DOI:
10.1039/C6RA00172F
(Paper)
RSC Adv., 2016,
6, 19707-19715
Design and evaluation of multifunctional antibacterial ion-doped β-dicalcium silicate cements favorable for root canal sealing
Received
4th January 2016
, Accepted 4th February 2016
First published on 12th February 2016
Abstract
A root canal sealer plays some important roles in accomplishing various functions including antibacteria and anti-microleakage. Meanwhile, such a sealer is also expected to readily induce apatite mineralization in damaged periapical tissues and reconstruct the surrounding alveolar bone. Taking the potent antibacterial ability of some biologically essential trace elements into account, we explore the effects of Zn or Cu doping in β-dicalcium silicate (β-C2Si) on the physicochemical modification and biological functions of its self-curing cement and compare with the β-C2Si cement free of foreign ion doping. An interesting aspect of the Zn or Cu doping in β-C2Si was the prolongation of setting time and the decrease of mechanical strength, but retardation in the degradation and improvement of anti-microleakage. Furthermore, the β-C2Si doped with 10% Cu exhibited more excellent antibacterial properties against P. gingivalis and E. faecalis. Additionally, there was similar apatite formation ability and cell growth on the β-C2Si cements with and without Zn-/Cu-doping within the initial 1–5 d. Totally, it is demonstrated that the physicochemical and biological performances are favorably altered with Zn or Cu doping in β-C2Si with a consequent effect on setting time, chemical stability (ion release, degradation), anti-microleakage, and antibacterial activity. Therefore, it is indicated that the Zn or Cu-doped β-C2Si is promising as a multifunctional root canal sealer.
1. Introduction
The ideal outcome of endodontic therapy may depend on the thorough elimination of the microbial source,1 complete sealing of the root canal2 and regeneration of the damaged periapical tissues and surrounding alveolar bone.3 In this context, a suitable root canal sealer should have good integration between materials and the dentinal wall, and also it should have excellent bioactivity to promote bone tissue regeneration. Moreover, it is expected to have permanent antibacterial properties to inhibit bacterial growth within the root canal and periapical tissue.4 In this regard, an ideal endodontic sealer may possess dimensional and chemical stability and excellent antimicrobial property. Nowadays, a variety of root canal sealers such as zinc oxide–eugenol, polydimethylsiloxane, epoxy resin and methacrylate resin have been used clinically. Unfortunately, these materials show varying degree of cytotoxicity, and even cause some acute inflammatory infiltrates in short time.5
Recently, a variety of Ca-silicate materials have attracted great attention in endodontic treatment because of their excellent bioactivity and good biocompatibility in vitro and in vivo.6–9 These characteristics of Ca-silicate materials are mainly related to the release of calcium ions and silicate groups in the appliance areas. It has been claimed that calcium ion is able to induce the deposition of hard tissue such as dentin and alveolar bone, and moreover it can cause elevation of pH value with antibacterial activities and accelerate healing process.10 Sun et al. reported that the released silicate groups from dicalcium silicate coatings exhibited excellent potential to induce apatite formation in vitro and in vivo, and furthermore to activate bone-related gene expression and stimulate cell proliferation.11 Mineral trioxide aggregate (MTA), a mixture of Ca(-Al) silicate compounds and bismuth oxide, has been used as root-end filling, root canal sealer, apexification, root-perforation repair, pulp capping materials in endodontics.12 However, the recent studies showed that the long setting time, potential of discoloration, severe cytotoxicity for long time, and genotoxic Al element that might destroy calcium homeostasis and cause cellular oxidation, have stimulated the development of alternative materials.9,13,14
