E stained section of (F) control, (G) 6 Gy irradiated, (H) NG (75 mg kg−1) pretreated and (I) NG pretreatment (75 mg kg−1) prior to irradiated hepatic tissue. Each photograph was analyzed by Image J software. Bar graphs are represented as (J) inflammation score and (K) necrotic score. Magnification of H&E stained images were 200×. Values are presented as mean ± SEM (n = 5). p < 0.05 was considered as significant. Statistical comparison: *control vs. IR (6 Gy); #IR (6 Gy) vs. NG (75 mg kg−1) + IR (6 Gy). | |
NG protected IR-induced liver dysfunction
The activities of ALT, AST, ALP and LDH were considered as serum biochemical markers of liver damage. Serum ALT, AST, ALP and LDH levels were measured to determine IR-induced liver damage (Fig. 1B–E). In irradiated mice, serum ALT, AST, ALP and LDH levels were substantially increased by 1.3, 1.56, 2.0 and 4.0-fold respectively as compared to the control group. However, pre-treatment with NG reduced the activities of ALT (1.07-fold), AST (1.28-fold), ALP (1.16-fold) and LDH (2.46-fold) significantly (p < 0.05) as compared with the irradiated mice.
NG restored IR-induced hepatic histopathological alteration
Histopathological studies also provided a good agreement with the results found from serum biochemical marker analysis. Liver sections of control and NG pretreated mice exhibited normal liver architecture (Fig. 1F and H). However, treatment with IR, large areas of extensive loss of hepatic architecture and inflammatory cell infiltration, including intralobular and periportal lymphocyte infiltration and sclerosis of portal areas was found everywhere (Fig. 1G). Extensive hepatocytes necrosis and apoptosis was also observed at a particular area in the IR group (Fig. 1J and K). Pretreatment with NG (75 mg kg−1 body weight) in the irradiated group showed robust protection of hepatocellular necrosis with a poorly dilated central vein and regular arrangement of hepatocytes around the central vein (Fig. 1I). A scattered regenerative zone of hepatocytes and very few necrotic zones were prominent and also no hemodynamic changes were found (Fig. 1I).
Time-dependant changes in plasma and tissue levels of unmetabolised form of NG
To measure the concentration of unmetabolised form of NG in murine plasma and liver after oral administration, high performance liquid chromatography (HPLC) was carried out using an electrochemical detector applying the internal standard (Table 2). HPLC chromatogram of plasma samples of the control group showed that unmetabolised form of NG was not present in the plasma of respective animals (limit of detection 5 ng ml−1). The concentration of unmetabolised form of NG in the plasma was measured after 1, 6 and 24 hours of NG administration at a dose of 75 mg kg−1 body weight. Unmetabolised form of NG concentrations was 87.53, 99.39 and 37 μg ml−1 at the respective time points (Table 2). The availability of unmetabolised form of NG in the liver was also determined at same time points. The concentrations of NG in the liver after 1, 6 and 24 hours were 62.09, 113.13 and 76.07 μg g−1 of tissue respectively (Table 2).
Table 2 Calculated concentrations of free NG after different time point of bioavailability in plasma and hepatic tissue
Sample |
Time (h) |
Retention time (RT) (minutes) |
Area |
% area |
Height |
Concentration (μg ml−1) |
(Arbitrary unit) |
Naringin (standard) |
— |
13.23 |
5 641 323 |
100 |
425 622 |
1000 |
Plasma |
1 |
13.28 |
493 782 |
1.35 |
38 026 |
87.53 |
6 |
13.21 |
560 695 |
1.59 |
41 601 |
99.39 |
24 |
13.33 |
209 220 |
0.60 |
16 930 |
37.09 |
Liver |
1 |
13.36 |
350 249 |
1.22 |
29 354 |
62.09 |
6 |
13.37 |
638 187 |
1.92 |
57 604 |
113.13 |
24 |
13.37 |
429 111 |
1.14 |
25 269 |
76.07 |
NG attenuated IR-induced enhancement of hepatic ROS
The effect of NG on IR-induced intracellular ROS production in the murine liver was determined by flow cytometry. IR-induced ROS generation in hepatic cells from the respective mice in the presence or absence of NG was detected using the fluorescent indicator, H2DCFDA. The relative DCF fluorescence intensity is proportional to the amount of ROS formed intracellularly. As shown in Fig. 2A and B, irradiated mice showed a significant enhancement of intracellular ROS (3.13-fold) over the control group. However, pretreatment with NG (75 mg kg−1) attenuated IR-induced ROS generation in the hepatic cells significantly when compared to the IR group. Only NG pretreated group did not show any enhancement of hepatic ROS (0.99-fold) over the control or IR group.
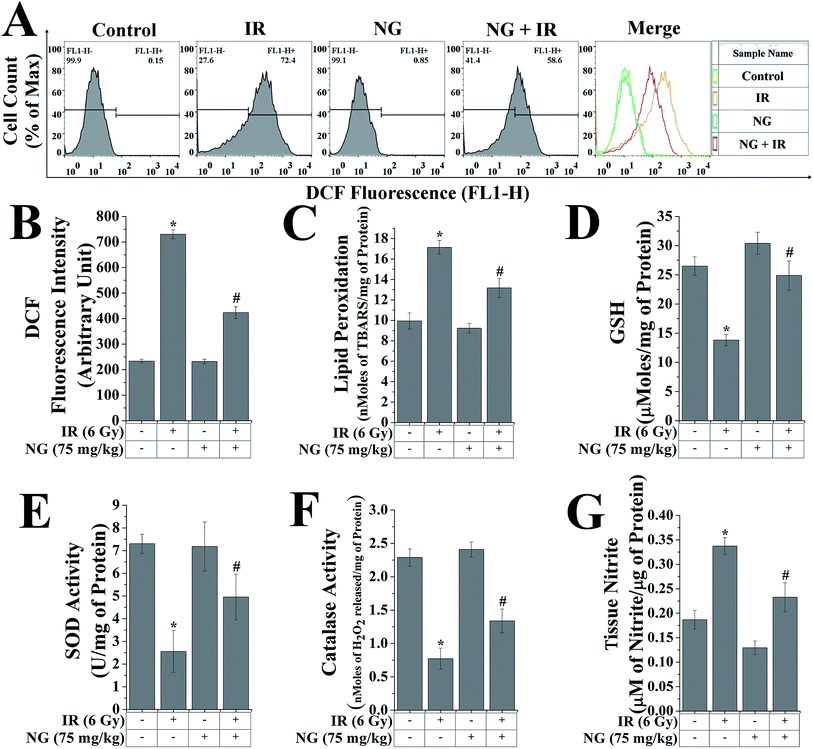 |
| Fig. 2 Assessment of hepatocellular anomalies upon irradiation (6 Gy) and NG pretreatment (75 mg kg−1). (A) Representative histogram showing intercellular DCF fluorescence. (B) Quantitative analysis of relative fluorescence intensity of DCF. Representative bar graphs showing (C) LPO, (D) GSH, (E) SOD activity, (F) catalase activity and (G) tissue nitrite concentration. Values are presented as mean ± SEM (n = 5). p < 0.05 was considered as significant. Statistical comparison: *control vs. IR (6 Gy); #IR (6 Gy) vs. NG (75 mg kg−1) + IR (6 Gy). | |
Reduction of IR-induced enhancement of hepatic lipid peroxidation (LPO) by NG
The levels of thiobarbituric acid reactive substances (TBARS) were significantly increased (p < 0.05) in the IR group (1.72-fold) when compared with the control group. Prior oral administration of NG (75 mg kg−1) along with IR significantly (p < 0.05) lowered the levels of TBARS (1.33-fold) when compared to the IR group. However, only NG pretreated group did not show any enhancement of TBARS level (0.93-fold) over the control or IR group (Fig. 2C).
