DOI:
10.1039/C6RA00650G
(Paper)
RSC Adv., 2016,
6, 22411-22418
Strange critical behaviors of ferromagnetic to paramagnetic transition in La0.5Ca0.5MnO3 nanowires bundles
Received
8th January 2016
, Accepted 15th February 2016
First published on 16th February 2016
Abstract
Highly ordered La0.5Ca0.5MnO3 nanowires bundles are synthesized using mesoporous SBA-15 silica as the hard template. The magnetic properties and critical behaviors of the ferromagnetic–paramagnetic (FM–PM) phase transition of the La0.5Ca0.5MnO3 nanowires bundles are investigated through isothermal magnetization methods. The calculated results show that the FM–PM transition is second order and the magnetic interaction has some deviations from the mean-field model. Though the obtained critical parameters of β = 0.596 ± 0.009, γ = 1.131 ± 0.008 and δ = 2.99 ± 0.05 follow the Widom scaling relation δ = 1 + γ/β and the single scaling equation M(H,|ε|) = εβf±(H/|ε|β+γ), the critical exponents do not obey the magnetization entropy scaling theory, indicating that the nanometer-size effect play a significant role on the magnetic phase transition and magnetic entropy for La0.5Ca0.5MnO3 nanowires bundles.
Introduction
Mixed-valent perovskite manganite compounds RE1−xAExMnO3 (RE = trivalent rare earth ions, such as La, Pr, Nd, Sm, etc. and AE = divalent alkaline earth ions, such as Sr, Ca, Ba and Pb etc.) have attracted much attention due to their extraordinary magnetic and electronic properties,1,2 as well as potential applications, such as cathodes for solid oxide fuel cells (SOFC),3,4 oxidation catalysts5 and magnetic sensors.6 Compared with transition metal groups of iron, nickel and cobalt which also have pretty large saturation magnetization,7–10 the perovskite oxides show peculiar physical properties such as colossal magnetoresistance (CMR) accompanied with FM–PM transition. A prominent feature of these materials is an insulation–metallic transition associated with ferromagnetic–paramagnetic transition. The double exchange (DE) effect in which eg electrons transfer between adjacent Mn3+ and Mn4+ ions and the Jahn–Teller effect were used to understand FM–PM transition in the manganites.1,11 In recent years, nano-structured perovskites have been investigated for their amazing chemical and physical properties such as high catalytic activities,12,13 electrochemical performance14 and unique magnetism15–19 which are different from their bulk counterparts. For nano-scale magnetic materials, the size effect becomes more significant because the magnetic disorder at surface and interface caused by defects, broken bonds, etc. can change of the magnetic properties of the system.20 In previous works, many attempts have been made to investigate magnetic nanoparticles due to the interesting size effects and surface magnetism.21,22 Several works showed that nanoscale La1−xAExMnO3 have two peculiar features: loss of charge orbital order and strengthen of FM order.15,23,24 An earlier study on La0.9Sr0.1MnO3 samples by H. Baaziz et al.25 has shown that the size reduction had strong impact on the critical behaviors. For La0.9Sr0.1MnO3 nano-particles with size of 44.98 nm, the critical exponent β (associated with spontaneous magnetization Ms) is a bit higher than the mean-field model value and γ (associated with the initial magnetic susceptibility χ0) is similar to the 3D Heisenberg model value. These phenomena were attributed to the smaller grain size and the larger surface effects. To understand the unique magnetic behavior, it is necessary to study the details of magnetic phase transition mechanism in nano-structure perovskites. For Ca2+ doped manganites, the order of ferromagnetic–paramagnetic phase transition may be related to the colossal magneto resistance (CMR) near Curie temperature (Tc).26,27 It has been reported the FM–PM phase transition for La1−xCaxMnO3 (x = 0.33) is first order due to strong electron–phonon coupling and intrinsic inhomogeneity.28 Furthermore, the FM–PM phase transition of La1−xCaxMnO3 (x = 0.4) is in good agreement with tricritical point values setting a boundary between first order (x < 0.4) and second order (x > 0.4) within the ferromagnetic range (0.2 < x < 0.5).28 For La1−xCaxMnO3 (x = 0.2), the FM–PM transition is second order corresponding to Heisenberg model describing short-range interaction.29 For single crystal La1−xCaxMnO3 (x ≈ 0.25), Belevtsev identified the FM–PM phase transition is first order.30 However, Zainullina estimated the FM–PM transition should be first order below 205 K while second order above 205 K.31 The La1−xSrxMnO3 also showed various FM–PM transition behaviors with different degrees of Sr doped.32,33 M. Hazzez et al. calculated the critical exponents of bulk La0.5Sr0.5MnO3 and showed that the transition is second-order corresponding to the mean-field model with critical exponents β = 0.507 and γ = 1.107 which dominated by long-range interaction around Tc.33 Since the FM–PM phase transition has a strong correlation with the level of divalent alkaline earth doping, it is important to focus on the FM–PM transition order of La1−xCaxMnO3 (x = 0.5). In general, half doped manganites show very interesting properties such as charge and orbital order, phase segregation and high CMR values.34,35 In the case of bulk La0.5Ca0.5MnO3, it's a paramagnetic insulator at high temperature, and becomes ferromagnetic upon cooling (Tc ∼ 225 K) and then antiferromagnetic at TN ∼ 155 K.35 The polycrystalline sample La0.5Ca0.5MnO3 exhibits double transition Tc ∼ 230 K and TN ∼ 170 K.36 However, there are few reports focusing on the FM–PM phase transition of nano-sized La0.5Ca0.5MnO3. Several methods have been reported for synthesising La0.5Ca0.5MnO3 nanomaterials, but no reports have focused on the hard template method.15,37 In this paper, we synthesized La0.5Ca0.5MnO3 nanowires bundles by hard template method using mesoporous silica SBA-15. Furthermore, the static magnetization were measured around Curie temperature (Tc) and the critical properties were investigated through isothermal magnetization methods.
