DOI:
10.1039/C6RA00368K
(Paper)
RSC Adv., 2016,
6, 17483-17493
BiOI hierarchical nanoflowers as novel robust peroxidase mimetics for colorimetric detection of H2O2†
Received
6th January 2016
, Accepted 1st February 2016
First published on 2nd February 2016
Abstract
In this paper, BiOI hierarchical nanoflowers (BiOI-F) were developed as a highly efficient biomimetic catalyst for the first time and used to detect H2O2. BiOI-F could be synthesized through a facile one-pot coprecipitation method, and some other BiOI samples with different morphologies were also prepared as controls. The crystal growth and morphology transition mechanism of BiOI samples was proposed, which were responsible for the distinct peroxidase-like activities. BiOI-F was demonstrated to exhibit the best intrinsic peroxidase-like activity compared to other BiOI samples due to its negative potential, large BET specific surface area, the exposed (001) facets, and the oxygen vacancies in the crystal lattice, which could efficiently catalyze the oxidation of 3,3,5,5-tetramethylbenzidine (TMB) in the presence of H2O2 to produce a blue oxide. In view of the excellent peroxidase mimetic catalytic activity of BiOI-F, a sensitive and convenient novel platform for colorimetric detection of H2O2 was successfully established, and the detection limit of H2O2 was as low as 0.05 μM. Moreover, the enhanced peroxidase-like activity of BiOI-F was proposed according to the crystal growth mechanism, surface property characterizations and active species trapping tests. In addition, the BiOI-F-based assay system displayed excellent selectivity, practicability, long-term stability, reproducibility, and reusability. This work not only develops a novel, simple and highly sensitive BiOI-F-based visual system for the detection of H2O2, but also provides some new insights into seeking for novel enzyme mimetics with enhanced catalytic activities, which have great prospects in practical applications for H2O2 detection and biomedical analysis.
1. Introduction
As classical efficient biological catalysts, natural enzymes have been widely applied in extensive fields of the chemical industry,1 biosensors,1–6 pharmaceutical processes,7,8 food processing,9 and agriculture9 due to the substrate specificity and high efficiency under mild conditions. For living organisms, natural enzymes are involved in numerous metabolic activities, and they can catalyze various useful chemical reactions. However, since most of the natural enzymes are proteins (a few are catalytic RNA molecules),3 they have some intrinsic drawbacks, including inherent instability, high costs of preparation, purification and storage, sensitivity in catalytic activity and easy denaturation in environmental conditions, which greatly restrict their practical applications.7–9 Therefore, it is interesting and challenging to develop novel and efficient artificial enzyme mimetics that can overcome certain drawbacks of natural enzymes.
Nowadays, various enzyme mimetics have been designed and developed. Among these enzyme mimetics, numbers of studies have been focused on peroxidase mimetics, including nanomaterials,4–6 cyclodextrin,10 Schiff base complex,11 and DNA complexes.12,13 More specifically, nanomaterials are attracting lots of attentions due to the large specific surface areas, more surface activation centers, and highly efficient catalytic activities,2,14,15 which greatly promote their enzyme mimetic performances. Furthermore, nanomaterials enzyme mimetics have some other advantages, such as easy preparation, low cost, structure and morphology diversity, good stability and reusability, and favored practicability under various conditions, all of which are superior to the natural enzymes.14,15 Therefore, nanomaterials enzyme mimetics are becoming a popular study point and have been used in biology, medicine, environment and other fields.2,14,15 Since Gao et al.4 reported the simulated peroxide enzyme properties of ferroferric oxide nanoparticles in 2007, many novel nanomaterials enzyme mimetics have been developed in recent years, such as graphene oxide,16,17 MoS2,18,19 MnSe,20 Fe3H9(PO4)6·6H2O,21 Fe-MIL-88NH2,22 WS2,23 FeSe–Pt@SiO2,24 Ag3PO4,25 Co–Al layered double hydroxides,6 and so on. Although the developed nanomaterials enzyme mimetics exhibit good catalytic activity and sensitivity, some disadvantages are also obvious. For example, noble/heavy metals or noble/heavy metals-containing compounds are usually used in a disposable way, which will lead to the loss of noble metals and potential environmental pollution.24–27 It is thus urgent to develop environmentally friendly enzyme mimetics with desirable catalytic activity, nice reusability and extremely good stability, which are well suited for practical applications.
Due to their low-cost, good chemical stability, excellent catalytic activity and environmentally friendly property, BiOX (X = F, Cl, Br, I) micro-nanomaterials are drawing great attentions recently, which have been used as catalysts,28–34 ferroelectric materials28,29 and pigments28,29 in various promising industrial applications. All BiOX compounds belong to the Sillen family with a typical layered tetragonal matlockite structure, characterized by [Bi2O2]2+ slabs interleaved by double slabs of halogen atoms.28,29 The strong interlayer bonding and weak interlayer van der Waals interactions lead to their unusual properties, especially the excellent catalytic activity.28,29 However, though they have good catalytic activities, the potential application of BiOX compounds in the field of biomedicine and immunoassay has not yet been fully developed. Recently, Li and coauthors35 have reported a photoactive layer-structured BiOBr as a biomimetic catalyst to detect H2O2 and glucose, which possessed a highly efficient intrinsic peroxidase-like activity and the detection limit of H2O2 could achieve as low as 0.3 μM. However, to the best of our knowledge, there is no report on the designing and developing BiOI-based biomimetic catalysts as enzyme mimics until now. As a nice light-sensitive material and visible-light-driven photocatalyst with the narrowest band gap among the BiOX compounds (about 1.7 eV), BiOI exhibits a much superior catalytic activity compared to that of BiOBr and BiOCl.32–34 Besides, various morphologies (such as microspheres, sheets, belts, and so on) and crystal structures (BiOI, Bi4O5I2, and Bi5O7I) tend to occur in BiOI,32–34,36 which will lead to diverse catalytic activities of different BiOI structures. Therefore, BiOI deserves intensive investigations towards an ideal biomimetic catalyst.
Herein, BiOI hierarchical nanoflowers were prepared via a facile one-pot coprecipitation method and developed as peroxidase mimetics to detect H2O2 for the first time. The crystal growth and morphology transition mechanism of BiOI samples was proposed, and the influence of crystal structures, morphologies and surface properties of BiOI samples on the peroxidase-like activities were investigated in detail. Among the as-prepared BiOI samples, the negatively charged BiOI-F with a large BET specific surface area exhibited the best peroxidase-like activity toward the catalytic oxidization TMB. Based on the excellent intrinsic peroxidase-like activity of BiOI-F, a novel platform with high sensitivity and selectivity for colorimetric detection of H2O2 was established. Analysis of the catalytic kinetics, catalytic mechanism, and reusability and stability of BiOI-F were also performed. Furthermore, the enhanced peroxidase-like catalytic mechanism of BiOI-F was proposed.
2. Experimental
2.1. Materials
3,3′,5,5′-Tetramethylbenzidine (TMB) was purchased from Sigma-Aldrich (Shanghai, China). Bi(NO3)3·5H2O, KI, NaOH, H2O2 (30 wt%), ethyl alcohol (EA), ethylene glycol (EG), isopropanol (IPA), p-benzoquinone (BQ), FeCl3·6H2O, CuSO4·5H2O, KNO3, NaClO, glucose, fructose, glutamate (Glu), phenylalanine (Phe), and other chemicals were all of analytical grade and were obtained from Sinopharm Chemical Reagent Co., Ltd. (China). Lircon antiseptic liquid was purchased from Shandong Lierkang Disinfection Technology Co., Ltd. (Dezhou, China). All aqueous solutions were prepared with Milli-Q water (Millipore, USA).