Enhancement of IR-induced depletion of hepatic non-enzymatic and enzymatic antioxidants by NG
A significant depletion (p < 0.05) of hepatic non-enzymatic (0.52-fold) as well as enzymatic antioxidants (0.35-fold for SOD and 0.34-fold for catalase) were found in murine liver treated with 6 Gy IR when compared to the control group. In contrast, pretreatment with NG (50 mg kg−1) significantly (p < 0.05) restored the suppressed level of these endogenous antioxidants towards normalcy (0.94-fold for GSH, 0.68-fold for SOD and 0.58-fold for catalase) when compared to the IR-treated group. Only NG-pretreated group did not show any significant alteration in GSH, SOD and catalase level over the IR and control group (Fig. 2D–F).
NG suppressed IR-induced enhancement of tissue nitrite
A significant (p < 0.05) augmentation of hepatic tissue nitrite level was seen in IR-treated group when compared to the control group. But this enhancement was reduced with the pretreatment with NG (75 mg kg−1). In NG-pretreated group alone, there was not any enhancement of tissue nitrite over the control group (Fig. 2G).
NG prevented IR-induced enhancement of DNA-PK
DNA-PK, the members of PI3K family, is one of the earliest sensors of IR-induced DNA damage and is also shown to be upstream kinase for p53 activation. Result demonstrated that the relative DNA-PK-FITC fluorescence intensity (4.45-fold) was markedly increased (p < 0.05) in the IR group with respect to the control and NG alone group (0.99-fold). However, NG pretreatment (75 mg kg−1) before irradiation significantly (p < 0.05) decreased the relative FITC fluorescence intensity (1.99-fold) over the IR group (Fig. 3A and B).
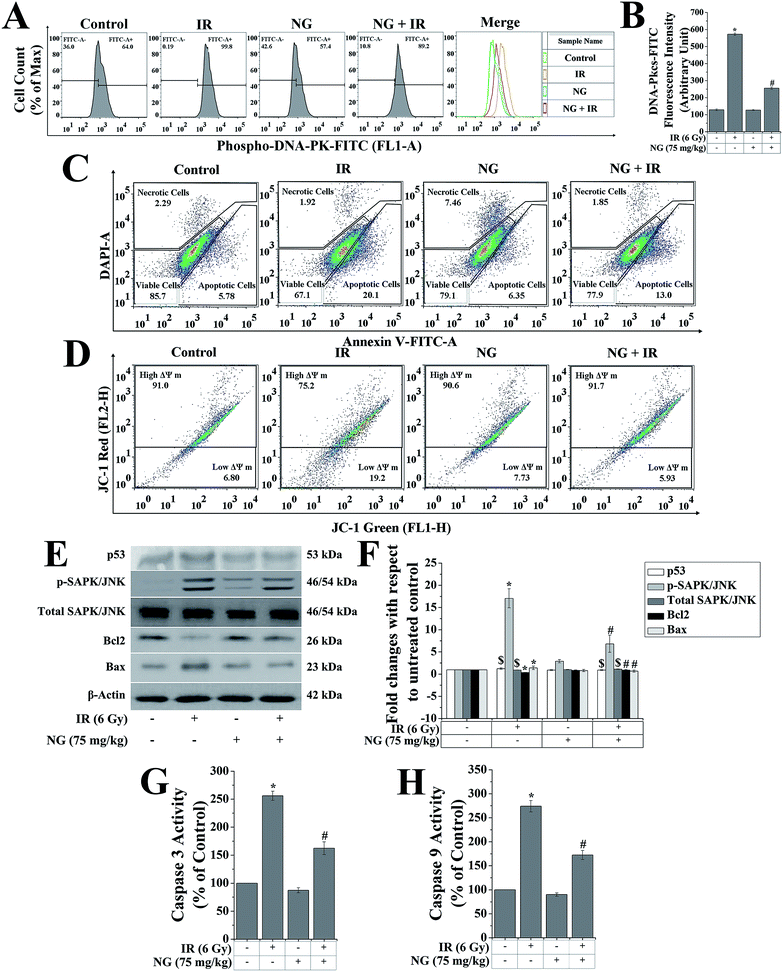 |
| Fig. 3 Assessment of IR-induced (6 Gy) DNA damage/induction of apoptosis and their prevention by NG (75 mg kg−1). (A) Representative flow cytometric histogram showing DNA-PK expression of isolated hepatic cells. (B) Quantitative analysis of DNA-PK-FITC fluorescence intensity. (C) Percent distribution of apoptotic and necrotic cells has been presented in all four quadrants using Annexin-V-FITC binding and DAPI uptake. (D) Mitochondrial membrane potential was evaluated with JC-1 dye. Mitochondrial dependent apoptotic pathway was analyzed using immunoblotting. (E) Representative immunoblots of p53, p-SAPK/JNK, total SAPK/JNK, Bcl2 and Bax. β-Actin was used as an internal control. (F) Relative intensity was obtained from densitometric analysis of respective immunoblots. Bar graphs representing the level of (G) caspase-3 activity and (H) caspase-9 activity. Values are presented as mean ± SEM (n = 5). p < 0.05 was considered as significant. Statistical comparison: *control vs. IR (6 Gy); #IR (6 Gy) vs. NG (75 mg kg−1) + IR (6 Gy) $non significant [control vs. IR (6 Gy) and IR (6 Gy) vs. NG (75 mg kg−1) + IR (6 Gy)]. | |
NG attenuated IR-induced apoptosis and necrosis
To determine the IR-induced apoptosis and necrosis of hepatic cells, flow cytometry was performed using Annexin-V-FITC/DAPI. As shown in Fig. 3C, significant apoptosis induction in hepatic cells was seen after mice exposed to 6 Gy IR. In the control group, 85.7% cells were viable, 5.78% cells were in the early stages of apoptosis induction and 2.29% were in the late stages of apoptosis or dead. After irradiation, the number of viable cells decreased to 67.1% with the concomitant increase in early apoptotic cells (20.1%). However, no significant decrease in the late stage of apoptotic/dead cell (1.92%) was seen in overall cell populations (positive for both Annexin V-FITC and DAPI). Pretreatment with NG reduced both the number of early and late stages of apoptosis upon irradiation to 13.0% and 1.85% respectively. These results indicated that NG has the potential to reduce the IR-induced apoptosis in hepatic cells.