Experimental details
Preparation of La0.5Ca0.5MnO3 nanowires bundles
The mesoporous silica SBA-15 was synthesized according to the procedure described in the previous reports.38 SBA-15 is a well ordered one-dimensional hexagonal silica-block copolymer mesophase and the pore size can be controlled. 5.85 g of the surfactants P123 (poly(ethyleneglycol)–poly(propylene glycol)–poly(ethylene glycol)) as the soft template was dissolved in the mixture of 94.235 g of HCl (2 mol) and deionized water with vigorously stirred for 2 h. And then a total of 13.4 ml of tetraethyl orthosilicate (TEOS, 98%, Aldrich) as the silicon source was added. After stirred for 5 min and aged at 45 °C for 24 h, the mixture was transferred into a Teflon-lined autoclave, then treated hydrothermally at 130 °C for 24 h. The product was filtered and washed with deionized water and ethyl alcohol after cooling to room temperature naturally. The final powder was calcined at 550 °C for 6 h to remove the P123.
To synthesize ordered La0.5Ca0.5MnO3 nanowires bundles, 0.0025 mol La(NO3)3·6H2O, 0.0025 mol Ca(NO3)2·4H2O, 0.01 mol 50 wt% Mn(NO3)2 solution and 0.01 mol citric acid were dissolved in the mixture of 30 ml ethanol and 10 ml distilled water with continuous stirring, followed by the addition of 2 g SBA-15. Then the mixtures were stirred at 60 °C until dry powders were obtained. The samples were then heated to 300 °C from room temperature (1 °C min−1) followed a calcination at 300 °C for 6 h to pyrolyze the precursor. The obtained materials were re-impregnated by 0.00125 mol La(NO3)3·6H2O, 0.00125 mol Ca(NO3)2·4H2O, 0.005 mol 50 wt% Mn(NO3)2 solution and 0.005 mol citric acid in the mixture of 30 ml ethanol and 10 ml distilled water at 60 °C and stirred to dry. Then the soaked samples were heated to 650 °C under the heating rate of 1 °C min−1 from room temperature and calcined at 650 °C for 6 h. To remove the template SBA-15, the obtained samples were treated in a 2 M NaOH solution at 80 °C for 0.5 h, followed by washing with distilled water and ethanol several times. The final obtained La0.5Ca0.5MnO3 nanowires bundles were copied the pore structure of the hard template SBA-15.
Characterization
Phase formation and crystallinity of the obtained La0.5Ca0.5MnO3 nanowires bundles were characterized by X-ray diffraction (XRD, DX-2700, Cu target, λ = 0.154 nm, U = 40 kV, step was 0.02°). Nitrogen physisorption experiments were measured at 77 K on a Micrometrics ASAP 2020 surface area and porosity analyzer. Specific surface areas of the powders was estimated from the relative pressure range of 0.06 to 0.2. The morphologies of the template SBA-15 and the prepared La0.5Ca0.5MnO3 nanowires bundles were observed by scanning electron microscope (SEM). The magnetic properties were studied by Superconducting Quantum Interference Device (SQUID) MPMS.
Results and discussion
The highly ordered 1D mesoporous structure in SBA-15 is observed by SEM (Fig. 1a). The diameter of the cages is measured to be about 10.8 nm from the SBA-15 SEM image, in good accordance with the pore diameter distribution graph which is inserted in Fig. 1b. Nitrogen sorption isotherm (Fig. 1b) for the SBA-15 samples shows a type IV and a large H1 hysteresis loop, indicating the typical mesoporous structure of the template.