2.2. Preparation of BiOI nanoflowers
BiOI nanoflowers were synthesized through a facile coprecipitation method at room temperature. In a typical procedure, 10.0 mmol of Bi(NO3)3·5H2O was added into 40 mL ethylene glycol (EG) under magnetic stirring to form a transparent solution. Meanwhile, 10.0 mmol of KI was dissolved in 40 mL water under magnetic stirring to obtain a transparent solution. Then the KI solution was added dropwise into the Bi(NO3)3 solutions slowly under vigorous stirring to form a final suspension. Afterwards, the pH value of the suspension was adjusted to 7.0 by 2.0 M NaOH. Then the suspension was sequentially stirred for 24 h. Finally, the precipitates were collected and washed several times by water and absolute ethyl alcohol (EA), followed by drying at 60 °C in air for 6 h. The final products BiOI nanoflowers were obtained, which were denoted as BiOI-F.
In addition, other BiOI samples were prepared as control. By using EA as the solvent instead of EG, BiOI microspheres were synthesized in a similar way with BiOI-F, which were denoted as BiOI-S. BiOI large plates were prepared by a facile hydrothermal method. Typically, 10.0 mmol of Bi(NO3)3·5H2O was dispersed in 40 mL water mixed with 20 mL NaOH aqueous solution (0.75 M) with ultrasonic for 30 min to form a uniform suspension. Then 20 mL KI aqueous solution (30.0 mmol) was added to the above suspension under stirring. The suspension was stirred for another 60 min and then transferred into a 100 mL Teflon-lined autoclave. The autoclave was sealed, maintained at 180 °C for 24 h, and cooled to room temperature naturally. The precipitates were collected by filtration, washed with water and absolute ethyl alcohol several times, respectively, dried at 60 °C in air for 6 h. And BiOI large plates were obtained and denoted as BiOI-LP. As increasing the NaOH amount to 1.0 M and 1.5 M during the reaction, BiOI nanoplates and Bi5O7I nanobelts could be obtained, which were denoted as BiOI-NP and Bi5O7I-B, respectively.
2.3. Characterization
The crystal structure of the as-prepared products was measured by powder X-ray diffraction (XRD) measurements on a Rigaku Ultima IV powder X-ray diffractometer (Japan) under the following conditions: 40 kV, 30 mA, and graphite-filtered Cu Kα radiation (λ = 0.15406 nm). The structure and morphology of the as-prepared products were recorded by field-emission scanning electron microscopy (FESEM, Hitachi S-4800, Japan). Transmission electron microscopy (TEM) image and high resolution transmission electron microscopy (HRTEM) images, and selected area electron diffraction (SAED) pattern were acquired on a JEOL JEM-2100 field-emission transmission electron microscope (Japan) at an acceleration voltage of 200 kV, equipped with energy-dispersive X-ray spectroscopy (EDX) for elemental analysis. The zeta potential measurements were performed on a zeta potential measuring instrument (Zetasizer, Nano-ZS, Malvern Instruments Limited, UK), which was examined six times (each time being the average of 100 runs) at pH 6.0 and the mean values and standard deviations were calculated automatically based on Smoluchowski's equation. The specific surface areas of the products were measured on the basis of the Brunauer–Emmett–Teller (BET) equation with an automatic nitrogen adsorption specific surface and pore size distribution analyzer (NOVA 4000e, Quantachrome Ins., USA) at 77 K after a pretreatment at 473 K for 2 h.
2.4. Peroxidase mimetic activity assay of BiOI-F
To investigate the peroxidase mimetic activity of the as-prepared BiOI-F samples, the catalytic oxidation of the peroxidase substrate TMB in the presence of H2O2 was tested. A standard catalytic experiment was carried out at room temperature with 100 μL of 1.0 mg mL−1 BiOI-F in 300 μL of 50.0 mM phosphate buffer solution (PBS, pH = 6.0) in the presence of 500 μL of 5.0 mM H2O2, using 100 μL of 8.0 mM TMB as a substrate. Reactions were monitored in a time-scan mode at 652 nm by a UV-visible spectrophotometer (Hitachi U-2900, Japan) right after all of the reagents were added and mixed. Moreover, the effect of H2O2 concentration (0–25.0 mM), BiOI-F concentration (0–300 μg mL−1), temperature (15–50 °C), and pH (3.0–9.0) on the peroxidase mimetic activity of BiOI-F were studied under the standard conditions mentioned above to obtain the optimal conditions of BiOI-F detection system. Besides, the peroxidase mimetic properties of other BiOI samples were also carried out under the same conditions above.
Steady-state kinetic measurements were carried out in time course mode at a wavelength of 652 nm on a UV-visible spectrophotometer under the standard reaction conditions as described above by varying concentrations of TMB at a fixed concentration of H2O2 or vice versa. The Michaelis–Menten constant was calculated by using the Lineweaver–Burk double reciprocal plot with the equation 1/v = (Km/Vmax)(1/[S]) + 1/Vmax, where v is the initial reaction velocity, Km is the Michaelis–Menten constant, Vmax is the maximum reaction velocity, and [S] is the concentration of substrates.37,38
2.5. Analysis of active species
In order to inspect the radicals that play key roles in the catalytic reaction, the active species trapping experiments were conducted by adding extra 5.0 mM IPA (a quencher of ˙OH) and 5.0 mM BQ (a quencher of ˙O2−) into the reaction system with other conditions of the assay experiment fixed.34
2.6. Colorimetric detection of H2O2 using BiOI-F as peroxidase mimetics
Colorimetric detection system of H2O2 was established based on BiOI-F peroxidase mimetics. Typically, 100 μL of 8.0 mM TMB, 100 μL of 1.0 mg mL−1 BiOI-F, and 500 μL of H2O2 with different concentrations were added into 300 μL of 50.0 mM PBS (pH = 6.0). Then the mixture was recorded at 652 nm on a UV-visible spectrophotometer.
To investigate the specificity and selectivity of the BiOI-F-based visual detection system, potentially interfering metal and non-metal ions as well as common organic compounds were tested under the same standard conditions mentioned above as control, including 20.0 mM of Fe3+, Cu2+, NO3−, ClO−, glucose, fructose, phenylalanine (Phe), and tryptophan (Trp). Furthermore, the practical applicability of the BiOI-F-based visual detection system was evaluated by using commercial antiseptic liquid in place of standard H2O2 with a same assay procedure. The antiseptic liquid was diluted 1000 times before the assay experiment.
2.7. The stability and reusability of BiOI-F
The stability and reusability of BiOI-F were conducted by repeating the tests of BiOI-F-based peroxidase mimetic detection H2O2 10 cycles under the standard assay conditions mentioned above. The reaction system was recorded at 652 nm by a UV-visible spectrophotometer for 10 minutes at room temperature. After each cycle, the BiOI-F products were reused in the next cycle before collected by centrifugation, washed several times with Milli-Q water and ethyl alcohol, and dried at 60 °C for 30 min. Moreover, the crystal structure and morphology of the recycled BiOI-F products after 10 cycles were analyzed by XRD and FEEM as described in Section 2.3.