β-Dicalcium silicate (β-C2Si), as one of critical components in Portland cement and promising implant for bone defect repair, has received significant attention in endodontic treatment owing to its excellent bioactivity, biocompatibility, and self-setting ability compared to conventional endodontic materials.4,15,16 When reacted with water at room temperature, β-C2Si is known to form calcium silicate hydrate gel (C–S–H) which contribute to the development of mechanical strength and the self-setting properties. Our previous studies have showed that biomimetic apatite can be deposited on the β-C2Si particles within 24 hours and osteogenic cells also establish close contacts with its ceramic after 24 hour culture.15,17 However, the pure β-C2Si cement showed slow hydraulicity, and its low antibacterial property particularly at the early stage would potentially affect its clinical efficacy in endodontic treatment.17
More recently, two attempts have been made to improve the properties of β-C2Si-based cements. Wu et al. incorporated Bi2O3 into the β-C2Si cements to improve the cytotoxicity and antimicrobial activity of the composite.9 Our previous attempts have found that CaO-rich β-C2Si powders could be used to develop the antibacteria-improved cements potentially favorable for root canal filling.4 On the other hand, the potential of some metallic cations to act as antibacterial agents in the context of oral infection and wound healing has been widely studied as an alternative to traditional antibiotic treatments which may be followed by bacterial resistance.18,19 For instance, zinc and copper, as essential mineral elements in human body, are widely reported to have antimicrobial effect on inflammation and infection.20 Zinc has also been proved to have a stimulatory effect on bone formation and mineralization. In clinic, bone growth retardation and defects are common findings in humans with zinc deficiency.21 Copper is known to be closely associated with the angiogenesis and shows stimulatory effect on endothelial cells proliferation at a specific dose level and wound healing by up-regulation of vascular endothelial growth factor (VEGF).22,23 In this regard, Ca-silicate biomaterials are valuable resources as they can be doped with some foreign metal ions to provide a smart strategy for the controlled delivery of therapeutic ions in situ, which would be favorable for promoting osteogenesis, and especially for endodontic treatment as root canal filler.
In this feasibility study, we employed a sol–gel reaction to prepare the β-C2Si powders doped with Zn or Cu, and a particular emphasis was given to the behaviour of Zn or Cu doping on the physicochemical and biological performances of β-C2Si-derived cements, with the aim of testing their potential as root canal sealer. In particular, their cell activity in vitro, antibacterial and anti-microleakage properties were compared with the pure β-C2Si cement. Moreover, in order to evaluate the effect of the foreign ion doping on the self-setting and bioactivity of the cements, the setting time and immersion in simulated body fluid (SBF) have been measured.
2. Materials and methods
2.1 Preparation of paste
Colloidal SiO2 and reagent-grade calcium nitrate tetrahydrate, zinc nitrate tetrahydrate, and copper nitrate hexahydrate (Shanghai Sinopharm Reagent Co.) were used directly. The β-C2Si powders with different Zn- or Cu-substituting-Ca ratio (0, 5, 10 mol%) were prepared by a sol–gel process.15 For easier abbreviation, we therefore defined the products with different Zn- or Cu-substituting-Ca ratio (x) as C2Si–xZn or C2Si–xCu (x = 0, 5, 10; Table 1). Briefly, the chemicals were mixed under vigorous stirring for 6 h at 60 °C, and aged at room temperature overnight. The obtained wet gel was further dried at 120 °C for 48 h. The dry gel was calcined at 800 °C for 3 h. The powders were ground for 6 h using a planetary ball mill in ethanol medium and then dried at 60 °C. After that, the C2Si–xZn or C2Si–xCu powders were hand-mixed with deionized water in a liquid-to-power (l/p) ratio of 0.5 ml g−1 and transferred to stainless-steel mold with diameter of 6 mm, then stored in a 37 °C, 100% humidity waterbath. After the paste was set for 1 d, the structure of the C2Si–xZn or C2Si–xCu cements were observed by scanning electron microscopy (SEM; JEM-6700F) and the phase composition was characterized by X-ray diffraction (XRD; Rigaku). A Zetasizer Nano S90 (Malvern Instruments, UK) was used to measure the particle size of the C2Si powders with Zn or Cu doping.