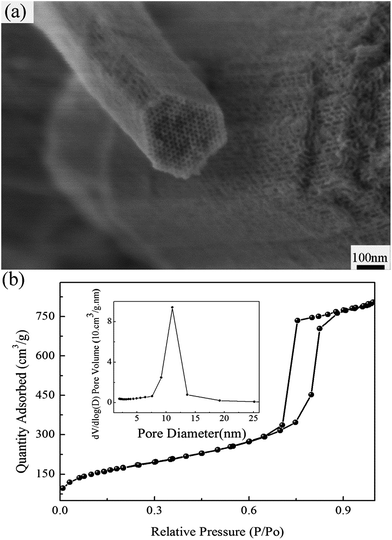 |
| Fig. 1 (a) SEM image of the hard template SBA-15. (b) Nitrogen sorption isotherms of SBA-15, the inserted picture is pore diameter distribution graph. | |
X-ray diffraction (XRD) pattern (Fig. 2) shows that the La0.5Ca0.5MnO3 nanowires bundles are single phase and can be indexed on basis of an orthorhombic cell with space group pnma.39 No peaks of impurities are observed. All the diffraction peaks are broad corresponding to the nanocrystals structure.
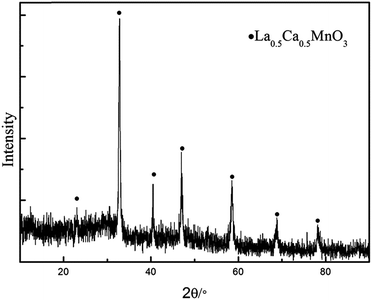 |
| Fig. 2 XRD pattern of La0.5Ca0.5MnO3 nanowires bundles templated from SBA-15. | |
The nanostructure and morphology of La0.5Ca0.5MnO3 were analyzed by scanning electron microscopy (SEM) as shown in Fig. 3a, in which the crossing section shows ordered arrangement and uniform nanowires structure, indicating the obtained samples reproduced the pore structure of SBA-15 successfully. This unique 1D nanowires bundles structure also leads to the high specific surface area 132.45 m2 g−1 (analyzed by nitrogen physisorption). Fig. 3b shows N2 adsorption–desorption isotherm of the La0.5Ca0.5MnO3 nanowires bundles and a type IV isotherm with a clear H1 type hysteretic loop is observed. It is a characteristic of mesoporous metal oxide materials, further indicating that the samples templated from molecular sieve have replicated the template pore structure successfully.
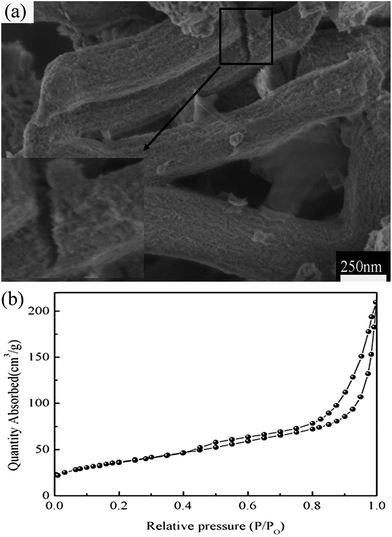 |
| Fig. 3 (a) SEM image of the prepared La0.5Ca0.5MnO3. (b) Nitrogen sorption isotherms of La0.5Ca0.5MnO3 nanowires bundles. | |
Shown in Fig. 4 is the temperature dependence of magnetization (M–T) under applied field of 100 Oe. All data were taken in the warming run after zero-field cooling (ZFC, solid circles) and field cooling (FC, open circle), respectively. An FM–PM transition occurs at 245 K, which was defined by the minimum in the dM/dT (insert in Fig. 4). A considerable divergence between the ZFC and FC curves implies the existence of surface magnetic disorders. Generally, for half doped La1−xCaxMnO3, the charge ordering state and antiferromagnetic phase are ubiquitous. However, these characteristics are not detected in this nanowires bundles system, indicating the charge ordering state maybe destroyed due to nanometer size effect. In order to deeply clarify the nanometer size effect on the magnetic phase transition, we further investigated the critical behaviors which can be described by the critical exponents.