NG attenuated IR-induced loss of mitochondrial transmembrane potential (MMP)
Alterations in mitochondrial integrity and function may play an important role in the apoptosis cascade. As demonstrated in Fig. 3D, exposure of mice to 6 Gy IR caused a substantial loss in hepatic mitochondrial membrane potential, in accordance with a decrease in JC-1 red-green fluorescence intensity ratio. This result indicated that IR substantially reduced the mitochondrial membrane potential. In contrast, pretreatment with NG prior to 6 Gy IR significantly (p < 0.05) attenuated this depolarization. These data indicated the protective role of NG against IR-induced mitochondrial damage.
NG attenuated IR-induced p53 activation and apoptosis
Activation of DNA-PK is thought to trigger the activation of p53. Irradiation was shown to induce p53 activation and is known to transduce the signals to SAPK/JNK. For this purpose, phosphorylation status of stress-activated SAPK/JNK (p-SAPK/JNK) was investigated. It was observed that 6 Gy IR enhanced the expression p53 (1.25-fold), SAPK/JNK (17.06-fold) and the proapoptotic mediator, Bax (1.42-fold) (Fig. 3E and F). Result also showed that IR-caused a significant (p < 0.05) increase in the activity of caspase-3 (2.56-fold) and caspase-9 (2.74-fold), indicating complete activation of the apoptosis machinery initiated by ROS and DNA damage (Fig. 3G and H).
Immunoblot and biochemical analysis also revealed that NG inhibited the IR-induced activation of proapoptotic proteins, p53 (0.19-fold), p-SAPK/JNK (6.83-fold), Bax (0.67-fold) and activity of caspase-3 (1.62-fold) and caspase-9 (1.72-fold). On the other hand, NG-pretreatment up-regulated the expression of pro-survival protein, Bcl-2 (0.92-fold) in the irradiated condition, indicating that NG effectively protected hepatocytes from IR-induced apoptosis followed by activation of the survival pathway (Fig. 3E–H).
NG inhibited IR-induced activation of p65 (NF-κB)
The NF-κB signaling pathway has been associated with the regulation of many genes involved in the inflammatory response and production of inflammatory cytokines and proinflammatory mediators. Immunoblot analysis demonstrated that mice exposed to 6 Gy IR exhibited an enhancement of nuclear translocation of p65 (2.00-fold) and a reduction of cytosolic p65 expression (1.18-fold) over the control group. NG pretreatment markedly abrogated the IR-induced activation of p65 (1.84-fold in cytosolic fraction and 1.12-fold in nuclear fraction) in the hepatic cells compared with the control group (Fig. 4A and B). Activation of p65 was further confirmed by investigating the phosphorylation status of IκBα. It was seen that 6 Gy IR caused a reduction in the levels of hypophosphorylated IκBα and increase in hyperphosphorylated IκBα (p-IκBα) (1.53-fold) when compared to the control group. NG pretreatment significantly reduced the IR-induced phosphorylation of IκBα (1.29-fold) with respect to the IR group (Fig. 4C and D). Accumulating evidence suggested that the inhibitor of kappa B (IκB) kinase (IKK), especially IKKα/β, is the essential upstream activator of p65 (NF-κB). IKKα/β can phosphorylate the inhibitory IκBα protein (subsequent ubiquitination and degradation), resulting in the dissociation of IκBα from NF-κB. In order to further understand how the nuclear translocation of NF-κB was inhibited by NG, the expression of IKKα/β was analyzed (Fig. 4C). As shown in Fig. 4D, exposure of IR significantly increased p-IKKα/β expression (1.52-fold) in hepatic tissue as detected by immunoblot analysis. However, pretreatment with NG potently inhibited IR-induced activation of IKKα/β (0.76-fold). Only NG pretreatment did not show significant phosphorylation of IKKα/β (0.93-fold) over the control and IR group. These results suggested that NG may inhibit inflammation via suppressing the NF-κB pathway (Fig. 4).