3. Results and discussion
3.1. Characterization of the as-prepared BiOI samples
The crystal structure, crystallinity and purity of the as-prepared BiOI samples were determined by XRD measurements. As shown in Fig. 1, all of the diffraction peaks in BiOI-F, BiOI-S, BiOI-LP, and BiOI-NP matched very well with the tetragonal-phase BiOI, consistent with the literature data (JCPDS no. 73-2062). Meanwhile, all diffraction peaks of Bi5O7I-B can be well indexed to orthorhombic-phase Bi5O7I (PDF 40-0548). No other impurity peaks were observed in the as-prepared BiOI samples, indicating the pure phase of these samples. However, it can be seen clearly that BiOI-S, BiOI-LP and Bi5O7I-B samples all displayed strong and sharp diffraction peaks, indicating the good crystallinity of the samples mentioned above. On the contrary, BiOI-F and BiOI-NP samples showed weak diffraction peaks and poor crystallinity. The differences in crystal structure and crystallinity of the as-prepared BiOI samples could be caused by different solvents and OH− concentrations in the preparation process. It can be seen in Fig. 1 that for BiOI-F (EG), BiOI-S (EA) and BiOI-LP (H2O) samples, the gradual narrower diffraction peaks and their higher intensity suggested the gradual larger size, which could also be evidenced by the FESEM images below. Moreover, the values of I(012)/I(110) increased when the solvent EG was substituted by EA and then replaced by water to synthesize BiOI samples. This might be ascribed to the reason that the ethyl alcohols could be preferentially adsorbed onto the (012) facets of BiOI crystals, inhibiting the preferential growth along (012) direction.32,33 Especially for BiOI-F prepared in EG solvent, the double hydroxides of EG could strongly coordinate with Bi3+ of the BiOI (012) facets, resulting in a stronger (110) diffraction peak in intensity than that of (012) diffraction peak and further leading to the value of I(012)/I(110) lower than the BiOI-S (EA, single hydroxide). Furthermore, it is remarkable that the broadening peaks of the BiOI-F could be ascribed to the small size of the building units from the hierarchical flower-like nanostructures, and implied the lattice distortion that generates more oxygen vacancies in BiOI-F,32,33 which could be ascribed to the EG-based preparation approach.32,33,35 The presence of oxygen vacancies around bismuth cations in the crystal lattice could induce the appearance of Bi(+3−x) species on the surface of BiOI, which would promote the reaction of generating oxidized radicals.
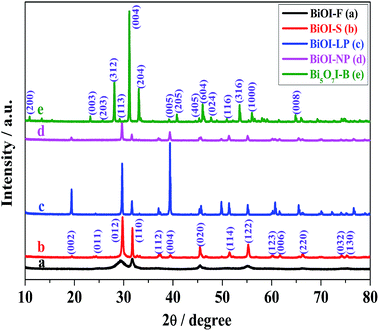 |
| Fig. 1 XRD patterns of the as-prepared BiOI samples. | |
On the other hand, for the BiOI-LP, BiOI-NP and Bi5O7I-B samples prepared in H2O with different OH− concentrations, the concentration of OH− definitively determined the crystal phase and morphology of BiOI. In the solvent H2O, (004) was the dominant crystal planes for BiOI-LP and all diffraction peaks were narrow and sharp. But as the concentration of OH− increased, the particle size remarkably decreased, accompanied by the broadening and weakening of all peaks in the diffraction pattern. Besides, the strongest diffraction peak shifted from 39.45° to 29.74°, indicating that the dominant crystal planes had changed from (004) to (102). With further increase in the amount of OH−, the plane spreading growth of BiOI was restrained and axially one-dimensional (1D) extending growth was achieved. The prepared crystal phase transformed from BiOI-LP to BiOI-NP with a smaller size, and finally to 1D Bi5O7I-B, revealing that the I− ions were replaced by OH− ions gradually. Therefore, these results demonstrated that the solvents and OH− concentration had significant effects on the crystal structure and crystallinity of BiOI samples, which might further lead to different peroxidase-like activities.
The detailed morphology and microstructure of the as-prepared BiOI samples were observed by FESEM. As shown in Fig. 2(A), the low-magnification FESEM image displays a panoramic morphology of the hierarchical flower-like BiOI-F samples with size in the range of 200–400 nm, indicating that the uniform BiOI nanoflowers could be prepared by the facile coprecipitation method with EG as solvents. The magnified FESEM image in Fig. 2(B) further reveals that the hierarchical flower-like nanostructures were self-assembled by many nanoplates with a thickness of 10 nm and a width of 100 nm. In addition, Fig. 2(C) clearly shows the FESEM image of BiOI-S prepared in EA. It can be seen that the BiOI-S samples were three-dimensional (3D) hierarchical microspheres and possessed a relatively uniform size about 3 μm, which were self-assembled by numerous interweaved nanoplates with a thickness of about 50 nm and a width of about 500 nm. Fig. 2(D) shows that the BiOI-LP samples prepared with H2O as solvents were composed of plenty of large sheets with the width of 5–10 μm and thickness of 500–800 nm. Significantly, the morphologies and sizes of the BiOI samples varied with the different solvents in preparation procedure. On the other hand, the BiOI samples prepared with different concentration of OH− present distinct morphologies, as shown in Fig. 2(E) and (F). It can be seen in Fig. 2(E) that the BiOI-NP samples prepared with more OH− revealed the irregular sheets with the size of about 2 μm and thickness of about 200 nm, which were smaller in size compared to that of BiOI-LP. With further increase in the concentration of OH−, the 1D belt-like Bi5O7I nanostructures with about 50 μm in length and 1 μm in width appeared (Fig. 2(F)), indicating that the strong alkaline condition promotes the plane spreading growth of BiOI samples to the 1D axially extending growth. Hence, it is reasonable to conclude that the morphology of BiOI (H2O) samples were dominated by the concentration of OH−, leading to the transformation from large sheets to small sheets and finally to 1D nanobelts. Moreover, different solvents were also confirmed to have a significant influence on the structure and morphology of BiOI samples.
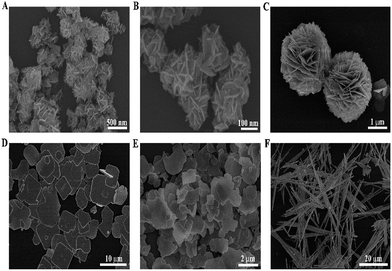 |
| Fig. 2 FESEM images of BiOI-F (A and B), BiOI-S (C), BiOI-LP (D), BiOI-NP (E), and Bi5O7I-B (F). | |
In order to further investigate the information about the intrinsic structures of the BiOI-F samples, TEM and HRTEM images and SAED patterns were measured. The typical TEM image in Fig. 3(A) shows flower-like hierarchical structures of the BiOI-F samples with a diameter of about 300 nm, which were assembled by many interlaced nanoplates. Besides, the inset of SAED pattern in Fig. 3(A) reveals a diffraction ring, which confirmed the polycrystalline structure of BiOI hierarchical nanoflowers. The HRTEM image (Fig. 3(B)) of the horizontal nanoplates, corresponding to the circled region of the flower-like structure, shows well-defined lattice fringes perpendicular to each other with the same d spaces of 0.282 nm, agreeing well with the interplanar spacing of the (110) and (−110) lattice plane of tetragonal BiOI. In addition, the inset of the HRTEM image in Fig. 3(B) shows that the vertical nanoplates had the lattice fringes with a d space of 0.913 nm, which could be indexed to the (001) lattice plane.32,33 On the other hand, H+ was the byproduct in the preparation process because of the hydrolysis of Bi(NO3)3 in the presence of water, which might be easily adsorbed on the O-terminated (001) facets, favoring the formation of BiOI nanoplates enclosed with dominant (001) facets. Therefore, these results confirmed that the bottom and top surfaces of the BiOI-F samples were identified as (001) facets, while the four lateral surfaces were (110) facets, and the nanoplates of BiOI-F samples were exposed (001) facets.32,33
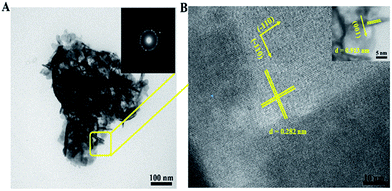 |
| Fig. 3 TEM image with the SAED image (inset) (A) and the corresponding HRTEM image (B) of BiOI-F. | |
To further investigate the chemical composition and element distribution of BiOI-F, TEM-EDX elemental mapping was examined when operating the TEM in scanning mode (STEM). Fig. 4(A) shows the EDX spectrum of BiOI-F, from which strong signals of Bi, I and O elements could be clearly observed without exhibiting other elements, confirming the coexistence of Bi, I and O elements in BiOI-F samples. The STEM mapping images in the inset of Fig. 4(A) display that the Bi, I and O elements were distributed uniformly in BiOI-F, further confirming the certain existence of the Bi, I and O elements in BiOI-F.