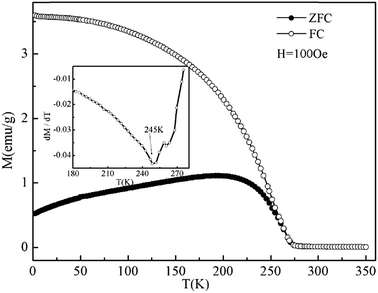 |
| Fig. 4 Temperature dependence of magnetization for La0.5Ca0.5MnO3 nanowires bundles measured at a field of 100 Oe. The insert depicts dM/dT vs. temperature curves for this material. | |
In common, the critical exponents are deduced by the fitting values obtained from the Arrott–Noakes equation of state,40 (H/M)1/γ = (T − Tc)/Tc + (M/M1)1/β where M1 refers to material constant. The Arrott plots of M1/β vs. (H/M)1/γ obtained under different temperatures should reveal a series of parallel straight lines and the line should pass through the origin when T = Tc. Generally, some different theoretical models were firstly used to construct tentative Arrott plots and then the best one was chosen to be initial Arrott plot for fitting data. In order to obtain correct values of β and γ, four different kinds of trial exponents including tricritical mean-field theory (β = 0.25, γ = 1), 3D Heisenberg model (β = 0.365, γ = 1.336), 3D Ising model (β = 0.325, γ = 1.24), and mean-field theory (β = 0.5, γ = 1), were used to make the regular Arrott plots (Fig. 5). As shown in Fig. 5d, only mean-field theory is suitable approximate for the materials. The M2 vs. H/M (β = 0.5, γ = 1) curves reveal an analogous linear behavior at T = 238 K and the line should just pass through the origin which determines the Tc approach to 238 K. However, all the lines are not completely parallel to each other and upward curvature, indicating the M2 vs. H/M with in the framework of mean-field needs to be modified.
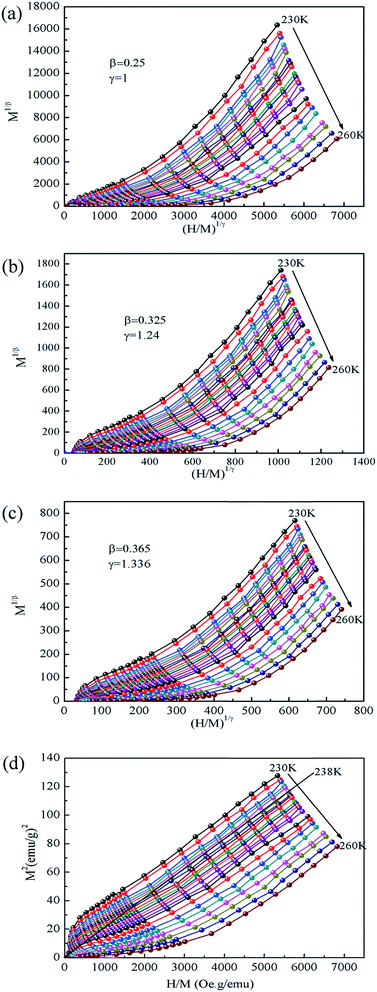 |
| Fig. 5 Arrott plot isotherms of M1/β vs. (H/M)1/γ: (a) tricritical mean-field theory (β = 0.25, γ = 1), (b) 3D Ising model (β = 0.325, γ = 1.24), (c) 3D Heisenberg model (β = 0.365, γ = 1.336), (d) mean-field theory (β = 0.5, γ = 1). | |
According to the criterion proposed by Banerjee,41 the positive slope of M2 vs. H/M lines corresponds to the second-order transition while the negative slope corresponds to the first-order transition. It is obvious that, in the present system, the positive slope of M2 vs. H/M indicates the FM to PM transition to be of the second order.
According to the scaling hypothesis,42 the critical behaviors of a magnetic system, which shows a second order magnetic phase transition near the curie point, were characterized through a series of critical exponents. The mathematical definition of the exponents from magnetization measurement can be described as
|
χ0(T)−1 = (h0/M0)εγ, ε > 0
| (2) |
|
M = DH1/δ, ε = 0, T = Tc
| (3) |
where
ε = (
T −
Tc)/
Tc is the reduced temperature,
h0,
M0 and
D are critical amplitudes. The parameters
β,
γ and
δ (associated with
Tc) are the critical exponents.
In order to obtain the accurate critical exponents, it is necessary to use some tentative exponents to construct a new Arrott plot and then fit the data of the liner part. As shown in Fig. 5d, Ms and χ0−1 can be obtained by liner extrapolation from the high-field region to the intercept. These values as functions of temperature, χ0−1 vs. T and Ms(T,0) vs. T, are plotted in Fig. 6. According to the eqn (1) and (2), the values can be fitted to two continuous curves, then the modified critical parameter β = 0.596 ± 0.009 with Tc = 238.6 ± 0.5 K, and γ = 1.131 ± 0.008 with Tc = 237.5 ± 0.6 K can be obtained.