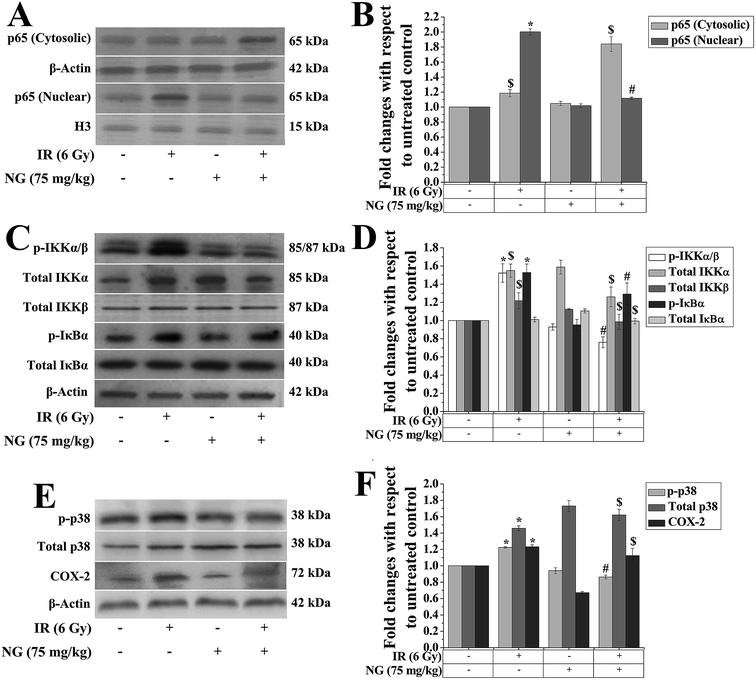 |
| Fig. 4 Assessment of IR-induced (6 Gy) NF-κB pathway activation and its prevention by the pretreatment of NG (75 mg kg−1). (A) Representative immunoblots of p65 in cytosolic and nuclear fraction of hepatic tissue. (B) Bar graph showing densitometric analysis of p65 expression in cytosolic and nuclear fraction. (C) Representative immunoblots of p-IKKα/β, total IKKα, total IKKβ, p-IκBα and total IκBα. (D) Densitometric analysis of relative protein expression of p-IKKα/β, total IKKα, total IKKβ, p-IκBα and total IκBα. (E) Representative immunoblots of p-p38, total p38 and COX-2. (F) Densitometric analysis of relative protein expression of p-p38, total p38 and COX-2 expression. β-Actin and H3 were used as internal control. Values are presented as mean ± SEM (n = 5). p < 0.05 was considered as significant. Statistical comparison: *control vs. IR (6 Gy); #IR (6 Gy) vs. NG (75 mg kg−1) + IR (6 Gy); $non significant [control vs. IR (6 Gy) and IR (6 Gy) vs. NG (75 mg kg−1) + IR (6 Gy)]. | |
NG attenuated IR-induced activation of MAPK
It is well established that the mitogen-activated protein kinase (MAPK) pathway plays a major role in IR-induced activation of NF-κB. To determine whether the inhibition of NF-κB by NG was mediated by the MAPK pathway, immunoblot analysis was done to investigate the IR-induced phosphorylation of MAPK family proteins (particularly p38-MAPK) and it's down regulation by NG pretreatment. As shown in Fig. 4E and F, IR-caused an increase in the phosphorylation of p38-MAPK (1.22-fold) in hepatic tissue, whereas pretreatment with NG significantly (p < 0.05) suppressed this phosphorylation (0.86-fold).
NG reduced IR-induced elevation of pro-inflammatory cytokines
The levels of TNF-α, IL-6 and IFN-γ in the hepatic tissue of the control and irradiated mice were shown in Fig. 5A–C. Levels of proinflammatory cytokines such as TNF-α (2.35-fold), IL-6 (4.08-fold) and IFN-γ (2.16-fold) were significantly (p < 0.05) increased in the IR-treated group in comparison with the control group. Pretreatment of irradiated mice with NG showed that the levels of these proinflammatory cytokines were significantly (p < 0.05) decreased (1.68-fold for TNF-α, 2.37-fold for IL-6 and 1.47-fold for IFN-γ) as compared with the IR group. NG pretreated group alone did not show any significant change in the levels of these proinflammatory cytokines (0.98-fold for TNF-α, 0.97-fold for IL-6 and 0.98-fold for IFN-γ) when compared to the control group (Fig. 5A–C).
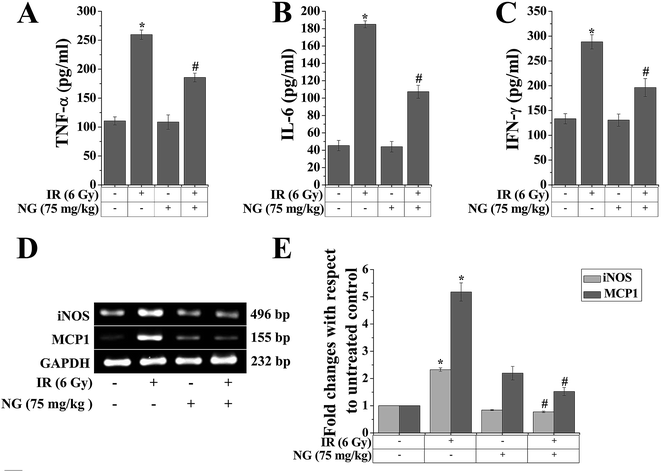 |
| Fig. 5 Assessment of IR-induced (6 Gy) hepatic inflammation and its suppression by NG (75 mg kg−1). Graph represents the level of (A) TNF-α, (B) IL-6 and (C) IFN-γ. (D) RT-PCR gel images of iNOS and MCP-1 expression. GAPDH was served as internal control. (E) Relative intensity was obtained from densitometric analysis of respective gel photographs. Values are presented as mean ± SEM (n = 5). p < 0.05 was considered as significant. Statistical comparison: *control vs. IR (6 Gy); #IR (6 Gy) vs. NG (75 mg kg−1) + IR (6 Gy). | |
As compared with the proinflammatory cytokine levels, the proinflammatory mediators, iNOS and MCP-1 gene expression was also markedly (p < 0.05) elevated in the IR-treated group (2.32-fold and 5.18-fold) with respect to the control group. However, NG considerably (p < 0.05) reduced both the iNOS and MCP-1 gene expression (0.77-fold and 1.52-fold) in the irradiated animals. Only NG pretreated group did not up-regulate these gene expression (0.84-fold and 2.20-fold) over the control and the IR group (Fig. 5D and E).
COX-2 is an inducible enzyme which plays a critical role in multiple pathophysiological processes including inflammation and tissue injury. Result showed that COX-2 expression (1.12-fold) significantly (p < 0.05) enhanced upon IR treatment (6 Gy). However, NG pretreatment decreased this level (1.12-fold) significantly (p < 0.05) when compared to the IR group. Only NG alone group did not show any significant enhancement of COX-2 expression (0.67-fold) over the IR and control group (Fig. 4E and F).
NG conferred cellular protection against IR-induced oxidative stress by Nrf2 activation
Nrf2 is anchored in the cytosol by Keap1, which is only distributed in the cytoplasm in physiological conditions. Altering Keap1 would therefore have a profound influence on Nrf2 activity. In the present study, the immunofluorescence analysis showed that the relative Keap1-FITC intensity was reduced in the NG pretreated group compared to the IR group (Fig. 6A).