Table 1 The molar ratio in the reactants and chemical compositions in Zn-/Cu-doped C2Si powders
Samples |
Molar ratio in reactants |
Content in powder (%) |
Ca |
Zn |
Cu |
Si |
Zn |
Cu |
β-C2Si |
2.00 |
0 |
0 |
1.00 |
0 |
0 |
C2Si–5Zn |
1.95 |
0.05 |
0 |
1.00 |
1.88 |
0 |
C2Si–10Zn |
1.90 |
0.10 |
0 |
1.00 |
3.72 |
0 |
C2Si–5Cu |
1.95 |
0 |
0.05 |
1.00 |
0 |
1.85 |
C2Si–10Cu |
1.90 |
0 |
0.10 |
1.00 |
0 |
3.67 |
2.2 Mechanical analysis
As for mechanical test, the cylindrical paste specimens (Ø 6 × 9 mm) were prepared in a stainless steel mold with a pressure of 2 MPa (to remove the differences in microstructure in the initial pastes) and then stored in 100% humidity at 37 °C for a given interval (1, 3, 7, and 28 d) in advance. The mechanical test was conducted on a universal testing machine (Instron) until failure at a loading rate of 1 mm min−1 to obtain compressive strength. Six replicates were carried out for each group, and the results were expressed as mean ± standard deviation (SD).
2.3 Setting time
Initial (I) and final (F) setting times were measured with the Vicat needle according to ISO9597-1989E.17 The initial setting time is defined as the time necessary so that the light needle (280 g, diameter 1.13 mm) plunges into the paste and have a span of 5 ± 1 mm to the tube bottom. The final setting time is defined as the time necessary so that heavy needle (350 g, diameter 2.0 mm) no longer leaves a visible print on the surface of the paste. Six specimens were tested for each group. Six replicates were carried out for each group, and the results were expressed as mean ± SD.
2.4 In vitro biodegradation analysis
As for degradation (weight loss) test, the cylindrical paste samples (Ø 6 × 2 mm) were prepared with 2.0 g powder (W0) and then stored in 100% humidity at 37 °C for 7 d to set. The degradation behavior was estimated in 0.05 mM Tris buffer (pH 7.4; 37 °C) with a cement-surface-area to solution–volume ratio of 0.1 cm−1. A quarter of the aqueous buffer was refreshed every day and the pH values in the buffers were measured by pH meter (Hanna Instruments, pH211). Then, the samples were gently rinsed with absolute ethanol, and dried (75 °C) up to mass constancy before weighing (Wt). The weight loss was expressed as (W0 − Wt)/W0 × 100%.
2.5 In vitro bioactivity analysis
The SBF, with ion concentration nearly equal to that of human blood plasma, was prepared according to the Kokubo's recipes.24 The paste samples (Ø 6 × 2 mm) were stored in 100% humidity at 37 °C for 7 d and then immersed in SBF at 37 °C with the cement-to-SBF ratio of 1.0 mg ml−1. At the preset time point (4 h to 28 d), 2.0 ml of supernatant was refreshed with fresh SBF for inductively coupled plasma (ICP) analysis. The specimens were gently rinsed with ethanol and dried in a vacuum overnight for SEM observation and energy dispersive X-ray spectrometry (EDX).
2.6 Cell viability assay
Cell viability was measured using the CCK-8 method in accordance with the manufacture's protocol. The 7-d-set cements were crushed into powders, and the extraction was carried out according to provisions reported.17 Briefly, 5 g of powder in 50 ml α-MEM was performed at 37 °C, in a humidified incubator with 5% CO2 for 24 h. The supernatant was then thoroughly filtered a 0.22 μm Millipore membrane and serial dilutions of paste dissolution were prepared (100.0, 50.0, 25.0, 12.5, 6.25 and 3.125 mg ml−1).