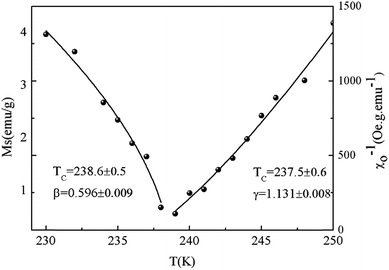 |
| Fig. 6 Temperature as a function of the spontaneous magnetization Ms (left circles) and the inverse initial susceptibility χ0−1 (right circles) along with the fitting curves based on the power laws (solid lines). | |
Fig. 7a shows the Arrott plot with modified exponents. A series of parallel lines are obtained in the high region of 1–6 T as shown in the Fig. 7b. It proves the critical exponents are suitable for the FM–PM transition in the La0.5Ca0.5MnO3 nanowires bundles and the curies temperature could be determined to be 238 K accurately.
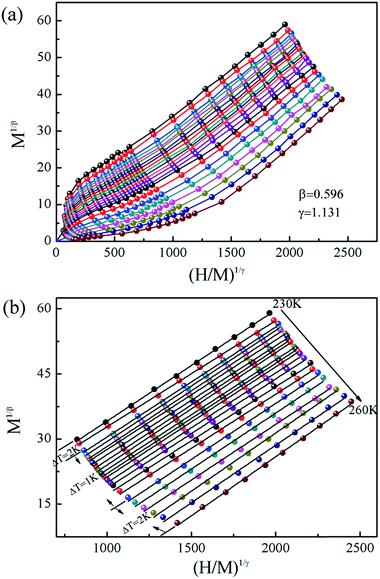 |
| Fig. 7 (a) Modified Arrott plot of M1/β vs. (H/M)1/γ with β = 0.596, γ = 1.131. (b) M1/β vs. (H/M)1/γ Arrott plot in the high field 1–6 T region and the fitting lines (solid lines). | |
According to the eqn (3), the third critical exponent δ can be determined. The isothermal magnetization at Tc = 238 K is shown in Fig. 8 and the insert picture shows the plot with ln–ln scale. The ln(M) as a function of ln(H) yield range with the slope 1/δ, then, the δ = 2.99 ± 0.05 is obtained. Besides according to the statistical theory, these critical exponents of β, γ and δ satisfy the Widom scaling relation:43
δ = 2.90 ± 0.04 was calculated from the relation. Obviously, the value of
δ = 2.99 ± 0.05 obtained from the inset of
Fig. 7 agrees well with the data calculated from the wisdom scaling relation. In the critical region, according to the prediction of the scaling equation, the magnetic equation can be written as
|
M(H,|ε|) = εβf±(H/|ε|β+γ)
| (5) |
where
f± are regular functions with
f+ for
T >
Tc and
f− for
T <
Tc. The
M(
H,
ε)
ε−β, as a function of
Hε−(β+γ), yields two universal curves for
T >
Tc and
T <
Tc, respectively. The experimental data fall on two curves as shown in
Fig. 9, which is in agreement with the scaling theory, indicating that the present exponents are reasonably accurate and unambiguous.
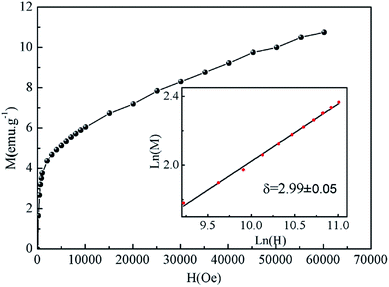 |
| Fig. 8 Isothermal M vs. H plot of La0.5Ca0.5MnO3 at Tc = 238 K; the inset shows the same plot in ln–ln scale and the solid line is the fitting straight line following eqn (3). | |
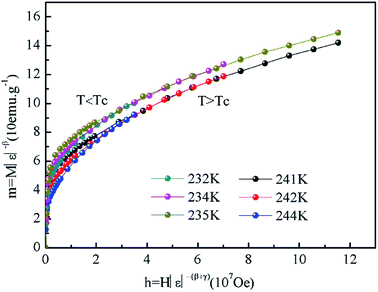 |
| Fig. 9 Scaling plots blow and above Tc using β and γ determined by modified critical exponent. | |
For the relation of the field dependence of magnetic entropy change in a magnetic system with a second-order phase transition, Oesterreicher and parker ever proposed a universal relational expression: |ΔSM| ∝ Hn, where n = 2/3,44 |ΔSM| is the maximum of magnetic entropy change around Tc.
However, due to the inappropriateness in some soft magnetic amorphous alloys with n = 2/3, Franco et al. modified the relation mentioned above and re-provided a serious of new relations named magnetocaloric effect scaling laws.45 One of the most necessary relations of the scaling laws is
|
n = 1 + (β − 1)/(β + γ)
| (6) |
here, we attempt to calculate the critical exponent of
β and
γ according to the
eqn (6) and
(4). In order to obtain the value of
n, we calculated the magnetic entropy change according to conventional method firstly.