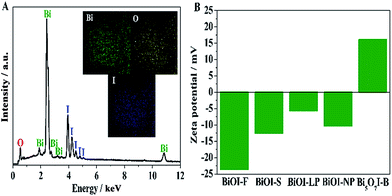 |
| Fig. 4 EDX spectrum (A) (inset: EDX elemental mapping images) of BiOI-F and zeta potentials of the as-prepared BiOI samples dispersed in ultrapure water (pH = 6.0) (B). | |
3.2. Growth mechanism of BiOI
According to the above XRD, FESEM and HRTEM characterizations, the crystal growth mechanism of the BiOI samples was proposed and shown in Scheme 1. Obviously, the solvents used in the preparation process had a significant influence on the morphologies of the obtained BiOI samples. Owing to the special chemical and physical properties, including the basicity, chelation, vapor pressure, and viscosity, EG can guide the crystal growth of BiOI.32,33 For instance, the higher viscosity will lead to the formation of thinner and smaller nanoplates since the ion diffusion rate in EG is low. In addition, on account of the effects of hydrogen bonds between hydroxyl groups, EG molecules can exist in long chains and act as a soft template,32,33 leading to the growth of BiOI nanoparticles into nanoplates and further directing the assembly of these nanoplates and formation of BiOI hierarchical structures. As illustrated in Scheme 1, when Bi(NO3)3 was dissolved in EG, bismuth alkoxides (Bi III–EG alkoxides) formed under the coordination effect, which would restrict the nucleating speed. Then these formed BiOI nanoparticles grew up and aggregate together due to the electrostatic effects, and gradually grew into 2D nanoplates to minimize their total surface energy. Subsequently, these nanoplates assembled together in an oriented attachment process and continuously grew to form a hierarchical flower-like structure (Fig. 2(B)), which is known as the Ostwald ripening process. For BiOI samples synthesized in EA, the crystal growth process is similar to that of in EG. However, considering the coordination effect between the EA and Bi3+ is weaker than that of EG, the relative high nucleating speed therefore generated more crystal nuclei at the beginning of the reaction. What's more, a relative low viscosity of EA facilitated a fast ion diffusion rate, and thus resulted in the formation of thick and large nanoplates following the anisotropic growth.32,33 Hence, a larger hierarchical microsphere (Fig. 2(C)) was finally formed through a dissolution–recrystallization process, which was also accumulated by the nanoplates with the assistance of Ostwald ripening process.
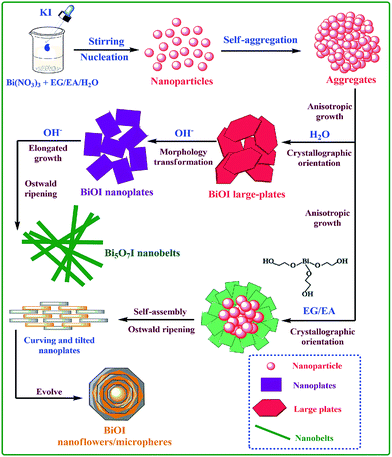 |
| Scheme 1 Schematic illustration of the growth process and the morphology transformation of BiOI samples by a different preparation route. | |
For BiOI samples synthesized in H2O, a high nucleation rate is expected to occur during the hydrothermal process, mainly benefiting from the fast ion diffusion in a low viscous solvent. When Bi(NO3)3 was added in water, BiONO3 would form in the hydrolysis process of Bi(NO3)3, along with the generation of HNO3. After the introduction of KI, BiOI nuclei appeared in a high supersaturation, which was followed by the anisotropic growth in the subsequent process via Ostwald ripening. Since BiOI has a layered structure characterized by (Bi2O2)2+ slabs interleaved by double slabs of I atoms,32,33 a sheet-like structure would be formed driven by the minimization of the total energy of the system. Finally, a smooth and large sheet structure formed by dissolution of small crystals, as seen in Fig. 2(D). Furthermore, it is a remarkable fact that H+ was the byproduct in the preparation process because of the hydrolysis of Bi(NO3)3 in the presence of water, and thus the pH value of the reaction system would also dominate the crystal structure and morphology of BiOI. In the H2O-based reaction system, OH− was used to adjust the pH value. The addition of OH− would facilitate the generation of BiONO3 by neutralizing H+ generated in the hydrolysis process of Bi(NO3)3. Hence, KI could react with the saturated BiONO3 to produce massive BiOI sheets with a large size when it was added. With the increase of OH− concentration, the nonbonding I atoms in the lattice of BiOI would be attacked by OH− and lost gradually, leading to the rupture of the large sheets and the transformation to smaller sheets (Fig. 2(E)). Furthermore, the excess OH− would restrain the plane spreading growth of BiOI structure and 1D axially extending growth was able to be achieved. Meanwhile, I− ions would be replaced by OH− ions gradually, leading to the formation of Bi5O7I nanobelts at last (Fig. 2(F)).
3.3. BET and zeta potential analysis
The BET specific surface area is an important influencing factor on the catalytic activity of catalysts, which was measured by nitrogen adsorption method.39 The BET specific surface areas were measured as 65.41 m2 g−1, 10.12 m2 g−1, 2.072 m2 g−1, 8.58 m2 g−1, and 13.64 m2 g−1 for BiOI-F, BiOI-S, BiOI-LP, BiOI-NP, and Bi5O7I-B, respectively. Evidently, BiOI-F samples exhibited a much higher BET specific surface area than that of other BiOI samples, which were in accordance with the morphologies observed by the FESEM images (Fig. 2). As is known, a larger BET specific surface area will provide more active sites and better absorption property for catalysts, promoting the catalytic activity obviously. Therefore, it is reasonable to expect that the as-prepared BiOI-F samples would present excellent peroxidase mimetic catalytic activity.
In addition, the zeta potentials of the as-prepared BiOI samples were measured at pH = 6.0. It can be seen in Fig. 4(B) that the zeta potentials were determined as −23.6 mV, −12.5 mV, −5.7 mV, −10.3 mV, and 16.1 mV for BiOI-F, BiOI-S, BiOI-LP, BiOI-NP, and Bi5O7I-B, respectively. The results clearly show that different reaction conditions combined with different solvents in preparation process leaded to different surface charge of BiOI samples, which would further give arise to different peroxidase mimetic activities.