MC3T3-E1 cells were obtained from the China Academy of Science Cell bank (Shanghai, China) and seeded into individual wells of a 96-well plate at a concentration of 5 × 103 cell per ml. The cultures were maintained in α-MEM supplemented with 10% fetal calf serum and 1% antibiotics. After culturing for 24 h, the original medium was then replaced with the prepared extracts, with fresh culture medium added to the control groups (regarded as hour 0), and cultures were maintained at standard conditions and collected after 24, 72, and 120 h, respectively. Then, CCK8 was added (20 μl per well) and cultured 1 h at 37 °C. Optical density (OD) at 450 nm was measured with a microplate reader (ELX800, Bio-TEK). The optical density represents the proliferation of MC3T3-E1 cells. The cements were also dehydrated in gradient ethanol (70, 80, 90, 95, 100 (v/v)) for 5 min, respectively. The samples were immersed in isoamyl acetate for 20 min and the vacuum-dried at 40 °C for 4 h. After that, the cell morphology on the cements was observed using SEM.
2.7 Endodontic space sealing ex vivo
This study was approved by the ethics committee of the First Affiliated Hospital, School of Medicine of Zhejiang University (ZJU2014-1-05-094), following informed consent taken from all patients. The freshly extracted teeth with mature apices and single canal configuration were obtained. Sixty freshly extracted crack-free human teeth, single-rooted, were selected in this study. External calculus and periodontal ligament tissue were carefully scraped with a scalpel blade. The crowns of the teeth were removed at the cement to enamel junction. Each root canal was drilled to the apical foramen with sequential files beginning with size 15k-file, then canals were mechanically prepared in a crown down technique with a series of ProFile (Dentsply Maillefer, Tulsa, OK, USA). In order to eliminate debris, 5.25% NaOCl and 0.9% NaCl were used as irrigation solutions between each file size. The specimens were delivered into the canals using a lentulo spiral.
All of the teeth were divided into five groups of 10 canals, each group being covered with a nail varnish layer leaving only the apex (1 mm) free for penetration in order to assess the degree of infiltration in the coronal–apical direction and filled with one of the paste. The remaining ten teeth were divided equally into positive and negative control groups. For positive control, the teeth did not filled with the paste and the surface was coated using nail varnish. The teeth without the filler and nail varnish was the negative control. All groups were kept at 37 °C, 100% humidity for 24 h, and then stored in air at room temperature for 12 h, and finally, immersed in 0.5% Rhodamine B at 37 °C for 72 h under vacuum for the first 10 min.
To measure the length of dye penetration, cross-sections were made longitudinally with a low speed carborundum disk, and digital images of specimen's cross-sections were photographed under a stereomicroscope (Olympus SZX7) equipped ToupCam™ Industrial Digital Camera TP705000A (China), then the maximum lengths of penetrations which occurred between the filing materials and the dentinal walls were measured in the longitudinal linear direction by image processing program ImageJ (NIH, Bethesda, MD, USA).
2.8 Antibacterial activity in vitro
Direct antimicrobial activity of the cement was tested by agar diffusion method using P. gingivalis (ATCC33277), and E. faecalis (ATCC19433). The experiment was carried out according to standard guidelines approved by the Tongji Stomatology School of Wuhan University Ethics Committee (WHU2009-3-07-04). E. faecalis were cultivated in brain-heart infusion broth aerobically at 37 °C for 1 d and P. gingivalis was grown in BHI broth supplemented with hemin and vitamin K anaerobically at 37 °C for 3 days to a concentration of 0.5 McFarland standard, which corresponds to approximately 3 × 108 CFU ml−1. A sterile cotton tipped swab was used to inoculate the bacterial suspension onto the agar plate to achieve a lawn of growth. For E. faecalis, Tryptic Soy Agar was used, and for P. gingivalis, Columbia blood agar was used.
The fresh paste was injected into cylindrical mold to form Ø 6 × 2 mm discs and the specimens were stored in at 37 °C, 100% humidity for 7 d. Then, the disks were maintained at room temperature for 1 h and incubated at 37 °C for 7 d anaerobically. Finally, the inhibition zone around the cements was determined by measuring its diameter (mm) including the diameter of the specimen (6 mm) using a 0.1 mm-precision rule.