46 Fig. 10a shows the temperature dependence of −Δ
S under different applied field changes from 1 T to 6 T. The relation of |Δ
SM|
versus H is presented in
Fig. 10b. The value of
n is determined to be
n = 1.03 ± 0.01. Based on the obtained
n and
δ, the new values of
β = 1.10 ± 0.04 and
γ = 2.2 ± 0.1 can be obtained. Thus, a new Arrott plot was redrawn with the obtained
β and
γ (not shown here). As a result, the plot shows unexpected unparallel lines, which demonstrated the unsuitable of magnetocaloric effect scaling laws on La
0.5Ca
0.5MnO
3 nanowires bundles material. Therefore, we believe that the magnetocaloric effect scaling laws have no reliability for the nano-meter system.
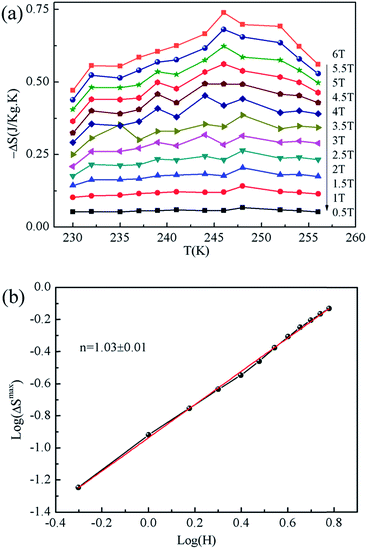 |
| Fig. 10 (a) Temperature dependence of the magnetic entropy changes −ΔS under different external magnetic fields. (b) The maximal magnetic entropy change |ΔSM| vs. H on log–log scale and the red line is the fitting curve. | |
Based on the results above, the critical exponents are similar to the mean-field model (β = 0.50, γ = 1.0 and δ = 3.0) which holds if the exchange interaction is long-range type.39,47–49 It can be observed that the critical exponents have some deviations from the mean-field theory. Physically, β describes how the ordered moment grows below Tc and γ describes the divergence of the magnetic susceptibility at Tc. In this nano-system, the obtained β = 0.596 ± 0.009 is higher than common value β, indicating that there is a slower increase of the ordered moment than usual ferromagnets, which can be interpreted by the large surface and interface in nanoscale materials providing more disordered spin magnetic moments. γ = 1.131 ± 0.008 is between the value of mean-field model and 3D Heisenberg model, indicating a higher curvature in the M(H) curve. There are two reasonable hypothesis for these unexpected critical behaviors: first one, in nanoscale materials, the magnetic influence from the disorder magnetic state is high as surface effect become more prominent in nanograins. The ordered arrangement nanowires bundles provide large surface and interface that enable the domination of disordered magnetic state of the surface sites and affects the spin-lattice coupling in manganese perovskites.50 In this situation, the decrease of particles' size leads to the inconsistent critical exponent between the actual and theoretical. The second one is the critical transition theories were developed in bulk system42 which may be not good for nano system. In addition, the magnetic entropy change shown in Fig. 10a is much lower than those in bulk materials.51 The magnetic entropy is defined by the ordering degree of magnetic moments under external magnetic field. The magnetic entropy change |ΔS| is known to increase monotonically with magnetic field strengthening. It is harder to reach magnetic moments order under external magnetic field for nanoscale materials compared with corresponding bulk materials due to the high surface disorder, which gives interpretations for the lower magnetic entropy for nano-materials. There is an unexpected phenomenon that the |ΔS| has an almost linear relation with the external field as shown in Fig. 10. The parameter n = 1.03 ± 0.01 was higher than those observed in other bulk materials.52 The higher n value found in the nanocrystalline manganites can be attributed to their sensitivity to the magnetic fields which was few mentioned in the past literatures.53
Conclusions
In summary, the La0.5Ca0.5MnO3 nanowires bundles were synthesized by hard template method. The critical properties of the perovskite manganite were studied using the isothermal magnetization around Curie point Tc. Furthermore, the reliability of the modified critical exponents were proved based on Widom scaling relation and single scaling equation. It is found the exponents have some deviations from the mean-field model and the critical parameters don't obey the magnetization entropy scaling theory in this system, which can be attributed to the surface disorders or unsuitability of the theory in nano-system. Further investigation is necessary to clarify the strong discrepancy of the reported critical exponents.
Acknowledgements
The research was funded by National Natural Science Foundation of China (51402276 and 51202235) and Foundation of Science and Technology Department of Zhejiang Province (No. 2010R50016, 2014C31125). We also thank High Magnetic Field Laboratory, Chinese Academy of Sciences, for support.
Notes and references
- E. O. Wollan and W. C. Koehler, Phys. Rev., 1955, 15, 545–563 CrossRef.
- P. G. Radaelli, Phys. Rev. B: Condens. Matter Mater. Phys., 1997, 55, 3015–3023 CrossRef CAS.