Therefore, based on the FESEM, TEM, EDX, BET, and zeta potential analysis, it can be deduced that the solvents and OH− concentrations during the preparation process had significant influences on the morphologies and structures of BiOI, leading to different surface properties, which might further contribute to the distinct peroxidase-like activities of different BiOI samples.
3.4. Peroxidase mimetic activities of the as-prepared BiOI samples
To investigate the peroxidase mimetic activity of the as-prepared BiOI-F samples, the catalytic oxidation of the typical peroxidase substrate TMB in the absence or presence of H2O2 was tested. The UV-visible absorption spectra of the reaction systems were recorded in the range of 400–800 nm on a UV-visible spectroscopy. As seen in Fig. 5(A), the H2O2 + BiOI-F system, the TMB + BiOI-F system, and the H2O2 + TMB system all showed no absorption, while the H2O2 + TMB + BiOI-F system showed an obvious absorption peak at 652 nm, indicating that BiOI-F could act as a peroxidase to oxidize TMB and generate TMB oxide with blue color in the presence of H2O2. What's more, it can be seen in Fig. 5(B) that the different reaction systems presented apparent color variations. The H2O2 + BiOI-F system, the TMB + BiOI-F system, and the H2O2 + TMB system were almost colorless, while the H2O2 + TMB + BiOI-F system presented an obvious deep blue color, agreeing well with the UV-visible absorption spectra shown in Fig. 5(A). Therefore, these results confirmed the well peroxidase-like performance of BiOI-F, providing the feasibility of its potential application in H2O2 detection.
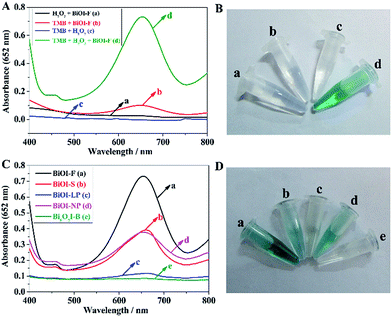 |
| Fig. 5 UV-visible absorption spectra (A) and color changes (B) of different reaction systems ((a) H2O2 + BiOI-F, (b) TMB + BiOI-F, (c) H2O2 + TMB, and (d) H2O2 + TMB + BiOI-F); UV-visible absorption spectra (C) and color changes (D) of in the presence of different BiOI samples ((a) BiOI-F, (b) BiOI-S, (c) BiOI-LP, (d) BiOI-NP, and (e) Bi5O7I-B). | |
In addition, the peroxidase mimetic catalytic activities of the as-prepared different BiOI samples were studied and compared through the catalytic oxidation of TMB in the presence of H2O2. It can be seen in Fig. 5(C) that the BiOI-F system showed the strongest absorption, followed by BiOI-S, BiOI-NP and BiOI-LP, whereas the Bi5O7I-B system showed almost no absorption, indicating the different peroxidase mimetic catalytic activities of the as-prepared BiOI samples. What's more, the color changes of TMB with H2O2 in the presence of different BiOI samples are observed in Fig. 5(D). As is shown, the BiOI-F system showed the most evident blue color compared to that of other BiOI samples, while the Bi5O7I-B system exhibited a colorless presentation, which were in well agreement with the UV-vis spectra shown in Fig. 5(C). These results indicated that the BiOI samples prepared by different methods exhibited distinct peroxidase-like properties. Among the BiOI samples, BiOI-F samples exhibited the best peroxidase mimetic catalytic performance, which might be ascribed to its larger BET specific surface area and more negative potential. The negatively charged BiOI-F would exhibit a strong affinity towards the positively charged TMB due to the two amino groups, and more TMB molecules would be absorbed on the surfaces of BiOI-F owing to the large BET specific surface area, both of which promoted the electron transfer and the reaction rate and thus enhanced the catalytic property, making BiOI-F a potential highly efficient colorimetric sensor for H2O2 detection. On the contrary, the positively charged Bi5O7I-B displayed the lowest peroxidase-like catalytic activity, which could hardly catalyze oxidation of TMB in the presence of H2O2, though Bi5O7I-B possessed a relative large BET specific surface area. Therefore, the results indicated that the surface charge and BET specific surface area jointly affect the peroxidase-like activity, and the negatively charged catalyst with a large BET specific surface area would exhibit an excellent peroxidase mimetic activity. The findings suggest the explicit strategy and direction in developing novel peroxidase mimetic catalysts.
Based on the above results and previous reports,16–25,35 a proposed peroxidase mimic mechanism for BiOI-F was illustrated in Scheme 2. The negatively charged BiOI-F with the large BET specific surface area acted as the peroxidase mimics, which could facilitate the electron transfer between TMB and H2O2 in the catalytic oxidation of TMB. During the reaction process, more positively charged TMB molecules were absorbed on the surface of BiOI-F as a chromogenic electron donor. Then the TMB molecules would donate the lone-pair electrons from the amino groups to the surface of BiOI-F, leading to the increase in electron density and mobility in BiOI-F and further accelerating the electron transfer from the BiOI-F to H2O2, thus promoted the catalytic reaction rate of TMB oxidation by H2O2.19,20,35 Subsequently, the TMB was oxidized through one electron transfer and the color variation of the system from colorless to blue was observed. Hence, the excellent peroxidase mimic catalytic activity of BiOI-F could be used as an ultrasensitive sensor in environmental and biological detection and assay.
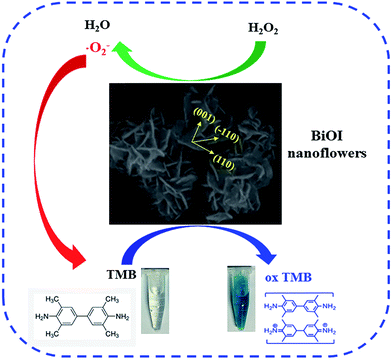 |
| Scheme 2 Schematic illustration of peroxidase mimetic activity of BiOI-F. | |
3.5. Active species study
It is known that some active radicals like
OH and
O2− could be easily generated in the H2O2 containing system.34,35 The radicals trapping process thus can be utilized to further explore the peroxidase mimic catalytic mechanism BiOI-F. Typical scavengers (IPA as
OH scavengers and BQ as
O2− scavengers) were added into the BiOI-F–H2O2–TMB system with other conditions fixed.34,35 It can be seen in Fig. 6 that an evident decrease in absorption was observed with the addition of BQ, and the color of the system showed an apparent fading and almost colorless finally, while a slight change in absorption and color was discovered in the system adding IPA. These results indicated that the strong oxidizing ˙O2− radicals generated during the reaction between BiOI-F and H2O2 played a key role in the peroxidase mimic catalytic reaction for BiOI-F, which would subsequently oxidized TMB to produce a TMB oxide with blue color. Such a process is consistent with the peroxidase-like catalytic mechanism proposed in Scheme 2. In view of the XRD results and literatures,35 it is known that the EG-based reaction system favored the BiOI with more oxygen vacancies. And in the exposed (001) facets of BiOI, the Bi–O square anti-prism would lead to many oxygen defects owing to the unstable bonds between the Bi atoms and O atoms. Simultaneously, in the crystal lattice the oxygen vacancies around Bi cations would result in the appearance of Bi(+3−x) species on the surface of BiOI, which would promote the reaction of generating oxidized
O2− radicals,35 and thus greatly enhanced the peroxidase-like activity. Moreover, the special 3d electronic structure in Bi3+ and I− further improved the catalytic activity of BiOI.