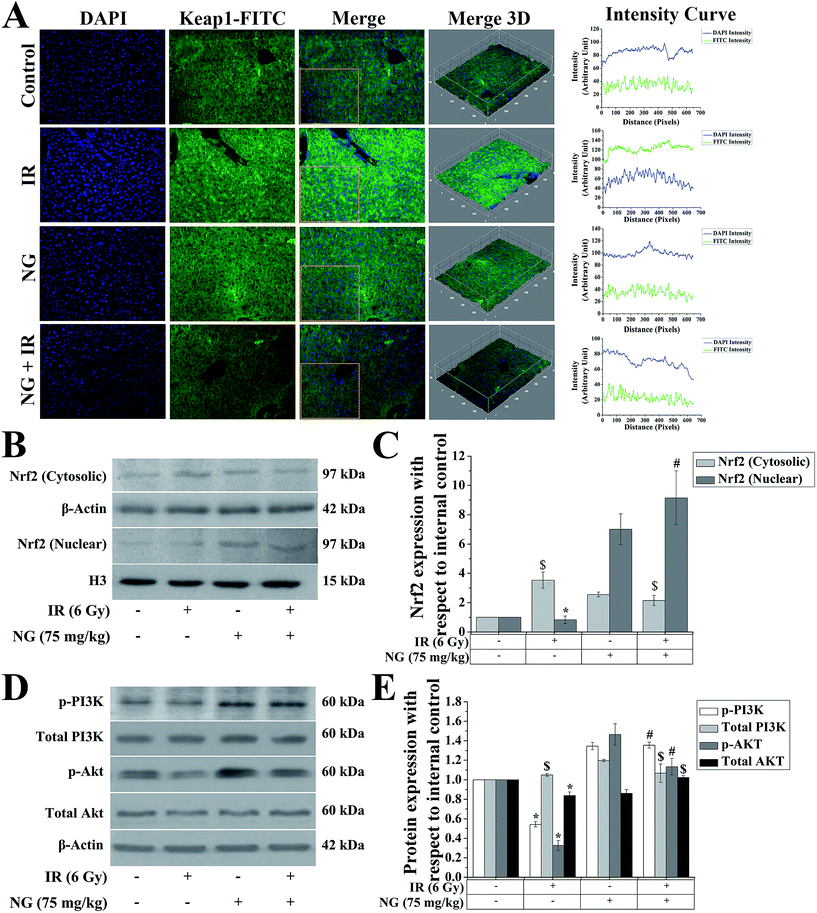 |
| Fig. 6 Assessment of Nrf2 pathway activation upon 6 Gy irradiation and NG (75 mg kg−1) pretreatment. (A) Immunofluorescence images showing qualitative expression of Keap1. Slides were viewed using fluorescence microscopy (magnification 200×). Intensity analysis of relative fluorescence of Keap1-FITC and DAPI using ImageJ software from the respective immunofluorescence micrographs. (B) Representative immunoblots of Nrf2 expression in cytosolic and nuclear fraction of hepatic tissue. (C) Graph showing densitometric analysis of Nrf2 expression in cytosolic and nuclear fraction. (D) Immunoblot analyses of relative protein expression of p-PI3K, total PI3K, p-Akt and total Akt. (E) Densitometric analysis of relative protein expression of p-PI3K, total PI3K, p-p-Akt and total Akt. β-Actin and H3 were used as internal control. Values are presented as mean ± SEM (n = 5). p < 0.05 was considered as significant. Statistical comparison: *control vs. IR (6 Gy); #IR (6 Gy) vs. NG (75 mg kg−1) + IR (6 Gy); $non significant [control vs. IR (6 Gy) and IR (6 Gy) vs. NG (75 mg kg−1) + IR (6 Gy)]. | |
To investigate whether the observed protective effect of NG was associated with a concomitant nuclear translocation of Nrf2, the appearance of Nrf2 protein in the cytoplasm and nuclei of hepatic cells following exposure of IR with or without NG treatment was examined. As shown in Fig. 6B and C, irradiated murine liver showed depletion in cytoplasmic Nrf2 (3.52-fold) and a significant decrease in nuclear Nrf2 (0.82-fold) compared to the control group. In contrast, cytoplasmic (2.15-fold) and nuclear Nrf2 (9.15-fold) levels in the NG pretreated group along with IR were significantly higher than that in the IR group.
NG activated Nrf2 through upregulation of PI3K/Akt pathway
To elucidate the upstream signaling pathway involved in NG mediated Nrf2 activation, the activation of PI3K/Akt, a major signaling molecule involved in the protection against cellular oxidative stress was assessed using immunoblot analysis. As shown in Fig. 6D and E, 6 Gy IR significantly lowered the phosphorylated form of PI3K (0.54-fold) and Akt (0.33-fold) compared to the control group. However, pretreatment with NG significantly (p < 0.05) increased in phosphorylation of PI3K (1.35-fold) and Akt (1.13-fold) in the IR-treated hepatic tissue.
NG enhanced IR-induced depletion of phase-II enzymes
Nrf2 binds to AREs and positively regulates the expression as well as induction of HO-1, NQO1, GST and Mn-SOD genes. To find out the relationship between Nrf2 and its downstream ARE-driven target antioxidative defence enzyme, the protein level of HO-1, NQO1, Mn-SOD and GST was examined through biochemical, immunoblot and flow cytometric analysis. Immunofluorescence analysis also showed similar kind of result as observed from immunoblot analysis. The relative Mn-SOD-FITC fluorescence intensity was lowered in IR-treated group. Interestingly NG pretreatment markedly augmented this intensity over the IR group (Fig. 7A).