- M. J. Zhi, G. W. Zhou, Z. L. Hong, J. Wang, R. Gemmen, K. Gerdes, A. Manivannan, D. L. Ma and N. Q. Wu, Energy Environ. Sci., 2011, 4, 139–144 CAS.
- Y. M. Choi, M. C. Lin and M. L. Liu, Angew. Chem., Int. Ed., 2007, 46, 7214–7219 CrossRef CAS PubMed.
- F. Teng, W. Han, S. H. Liang, B. Gaugeu, R. L. Zong and Y. F. Zhu, J. Catal., 2007, 250, 1–11 CrossRef CAS.
- Y. Tian, D. R. Chen and X. L. Jiao, Chem. Mater., 2006, 18, 6088–6090 CrossRef CAS.
- Q. L. He, T. T. Yuan, S. Y. Wei and Z. H. Guo, J. Mater. Chem. A, 2013, 1, 13064–13075 CAS.
- Z. H. Guo, L. L. Henry, V. Palshin and E. J. Podlaha, J. Mater. Chem., 2006, 16, 1772–1777 RSC.
- X. L. Chen, S. Y. Wei, C. Gunesoglu, J. H. Zhu, C. S. Southworth, L. Y. Sun, A. B. Karki, D. P. Young and Z. Guo, Macromol. Chem. Phys., 2010, 211, 1775–1783 CrossRef CAS.
- Q. L. He, T. T. Yuan, S. Y. Wei, N. Haldolaarachchige, Z. P. Luo, D. P. Young, A. Khasanov and Z. H. Guo, Angew. Chem., Int. Ed., 2012, 51, 8842–8845 CrossRef CAS PubMed.
- C. Zener, Phys. Rev., 1951, 82, 403–405 CrossRef CAS.
- S. H. Liang, F. Teng, G. Bulgan and Y. F. Zhu, J. Phys. Chem. C, 2007, 111, 16742–16749 CAS.
- B. Seyfi, M. Baghalha and H. Kazemian, Chem. Eng. J., 2009, 148, 306–311 CrossRef CAS.
- R. Pinedo, I. R. de Larramendi, D. Ji de Aberasturi, I. G. de Muro, A. T. Aguayo and J. I. Ruiz de Larramendi, J. Mater. Chem., 2011, 21, 10273–10276 RSC.
- T. Zhang, C. G. Jin, T. Qian, X. L. Lu, J. M. Bai and X. G. Li, J. Mater. Chem., 2004, 14, 2787–2789 RSC.
- D. Zhu, H. Zhu and Y. H Zhang, J. Phys.: Condens. Matter, 2002, 14, L519–L524 CrossRef CAS.
- T. Tajiri, H. Deguchi, S. Kohiki, M. Mito, S. Takagi, M. Mitome, Y. I. Murakami and A. Kohno, J. Phys. Soc. Jpn., 2008, 77, 074715 CrossRef.
- Z. H. Wang, T. H. Ji, Y. Q. Wang, X. Chen, R. W. Li, J. W. Cai, J.-R. Sun, B. G. Shen and C. H. Yan, J. Appl. Phys., 2000, 87, 5582–5584 CrossRef CAS.
- A. Carretero-Genevrier, J. Gázquez, J. C. Idrobo, J. Oró, J. Arbiol, M. Varela, E. Ferain, J. Rodríguez-Carvajal, T. Puig, N. Mestres and X. Obradors, J. Am. Chem. Soc., 2011, 133, 4053–4061 CrossRef CAS PubMed.
- P. ękała, V. Drozd, J. F. Fagnard and P. Vanderbemden, J. Alloys Compd., 2010, 507, 350–355 CrossRef.
- J. N. Huang, Y. H. Cao, X. Zhang, Y. T. Li, J. Guo, S. Y. Wei, X. F. Peng, T. D. Shen and Z. H. Guo, AIP Adv., 2015, 5, 097183 CrossRef.
- D. L. Leslie-Pelecky, Chem. Mater., 1996, 8, 1770–1783 CrossRef CAS.
- A. Biswas, I. Das and C. Majumdar, J. Appl. Phys., 2005, 98, 124310 CrossRef.
- N. S. Bingham, P. Lampen, M. H. Phan, T. D. Hoang, H. D. Chinh, C. L. Zhang, S. W. Cheong and H. Srikanth, Phys. Rev. B: Condens. Matter Mater. Phys., 2012, 86, 064420 CrossRef.
- H. Baaziz, A. Tozri, E. Dhahri and E. K. Hlil, Solid State Commun., 2015, 208, 45–52 CrossRef CAS.
- A. P. Ramirez, J. Phys.: Condens. Matter, 1997, 9, 8171 CrossRef CAS.
- J. M. D. Coey, M. Viret and S. von Molnaír, Adv. Phys., 1999, 48, 167–293 CrossRef CAS.