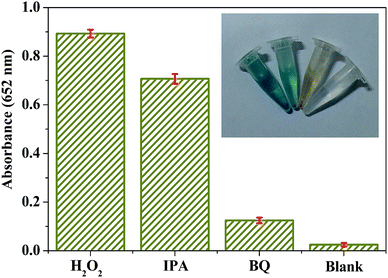 |
| Fig. 6 Time-dependent absorbance at 652 nm of 0.8 mM TMB reaction solutions in the absence or presence of scavengers (IPA and BQ) in 15 mM PBS (pH = 6.0) with 2.5 mM H2O2 and 100 μg mL−1 BiOI-F at room temperature. Inset: related color changes. | |
3.6. Optimal conditions for H2O2 detection based on BiOI-F peroxidase mimetics
To further probe the peroxidase mimetic activity of BiOI-F samples, the catalytic performance of BiOI-F under different BiOI-F concentrations and H2O2 concentrations were tested. Fig. 7(A) and (B) present that the catalytic reaction rate increased with the increase of BiOI-F concentration, and the solution color also deepened gradually. For the convenience of operation, the concentration of 100 μg mL−1 for BiOI-F was selected in the following experiments. Fig. 7(C) shows the time course-dependent absorbance changes by adding different concentrations of H2O2. It can be seen that the catalytic reaction rate increased with the increase of H2O2 concentration, and there was no inhibition in the catalytic reaction at high H2O2 concentration. Therefore, the enzyme catalytic ability of BiOI-F was better and more stable than that of HRP at high H2O2 concentration. Besides, the concentration of 2.5 mM was chosen as the optimal H2O2 concentration in the following experiments. In addition, Fig. 7(D) displays the color variations of the systems with different H2O2 concentrations, and the increase of H2O2 concentration leaded to the color variation from light to dark blue, which further demonstrated the feasibility of H2O2 detection via the BiOI-F-based colorimetric system.
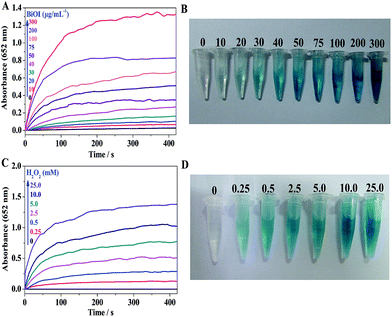 |
| Fig. 7 Time-dependent absorbance (A) and color changes (B) at 652 nm of 0.8 mM TMB reaction solutions in the absence or presence of different concentrations of BiOI-F in 15 mM PBS (pH = 6.0) with 2.5 mM H2O2 at room temperature; time-dependent absorbance (C) and color changes (D) at 652 nm of 0.8 mM TMB reaction solutions in the absence or presence of different concentrations of H2O2 in 15 mM PBS (pH = 6.0) with 100 μg mL−1 BiOI-F at room temperature. | |
In addition, the peroxidase mimetic catalytic activity of BiOI-F is closely dependent on the temperatures, pH values, and other experimental conditions as well as the natural enzymes.40,41 Hence, the influences of temperature and pH on the catalytic activity of BiOI-F were investigated. Fig. 8(A) shows a temperature-dependent assay in the range of 15 °C to 50 °C. It is seen that the catalytic activity was evidently affected by the temperature, and the catalytic activity increased first with elevating temperature and then declined when temperature was higher than 25 °C. Therefore, for the convenience of operation, 25 °C was selected as the optimal temperature. Moreover, Fig. 8(B) displays that the maximum absorption for BiOI-F system appeared at pH = 6.0, while the pH value above or lower than 6.0 would reduce the peroxidase-like activity. Thus, the optimal pH value was selected as 6.0. On the other hand, the temperature and pH influence tests indicated that the BiOI-F-based assay system could maintain a relative reasonable peroxidase-like catalytic performance under some harsh environments.
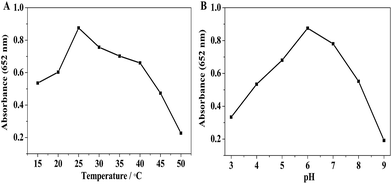 |
| Fig. 8 Dependency of peroxidase mimetic activity of BiOI-F on temperature (A) and pH (B). Experiments were carried out by using 100 μg mL−1 BiOI-F in 15 mM PBS (pH = 6.0) with 2.5 mM H2O2 and 0.8 mM TMB as substrates. | |
3.7. Steady-state kinetics assay and catalytic mechanism of BiOI-F peroxidase mimetics
The peroxidase mimetic catalytic mechanism of BiOI-F was further investigated using steady-state kinetics with H2O2 and TMB as substrates by varying the concentration of one substrate while keeping the other substrate concentration constant.18–25,35 The typical Michaelis–Menten curves were recorded over a suitable range of TMB and H2O2 concentrations, as shown in Fig. 9(A) and (B). It can be seen that the peroxidase-like catalytic reaction based on BiOI-F followed the typical Michaelis–Menten behavior18–25 towards both substrates: TMB and H2O2. Hence, a series of catalytic parameters were determined using the Michaelis–Menten model and applied to the Lineweaver–Burk double reciprocal plot (Fig. 9(C) and (D)) according to the equation:18–25,37,38 1/v = (Km/Vmax) × (1/[S]) + 1/Vmax, where v is the initial reaction velocity, Km is the Michaelis–Menten constant, Vmax is the maximum reaction velocity, and [S] is the concentration of substrates,18–25 as shown in Table S1.† Km is commonly identified as an indicator of enzyme affinity to substrates, and the smaller the value of Km, the stronger affinity between the enzyme and the substrate.14,20,35 Hence, the Km (H2O2) of BiOI-F was significantly lower than that of the natural enzyme HRP14,20,35 (Table S1†), indicating that BiOI-F had a higher binding affinity for H2O2 than HRP. On the other hand, the Km (TMB) of BiOI-F was higher than that of HRP14,20,35 (Table S1†), presenting that BiOI-F had a lower binding affinity for TMB than HRP. What's more, the activity over wide concentration ranges of TMB and H2O2 was examined to further evaluate the catalysis mechanism of BiOI-F. The double reciprocal plots of initial velocity against different concentrations of one substrate were obtained while keeping the concentration of the other substrate fixed. As shown in Fig. 9(C) and (D), the double-reciprocal plots exhibits the characteristic parallel lines of a ping-pong mechanism, indicating that BiOI-F bound and reacted with the first substrate and then released the first product before reacting with the second substrate, which is similar to that of HRP.4,18–25,35,37
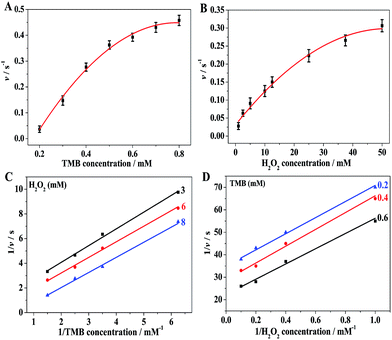 |
| Fig. 9 Steady-state kinetic assays of BiOI-F. The reaction velocity (V) was measured using 100 μg mL−1 of BiOI-F in 15 mM PBS (pH = 6.0) at room temperature. The TMB concentration was varied and the concentration of H2O2 was 10.0 mM (A), the H2O2 concentration was varied and the concentration of TMB was 0.8 mM (B), and the double-reciprocal plots of peroxidase mimetic activity of BiOI-F with a fixed concentration of one substrate relative to varying concentration of the other substrate (C and D). | |
3.8. Detection of H2O2 using BiOI-F as peroxidase mimetics
A colorimetric method for detection of H2O2 was developed on the basis of the intrinsic peroxidase-like catalytic activity of BiOI-F. Since the absorbance at 652 nm of the oxidized TMB is in proportion to the H2O2 concentration, H2O2 can be easily detected by a UV-visible spectrometer or observed by the naked eye. Fig. 10(A) displays the time-course dependent absorbance changes at 652 nm of oxidized TMB in the presence of H2O2 with different concentrations. It can be seen in Fig. 10(A) that the reaction rate of the enzymatic reaction increased with the concentration of H2O2 varied from 0.25 μM to 0.5 mM. Besides, a typical H2O2 concentration–response curve can be seen in Fig. 10(B), showing that the curve was linear in the range from 0.25 μM to 20 μM. The detection limit of H2O2 can be as low as 0.05 μM. The linear regression equation was A652 nm = 4.151 × 10−4 + 0.9781C (mM), with a correlation coefficient of 0.9969. In addition, as shown in the inset of Fig. 10(B), the color variation for H2O2 response could be obviously observed as low as 0.05 μM. Furthermore, compared to other nanomaterials with peroxidase-like activity reported previously, BiOI-F presented a reasonable linear range for H2O2 detection and the detection limit was lower than that of some reported nanomaterials,6,18,21,35,42 as shown in Table S2.† Therefore, these results indicated that the BiOI-F-based H2O2 detection system was not only a convenient approach for visual observation but also an ultrasensitive method to determine H2O2, further confirming and offering an excellent biosensing platform for the detection of H2O2.