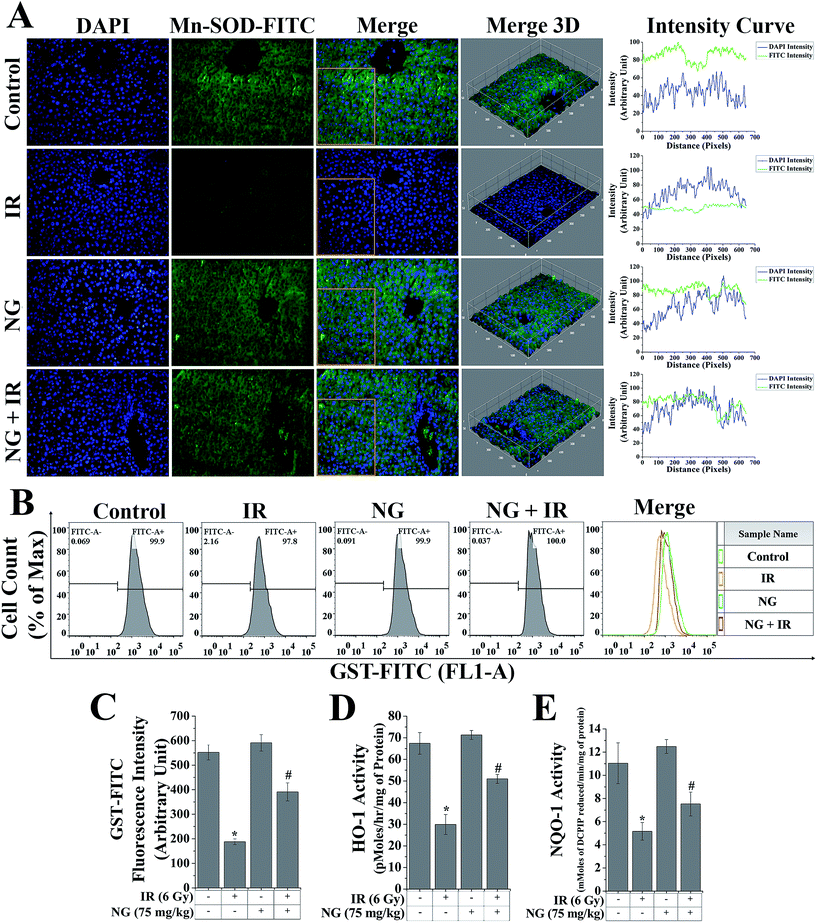 |
| Fig. 7 Assessment of NG (75 mg kg−1) and IR-induced (6 Gy) modulation of antioxidant and phase-II detoxifying enzyme system. (A) Immunofluorescence images showing qualitative expression of Mn-SOD. Slides were viewed using fluorescence microscopy (magnification 200×). Intensity analysis of relative fluorescence of Mn-SOD-FITC and DAPI using ImageJ software from the respective immunofluorescence micrographs. (B) Flow cytometric histogram showing protein expression of GST. (C) Relative fluorescence intensity of GST-FITC. Bar graph showing hepatic (D) HO-1 and (E) NQO-1 activity. Values are presented as mean ± SEM (n = 5). p < 0.05 was considered as significant. Statistical comparison: *control vs. IR (6 Gy); #IR (6 Gy) vs. NG (75 mg kg−1) + IR (6 Gy). | |
Flow cytometry analysis also confirmed that GST expression was depleted (0.34-fold) in the IR group over the control group. Furthermore, NG pretreatment was remarkably enhanced the GST expression (0.71-fold) upon irradiation compared to the IR group (Fig. 7B and C).
Fig. 7D and E showed that HO-1 and NQO-1 enzyme activity were significantly lowered (0.44-fold and 0.47-fold) in the IR group with respect to the control group. However, NG pretreatment enhanced this level by 0.76-fold and 0.68-fold compared to the IR group.
Discussion
Interaction of IR with living system causes a variety of cellular anomalies depending on the absorbed dose, duration of exposure, interval after exposure and susceptibility of tissues.3 Exposure of mammalian system to ionizing radiation can cause the development of a complex series of potentially fatal physiological and morphological changes, such as nausea, vomiting, loss of appetite, weakened immune function followed by hepatic damage.10 It imposes deleterious consequences on the living system through the creation of oxidative stress. The current observation demonstrated that irradiated mice exhibited sign of radiation sickness within 4 days after exposure to 6 Gy IR which included reduction in food, water intake and ruffling of hairs. The death of 50% of the animals exposed to 6 Gy IR within 10 days might be due to functional failure of the gastrointestinal tract. The remaining animals died within the next 20 days might be due to hematopoietic syndrome and a characteristic set of symptoms like, irritability, weight loss, emaciation, lethargy. A significant reduction in mortality was achieved when NG at the dose of 75 mg kg−1 body weight was given orally for three consecutive days before irradiation. This observation is in agreement with the data of survival analysis when mice were given several other antioxidant radioprotectors (quercetin, rutin and apigenin) along with IR.20,21
The current study was also carried out to define the nature of IR-induced hepatic damage and its reversal by pretreatment with NG. A significant increase in the activities of serum ALT, AST and LDH were found in IR-exposed animals, indicating decreased functional ability of the liver upon irradiation. The microscopic evidence also supported the degenerating hepatocytes, infiltration of immune cells.22 Several phytochemicals have shown to reduce the hepatodegenerative changes either by modulating liver toxicity marker or directly scavenges ROS.10,22 Pretreatment with NG significantly reversed this pathophysiological condition, suggesting its ability to reduce the serum toxicity marker.10
Oxidative stress is the result of a redox imbalance between the generation of ROS and the compensatory response from the endogenous antioxidant network. The results demonstrated that IR caused an increased intracellular ROS generation in the hepatocytes. This increased ROS may contribute to oxidative tissue damage as assessed by increased LPO and decreased GSH levels in the liver of IR-treated animals. These results are in accordance with reports of other investigators.23 GSH plays an important role in maintaining cellular homeostasis, and its depletion may cause oxidative injury in hepatocytes.24 NG treatment in the irradiated animals displayed an elevation in the GSH level presumably due to the antioxidant and free radical-scavenging capacities of NG. Other antioxidant enzymes like SOD and CAT were exhausted to combat excessive levels of LPO and ROS generation due to IR exposure. The adverse effects of irradiation were counteracted by NG, which proved that NG exhibited antioxidant effects not only on the nonenzymatic defence system but also the enzymatic ones.
In response to IR-induced DNA damage, the tumor suppressor p53 becomes activated as a redox-regulated transcription factor, leading to the regulation of distinct downstream pathways controlling cell cycle progression and apoptosis.4 DNA-PK, an upstream activator of p53, also activated in response to 6 Gy IR, exhibited its function in the activation of apoptosis as it constitutes one of the major sensor of IR-induced DNA damage thereby linking the DNA damage to apoptosis. The reports indicated that p53-mediated apoptosis was also regulated by mitochondrial-dependant mechanism.25 Current findings also revealed that 6 Gy IR markedly lowered the mitochondrial transmembrane potential that initiated mitochondrial-dependant apoptosis phenomenon and triggered the activation of apoptosis-associated protein expression. Thus, the result of Annexin-V-FITC/DAPI showed that irradiation significantly augmented the apoptosis and necrosis ratio over the control group, suggesting its involvement in cellular death. Increased ROS activates SAPK/JNK pathway resulting in the initiation of apoptosis in a wide range of cells by regulating the activities of pre-existing cellular Bcl-2 family proteins.25 In the present study, it was evident that IR-induced increase in intracellular ROS leads to the activation of SAPK/JNK.25 Recruitment of this ROS-dependent proapoptotic machinery caused an increased expression of the proapoptotic protein Bax.25 Results also demonstrated that a decrease in the level of Bcl-2 protein in the irradiated condition, suggesting an association of increased ratio of Bax/Bcl-2 with the leakage of outer mitochondrial membrane, which helps in releasing of cytochrome c into the cytosol thereby triggering apoptosis.26 The increased levels of activated caspase 3 and caspase 9 established the final apoptotic fate of the hepatic cells in IR-treated animals. Interestingly, the above IR-induced apoptosis phenomenon and also the indices of apoptosis were dramatically reversed with the pretreatment of NG (75 mg kg−1 body weight). NG pretreatment caused a reduction in the intracellular ROS, inhibition of mitochondrial depolarization, alteration of upstream mediators of apoptosis such as DNA-PK, p53, SAPK/JNK, reduced expression of Bax and increased expression of Bcl-2 along with the decrease in the active caspase 3 and caspase 9 which promoted survival of the hepatocytes in the irradiated condition.