- D. Kim, B. Revaz, B. L. Zink and F. Hellman, Phys. Rev. Lett., 2002, 89, 227202 CrossRef CAS PubMed.
- C. S. Hong, W. S. Kim and N. H. Hu, Phys. Rev. B: Condens. Matter Mater. Phys., 2001, 63, 092504 CrossRef.
- B. I. Belevtsev, G. A. Zvyagina, K. R. Zhekov, I. G. Kolobov, E. Y. Beliayev, A. S. Panfilov, N. N. Galtsov, A. I. Prokhvatilov and J. Fink Finowicki, Phys. Rev. B: Condens. Matter Mater. Phys., 2006, 74, 054427 CrossRef.
- R. I. Zainullina, N. G. Bebenin, V. V. Ustinov, Y. M. Mukovskii and D. A. Shulyatev, Phys. Rev. B: Condens. Matter Mater. Phys., 2007, 76, 014408 CrossRef.
- C. V. Mohan, M. Seeger, H. Kronmüller, P. Murugaraj and J. Maier, J. Magn. Magn. Mater., 1998, 183, 348–355 CrossRef CAS.
- M. Hazzez, N. Ihzaz, M. Boudard and M. Oumezzine, Phys. B, 2015, 468–469, 39–44 CrossRef CAS.
- G. Iniama, P. de la Presa, J. M. Alonso, M. Multigner, B. I. Ita, R. Cortés-Gil, M. L. Ruiz-González, A. Hernando and J. M. Gonzalez-Calbet, J. Appl. Phys., 2014, 116, 113901 CrossRef.
- P. G. Radaelli, D. E. Cox, M. Marezio, S.-W. Cheong, P. E. Schiffer and A. P. Ramirez, Phys. Rev. Lett., 1995, 75, 4488–4491 CrossRef CAS PubMed.
- A. Das, P. D. Babu, S. Chatterjee and A. K. Nigam, Phys. Rev. B: Condens. Matter Mater. Phys., 2004, 70, 224404 CrossRef.
- M. Pękała, V. Drozd, J. F. Fagnard and P. Vanderbemden, J. Alloys Compd., 2010, 507, 350–355 CrossRef.
- D. Y. Zhao, Q. S. Huo, J. L. Feng, B. F. Chmelka and G. D. Stucky, J. Am. Chem. Soc., 1998, 120, 6024–6036 CrossRef CAS.
- I. Walha, H. Ehrenberg, H. Fuess and A. Cheikhrouhou, J. Alloys Compd., 2007, 433, 63–67 CrossRef CAS.
- A. Arrott and J. E. Noakes, Phys. Rev. Lett., 1967, 19, 786–789 CrossRef CAS.
- B. K. Banerjee, Phys. Lett., 1964, 12, 16 CrossRef.
- H. E. Stanley, Introduction to Phase Transitions
and Critical Phenomena, Oxford University Press, London, 1971 Search PubMed.
- B. Widom, J. Chem. Phys., 1964, 41, 1633 CrossRef.
- H. Oesterreicher and F. T. Parker, J. Appl. Phys., 1984, 55, 4334–4338 CrossRef CAS.
- V. Franco, A. Conde, J. M. Romero-Enrique and J. SBlázquez, J. Phys.: Condens. Matter, 2008, 20, 285207 CrossRef.
- J. Y. Fan, L. S. Ling, B. Hong, L. Pi and Y. H. Zhang, J. Magn. Magn. Mater., 2009, 321, 2838–2841 CrossRef CAS.
- A. K. Pramanik and A. Banerjee, Phys. Rev. B: Condens. Matter Mater. Phys., 2009, 79, 214426 CrossRef.
- S. N. Kaul, J. Magn. Magn. Mater., 1985, 53, 5–53 CrossRef CAS.
- M. E. Fisher, S. K. Ma and B. G. Nickel, Phys. Rev. Lett., 1972, 29, 917–920 CrossRef.
- M. Kumaresavanji, C. T. Sousa, A. Pires, A. M. Pereira, A. M. L. Lopes and J. P. Araujo, Appl. Phys. Lett., 2014, 105, 083110 CrossRef.
- M. Quintero, S. Passanante, I. Irurzun, D. Goijman and G. Polla, Appl. Phys. Lett., 2014, 105, 152411 CrossRef.
- J. Y. Fan, L. Pi, L. Zhang, W. Tong, L. S Ling, B. Hong, Y. G. Shi, W. C. Zhang, D. Lu and Y. H. Zhang, Appl. Phys. Lett., 2011, 98, 072508 CrossRef.
- M. Pękała, J. Appl. Phys., 2010, 108, 113913 CrossRef.
|
This journal is © The Royal Society of Chemistry 2016 |