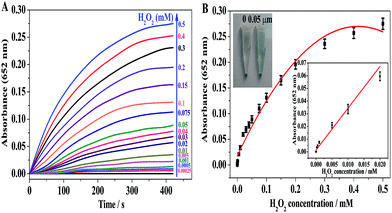 |
| Fig. 10 Time-dependent absorbance changes at 652 nm in the presence of different concentrations of H2O2 in 15 mM PBS (pH = 6.0) with 100 μg mL−1 BiOI-F at room temperature (A) and a dose–response curve for H2O2 detection (B). Inset: the linear calibration plot for H2O2 and color changes. | |
3.9. Selectivity and real sample assay for BiOI-F peroxidase mimetics
In reveal samples, there are often organic and/or inorganic substances, which may or may not interfere with the analysis of H2O2 with the use of the BiOI-F-based H2O2 detection system. Consequently, several potentially interfering metal and non-metal ions as well as common organic compounds were tested to estimate the selectivity and practical applicability of the proposed BiOI-F-based optical H2O2 detection system, including 20.0 mM of Fe3+, Cu2+, NO3−, ClO−, glucose, fructose, Phe, and Trp. Besides, the commercial antiseptic liquid diluted 1000 times was selected as the real sample (RS) and tested with the procedure similar to the above experiments. It can be seen in Fig. 11 that though the concentrations of other substances were 8 times higher than that of the standard H2O2 (2.5 mM), no obvious absorbance at 652 nm and color change was measured and observed, indicating that there was few interference effect in the presence of these interferents. In addition, the diluted commercial antiseptic liquid showed an obvious absorbance at 652 nm, and the color variation could be distinguished distinctively by the naked eye compared to other substances and standard H2O2. And the concentration of H2O2 in the commercial antiseptic liquid was calculated to be about 0.83 M in the light of the absorbance and the calibration curve. Therefore, these results indicated that the BiOI-F-based assay system was highly selective for H2O2 detection and favored an application in practical detection and analysis.
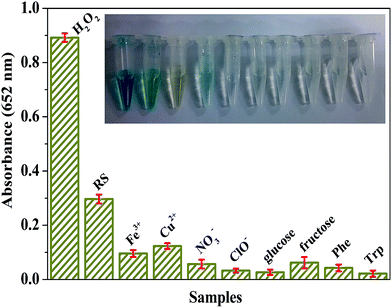 |
| Fig. 11 Selectivity and reveal sample (antiseptic liquid was diluted 1000 times) analysis for BiOI-F detection system with interfering substances by monitoring the absorbance at 652 nm, including 20.0 mM of Fe3+, Cu2+, NO3−, ClO−, glucose, fructose, Phe, and Trp. Inset: the related color changes. | |
3.10. Stability and reusability of BiOI-F as peroxidase mimetics
The stability and reusability of the BiOI-F-based assay system were probed by conducting the catalytic reaction successively for ten cycles. After each cycle, the BiOI-F samples were collected by centrifugation, washed several times with Milli-Q water and ethyl alcohol, and dried. Then the BiOI-F samples were reused in the next reaction cycle. It can be seen in Fig. 12(A) that there was no obvious loss in the absorbance values at 652 nm of the reaction system during the ten successive cycles with every cycles lasting for 10 min, revealing no loss of peroxidase mimic activity during ten successive cycles. Besides, the relative standard deviation (RSD) of the absorbance values was only 1.81%, presenting the excellent reproducibility, stability and reusability of the BiOI-F-based assay system. Moreover, the nearly same color change in each reaction system further confirmed its good reproducibility even reused after 10 cycles. Furthermore, the change in crystal structure and morphology of BiOI-F samples after ten successive reactions were characterized by XRD and FESEM. It can be seen in Fig. 12(C) that the XRD pattern of BiOI-F after ten successive cycles showed no significant changes in both peak intensity and position, implying a stable crystal structure. The FESEM image in Fig. 12(D) displays that there was no distinctive variation in morphology of BiOI-F, though a little impurity appeared on the surface of the nanoflowers, revealing its good stability. These results indicated that BiOI-F samples exhibited an excellent stability, reusability and reproducibility during the peroxidase mimic catalytic reaction, which guaranteed a long-term and practical use in various environment for analysis and detection.
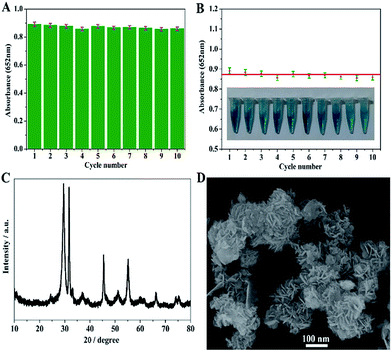 |
| Fig. 12 Stability and reusability experiments of BiOI-F detection system conducted at 100 μg mL−1 BiOI-F in 15 mM PBS (pH = 6.0) with 2.5 mM H2O2 and 0.8 mM TMB as substrates (A and B); XRD pattern (C) and FESEM image of BiOI-F after ten cycles (D). Inset: the related color changes in ten cycles. | |
4. Conclusions
In conclusion, BiOI hierarchical nanoflowers were prepared via a facile one-pot coprecipitation method and developed as peroxidase mimics to detect H2O2. BiOI-F could be synthesized through a facile one-pot coprecipitation method, and some other BiOI samples with different morphologies were also prepared as control. The crystal growth and morphology transition mechanism of BiOI samples was proposed, which was responsible for the distinct peroxidase-like activities. The BiOI-F was demonstrated to exhibit the best intrinsic peroxidase-like activity comparing to other BiOI samples due to its negative potential, large BET specific surface area, the exposed (001) facets, and the oxygen vacancies in the crystal lattice. The detection limit of H2O2 via BiOI was low to 0.05 μM, realizing the simple and ultrasensitive detection of H2O2. The ˙O2− radicals were proved to play major roles in the peroxidase-like catalytic reaction for BiOI-F-based assay system. In addition, BiOI-F peroxidase mimics showed a good selectivity, practicability, stability, reproducibility, and reusability, making it a promising prospect in practical detection of H2O2 and biomedical analysis. This work not only develops a novel, fast response, low cost, accessible and highly sensitive system for visual detection of H2O2, but also provides some new insights into seeking for novel enzyme mimetics with enhanced catalytic activities.