In the absence of an appropriate compensatory response from the endogenous antioxidant network, the system becomes overwhelmed (redox imbalance), leading to the activation of stress-sensitive signaling pathways, such as NF-κB, and others. The redox-regulated transcription factor, NF-κB is one of the central regulators in the IR-induced inflammation.10 Several scientific reports suggested that it could be inhibited by application of various antioxidants.4 The results of the present study demonstrated that NF-κB was activated in the irradiated condition as evident from its nuclear accumulation. Activation of NF-κB was also confirmed by the increased levels of phosphorylated IκBα levels. NG treatment along with irradiation inhibited the NF-κB nuclear translocation in hepatic cells. Similarly, IKK complex, the kinase inhibitor of IκB was also hyperphosphorylated in the presence of irradiation, suggesting its direct involvement in the activation NF-κB. NG markedly decreased the IκBα degradation by inhibiting the upstream component phosphorylation leading to inhibition of NF-κB activation in the NG pretreated condition.
An up-regulation of various pro-inflammatory markers such as IL-6, TNF-α, IFN-γ, iNOS, COX-2 and MCP-1 were also found in IR-treated group which ultimately culminate into hepatic injury.10,4 In accordance with the previous scientific reports, the regulation of these pro-inflammatory markers is associated with the NF-κB activation.10 In the present study, it was also observed that IR significantly increased the NO production in hepatic tissue, suggesting that the increased level of NO may augment ROS-mediated cell death in the hepatocytes. However, NG significantly reduced these pro-inflammatory factors and NO release contributing to the reversal of the inflammatory effects of IR.
Nrf2, another important redox-regulated transcription factor, acts as a sensor for oxidative stress and regulates the activation of defensive genes against the adverse effects of oxidative stress promoting cell survival.6 An increased level of hepatic Nrf2 expression with enhanced nuclear translocation was found in mice pretreated with NG and subsequently exposed to IR. In addition, there was a significant alteration of Keap1 expression in the NG-pretreated condition suggesting positive suppression of the IR-induced activation Keap1 followed by augmenting the nuclear translocation of Nrf2. Further, PI3K/Akt which is one of the major upstream regulators of Nrf2 signaling pathway,5 was also modulated with the treatment of NG, implying the correlation between cell survival and activation of Nrf2 as PI3K/Akt signaling plays an important role in the regulation of cell fate. The protection conferred by NG against IR-induced hepatotoxicity may possibly be via increased nuclear translocation of Nrf2 and by the induction of antioxidant and phase II detoxifying enzymes. NG pretreatment also increased the activities of Nrf2-targeted antioxidant and phase 2 detoxifying enzymes namely; Mn-SOD, GST, HO-1 and NQO-1 in IR-treated mice. Induction of Mn-SOD, GST, HO-1 and NQO-1 plays an important role in conferring hepatoprotection by many phytochemicals in several models of hepatic injuries. Thus, these results suggested that IR-induced hepatic damage could be reversed by modulation of these antioxidative and phase-II detoxifying enzymes with the treatment of NG. Many phytochemicals like curcumin, epicatechin and others have been observed to confer chemopreventive activities by suppressing NF-κB and activating Nrf2 by modulating the crosstalk between these two transcription factors.26 The present study suggested that NG may confer the cytoprotective effects by modulating the cross-talk between NF-κB and Nrf2.
A considerable number of reports have shown that dietary phytochemicals can act in native form in a wide range of internal organ after oral ingestion and gets degraded after metabolic activation.27 Reports also suggested that native form as well as its metabolites (methylated and glucuronidated) played a major role in scavenging the free radicals to mitigate the oxidative stress. It also modulated the redox-regulated cellular signaling system and triggered the broad range of cellular proteins associated with hepatic damage.10,13 As the liver is the main organ of metabolism, most of the phytochemicals are metabolized in the liver and accumulated poorly in the hepatic tissue. Though, after oral ingestion, all phytochemicals primarily present in the plasma and then after metabolism, it is distributed to different organ. In our bio-distribution experiment, we found that after oral ingestion of NG, most of the native NG was found in both plasma and hepatic tissue after 6 h and then disappeared slowly after 24 h. These observations suggested that the protective action of NG may depend on either the ability of the native NG to act as a scavenger of IR-induced generation of free radicals or by interacting with cell signaling cascades either by itself or its metabolite form.
Thus, in conclusion, it can be said that IR-induced hepatic damage is mainly due to the oxidative challenge that ultimately leads to hepatic cell death. However, treatment with NG reversed the toxic effects of IR by decreasing the oxidative stress, activation of antioxidant and anti-inflammatory defence mechanisms in the liver thereby reducing apoptosis. NG also found to reduce IR-mediated DNA damage and associated with the reduction of apoptosis through the modulation of DNA-PK/p53/JNK axis. Results suggested that NG exerted hepatoprotective effects mainly by differential regulation of transcription factors Nrf2 and NF-κB (Fig. 8). These antioxidative and anti-inflammatory properties of NG make it a beneficial hepatoprotective agent and provide a strategy to protect the IR-induced deleterious systemic and cellular effects through the alteration of redox-regulated cellular signaling system.
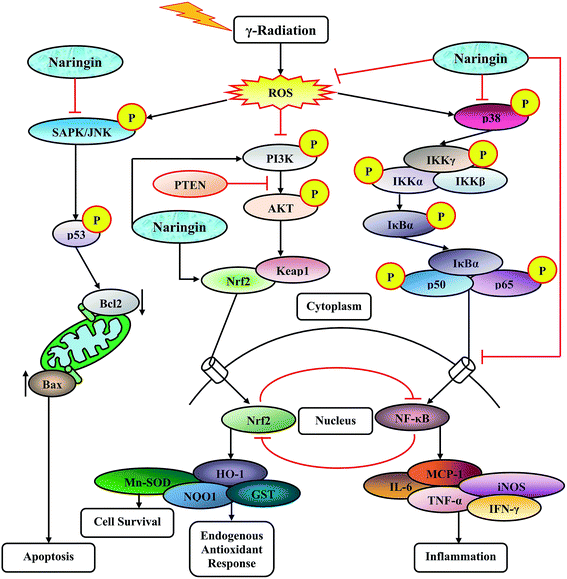 |
| Fig. 8 Schematic representation of proposed mechanism of cytoprotective action of NG on IR-induced hepatic injury with the involvement of redox-regulated cellular signaling system. | |
Acknowledgements
The authors have declared no conflict of interest. The authors are thankful to Professor M. Nandi of SINP, Kolkata for providing necessary irradiation facilities. The authors gratefully acknowledge CRNN and DBT-IPLS, University of Calcutta, for providing research facilities. The authors acknowledge the Council of Scientific and Industrial Research (CSIR) for major funding support [23(0024)/12/EMRII]. A research fellowship from CSIR-SRF, Govt. of India, to Krishnendu Manna is gratefully acknowledged.
References
- R. Baskar, K. A. Lee, R. Yeo and K. W. Yeoh, Int. J. Med. Sci., 2012, 9, 193–199 CrossRef PubMed.
- M. E. Lomax, L. K. Folkes and P. O'Neill, Clin. Oncol., 2013, 25, 578–585 CrossRef CAS PubMed.
- G. E. Adams, Br. J. Cancer, Suppl., 1987, 8, 11–18 CAS.
- K. Manna, U. Das, D. Das, S. B. Kesh, A. Khan, A. Chakraborty and S. Dey, Free Radical Res., 2015, 49, 422–439 CrossRef CAS PubMed.
- Y. R. Su, J. A. Meador, C. R. Geard and A. S. Balajee, Exp. Dermatol., 2010, 19, E16–E22 CrossRef PubMed.
- I. Buendia, P. Michalska, E. Navarro, I. Gameiro, J. Egea and R. Leon, Pharmacol. Ther., 2016, 157, 84–104 CrossRef CAS PubMed.
- Z. Y. Su, L. Shu, T. O. Khor, J. H. Lee, F. Fuentes and A. N. Kong, Top. Curr. Chem., 2013, 329, 133–162 CrossRef CAS PubMed.
- S. Bharti, N. Rani, B. Krishnamurthy and D. S. Arya, Planta Med., 2014, 80, 437–451 CrossRef CAS PubMed.
- G. C. Jagetia, V. A. Venkatesha and T. K. Reddy, Mutagenesis, 2003, 18, 337–343 CrossRef CAS PubMed.
- U. Das, K. Manna, M. Sinha, S. Datta, D. K. Das, A. Chakraborty, M. Ghosh, K. D. Saha and S. Dey, PLoS One, 2014, 9, e97599 CrossRef PubMed.
- S. B. Kesh, K. Sikder, K. Manna, D. K. Das, A. Khan, N. Das and S. Dey, Life Sci., 2013, 92, 938–949 CrossRef CAS PubMed.
- P. O. Seglen, Methods Cell Biol., 1976, 13, 29–83 CrossRef CAS PubMed.
- M. Sinha, D. K. Das, K. Manna, S. Datta, T. Ray, A. K. Sil and S. Dey, Free Radical Res., 2012, 46, 842–849 CrossRef CAS PubMed.
- K. Manna, A. Khan, D. Kr Das, S. Bandhu Kesh, U. Das, S. Ghosh, R. Sharma Dey, K. Das Saha, A. Chakraborty, S. Chattopadhyay, S. Dey and D. Chattopadhyay, J. Ethnopharmacol., 2014, 155, 132–146 CrossRef CAS PubMed.
- M. S. Carraway, A. J. Ghio, J. D. Carter and C. A. Piantadosi, Am. J. Physiol.: Lung Cell. Mol. Physiol., 2000, 278, L806–L812 CrossRef CAS PubMed.
- L. H. De Haan, A. M. Boerboom, I. M. Rietjens, D. van Capelle, A. J. De Ruijter, A. K. Jaiswal and J. M. Aarts, Biochem. Pharmacol., 2002, 64, 1597–1603 CrossRef CAS PubMed.
- J. M. Hevel, K. A. White and M. A. Marletta, J. Biol. Chem., 1991, 266, 22789–22791 CAS.
- N. Chatterjee, S. Das, D. Bose, S. Banerjee, T. Jha and K. D. Saha, Toxicol. Lett., 2015, 232, 499–512 CrossRef CAS PubMed.
- P. Chomczynski and N. Sacchi, Nat. Protoc., 2006, 1, 581–585 CrossRef CAS PubMed.
- N. Begum, N. Prasad and K. Thayalan, Int. J. Nutr., Pharmacol., Neurol. Dis., 2012, 2, 45–52 CrossRef CAS.
- S. L. Patil, H. Somashekarappa and K. Rajashekhar, Indian J. Nucl.
Med., 2012, 27, 237–242 CrossRef PubMed.
- A. Khan, K. Manna, D. K. Das, S. B. Kesh, M. Sinha, U. Das, S. Biswas, A. Sengupta, K. Sikder, S. Datta, M. Ghosh, A. Chakrabarty, A. Banerji and S. Dey, Free Radical Res., 2015, 49, 1173–1186 CrossRef CAS PubMed.
- H. Hosny Mansour and H. Farouk Hafez, Ecotoxicol. Environ. Saf., 2012, 80, 14–19 CrossRef CAS PubMed.
- S. A. Marchitti, Y. Chen, D. C. Thompson and V. Vasiliou, Eye Contact Lens, 2011, 37, 206–213 Search PubMed.
- S. Benchimol, Cell Death Differ., 2001, 8, 1049–1051 CrossRef CAS PubMed.
- S. Mukherjee, S. Ghosh, S. Choudhury, A. Adhikary, K. Manna, S. Dey, G. Sa, T. Das and S. Chattopadhyay, J. Nutr. Biochem., 2013, 24, 2040–2050 CrossRef CAS PubMed.
- S. Kumar and A. K. Pandey, Sci. World J., 2013, 2013, 16 Search PubMed.
Footnote |
† Electronic supplementary information (ESI) available. See DOI: 10.1039/c6ra01102k |
|
This journal is © The Royal Society of Chemistry 2016 |
Click here to see how this site uses Cookies. View our privacy policy here.