Acknowledgements
This work was supported by “Top Hundred Talents Program” of Chinese Academy of Sciences (CAS) and National Natural Science Foundation of China (Grant No. 41476068).
Notes and references
- Y. J. Song, W. L. Wei and X. G. Qu, Adv. Mater., 2011, 23, 4215–4236 CrossRef CAS PubMed.
- H. Wei and E. K. Wang, Chem. Soc. Rev., 2013, 42, 6060–6093 RSC.
- B. M. Nestl, S. C. Hammer, B. A. Nebel and B. Hauer, Angew. Chem., Int. Ed., 2014, 53, 3070–3095 CrossRef CAS PubMed.
- L. Gao, J. Zhuang, L. Nie, J. Zhang, Y. Zhang, N. Gu, T. Wang, J. Feng, D. Yang, S. Perrett and X. Yan, Nat. Nanotechnol., 2007, 2, 577–583 CrossRef CAS PubMed.
- R. André, F. Natálio, M. Humanes, J. Leppin, K. Heinze, R. Wever, H. C. Schröder, W. E. G. Müller and W. Tremel, Adv. Funct. Mater., 2011, 21, 501–509 CrossRef.
- L. J. Chen, B. Sun, X. Wang, F. M. Qiao and S. Y. Ai, J. Mater. Chem. B, 2013, 1, 2268–2274 RSC.
- J. M. Woodley, Trends Biotechnol., 2008, 26, 321–327 CrossRef CAS PubMed.
- J. Tao and J. Xu, Curr. Opin. Chem. Biol., 2009, 13, 43–50 CrossRef CAS PubMed.
- J. Barber, Chem. Soc. Rev., 2009, 38, 185–196 RSC.
- Z. Yang and H. Ji, ACS Sustainable Chem. Eng., 2013, 1, 1172–1179 CrossRef CAS.
- A. Khorshid, R. R. Amin and Y. M. Issa, J. Chem. Acta, 2013, 1, 52–58 Search PubMed.
- X. Chen, X. Zhou and J. Hu, Anal. Methods, 2012, 4, 2183–2187 RSC.
- L. J. Chen, K. Sun, P. Li, X. Z. Fan, J. C. Sun and S. Y. Ai, Nanoscale, 2013, 5, 10982–10988 RSC.
- Y. Lin, J. Ren and X. Qu, Acc. Chem. Res., 2014, 47, 1097–1105 CrossRef CAS PubMed.
- Q. Wang, Z. Yang, X. Zhang, X. Xiao, C. K. Chang and B. Xu, Angew. Chem., Int. Ed., 2007, 46, 4285–4289 CrossRef CAS PubMed.
- Y. Song, K. Qu, C. Zhao, J. Ren and X. Qu, Adv. Mater., 2010, 22, 2206–2210 CrossRef CAS PubMed.
- M. Liu, H. Zhao, S. Chen, H. Yu and X. Quan, ACS Nano, 2012, 6, 3142–3151 CrossRef CAS PubMed.
- T. Lin, L. Zhong, L. Guo, F. Fu and G. Chen, Nanoscale, 2014, 6, 11856–11862 RSC.
- K. Zhao, W. Gu, S. Zheng, C. Zhang and Y. Xian, Talanta, 2015, 141, 47–52 CrossRef CAS PubMed.
- F. M. Qiao, L. J. Chen, X. Li, L. Li and S. Y. Ai, Sens. Actuators, B, 2014, 193, 255–262 CrossRef CAS.
- T. Zhang, Y. Lu and G. Luo, ACS Appl. Mater. Interfaces, 2014, 6, 14433–14438 CAS.
- Y. L. Liu, X. J. Zhao, X. X. Yang and Y. F. Li, Analyst, 2013, 138, 4526–4531 RSC.
- Q. Chen, J. Chen, C. J. Gao, M. L. Zhang, J. Y. Chen and H. D. Qiu, Analyst, 2015, 140, 2857–2863 RSC.
- F. M. Qiao, Z. Wang, K. Xu and S. Y. Ai, Analyst, 2015, 140, 6684–6691 RSC.
- Z. B. Xiang, Y. Wang, P. Ju and D. Zhang, Microchim. Acta, 2016, 183, 457–463 CrossRef CAS.
- K. Saha, S. S. Agasti, C. Kim, X. Li and V. M. Rotello, Chem. Rev., 2012, 112, 2739–2779 CrossRef CAS PubMed.
- W. J. Shi, H. Fan, S. Y. Ai and L. Zhu, RSC Adv., 2015, 5, 32183–32190 RSC.
- X. Zhang, Z. H. Ai, F. L. Jia and L. Z. Zhang, J. Phys. Chem. C, 2008, 112, 747–753 CAS.
- H. F. Cheng, B. B. Huang and Y. Dai, Nanoscale, 2014, 6, 2009–2026 RSC.
- J. Jiang, K. Zhao, X. Y. Xiao and L. Z. Zhang, J. Am. Chem. Soc., 2012, 134, 4473–4476 CrossRef CAS PubMed.
- H. J. Zhang, Y. X. Yang, Z. Zhou, Y. P. Zhao and L. Liu, J. Phys. Chem. C, 2014, 118, 14662–14669 CAS.
- K. X. Ren, K. Zhang, J. Liu, H. D. Luo, Y. B. Huang and X. B. Yu, CrystEngComm, 2012, 14, 4384–4390 RSC.
- J. Hu, S. X. Weng, Z. Y. Zheng, Z. X. Pei, M. L. Huang and P. Liu, J. Hazard. Mater., 2014, 264, 293–302 CrossRef CAS PubMed.
- L. Ye, J. Chen, L. Tian, J. Liu, T. Peng, K. Deng and L. Zan, Appl. Catal., B, 2013, 130–131, 1–7 CAS.
- L. Li, L. Ai, C. Zhang and J. Jiang, Nanoscale, 2014, 6, 4627–4634 RSC.
- S. M. Sun, W. Z. Wang, L. Zhang, L. Zhou, W. Z. Yin and M. Shang, Environ. Sci. Technol., 2009, 43, 2005–2010 CrossRef CAS PubMed.
- D. J. Porter and H. J. Bright, J. Biol. Chem., 1983, 258, 9913–9924 CAS.
- L. Ai, L. Li, C. Zhang, J. Fu and J. Jiang, Chem.–Eur. J., 2013, 19, 15105–15108 CrossRef CAS PubMed.
- L. Zhang, W. Wang, Z. Chen, L. Zhou, H. Xu and W. Zhu, J. Mater. Chem., 2007, 17, 2526–2532 RSC.
- P. D. Josephy, T. Eling and R. P. Mason, J. Biol. Chem., 1982, 257, 3669–3675 CAS.
- K. Chattopadhyay and S. Mazumdar, Biochemistry, 2000, 39, 263–270 CrossRef CAS PubMed.
- H. Wei and E. Wang, Anal. Chem., 2008, 80, 2250–2254 CrossRef CAS PubMed.
Footnote |
† Electronic supplementary information (ESI) available. See DOI: 10.1039/c6ra00368k |
|
This journal is © The Royal Society of Chemistry 2016 |