2.9 Statistical analysis
Statistical analysis was performed by using SPSS software (IBM). Experimental results were expressed as means ± SD. Statistical analysis was carried out using one-way ANOVA, and a p-value of less than 0.05 was considered statistically significant.
3. Results and discussion
3.1 Characterization of the Zn-/Cu-doped C2Si
The C2Si–xZn or C2Si–xCu powders, in which Zn or Cu ions can occupy some Ca sites in the crystalline network, was prepared by a sol–gel process. The pure β-C2Si and Zn-doped β-C2Si (i.e. C2Si–xZn) were white, but the Cu-doped β-C2Si (i.e. C2Si–xCu) changed light grey with increasing the Cu content due to the contribution of colorful cupper ions. As shown in Fig. 1A, on comparing with the standard XRD pattern of β-C2Si (JCPDS 33-0302), it was clearly showed that the colloidal SiO2 and Ca(NO3)2·4H2O, free of Zn/Cu doping, could produce β-C2Si phase, even though minor patterns for CaO could be observed. This result is consistent with the previous study, in which the free CaO could not be completely avoided during β-C2Si synthesis with stoichiometric Ca/Si molar ratio of 2.0 in the starting materials by a sol–gel route.4 However, the peak intensity (37.36°, 53.84°/2θ) for CaO tended to decrease with the doping of Zn or Cu ions into β-C2Si. It is reasonable to postulate that these changes are attributed to the lower Ca/Si molar ratio of 2.0 in the starting materials and slow hydrolysis and condensation reactions of colloidal SiO2 and Ca(NO3)2 by Zn or Cu doping to form silica gel, which resulted in the decrease of free CaO in the powders. The grain size and the surface morphologies of the samples after setting for 1 d shown by the SEM micrographs (Fig. 1B) indicated that the particle size was below 2 μm and exhibited particle-like shape with agglomerative morphologies. The β-C2Si indicated more compact structures with smaller agglomerate grains. As can be seen from Fig. 2, the particle size of pure β-C2Si was 489 nm, whereas the mean diameter of 5% and 10% Zn-doped β-C2Si was from 439 nm to 467 nm. In the 5% and 10% Cu-doped β-C2Si, the characteristic particle scale was set by the grain size, typically between 423 and 427 nm. It is suggested that the foreign ions both inhibit the growth of the β-C2Si particles.
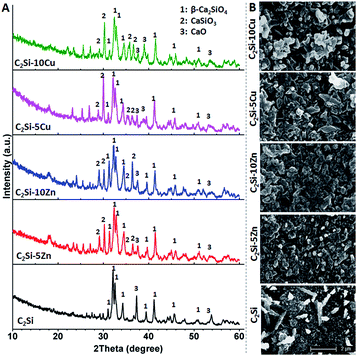 |
| Fig. 1 (A) XRD patterns of the β-C2Si powders without and with doping Zn/Cu. (B) SEM images of the surface and fracture morphologies of the cements after setting 1 d. | |
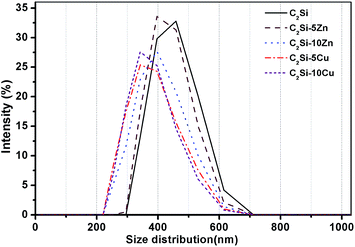 |
| Fig. 2 Particle size distribution of the β-C2Si powders without and with doping Zn/Cu. | |
3.2 Setting time and compressive strength
The paste setting time and sample compressive strength are both correlative factors in root canal therapy. Generally, the composition of cement, the particle size, foreign ion, and the l/p ratio play important parts in the setting time.25 Previous study reported that the smaller particle size of Ca-silicate-based cement lead to faster setting time and slower hydration speed.26 Our experiments were performed to evaluate the influence of Zn or Cu doping on the setting time and compressive strength of β-C2Si cement. Fig. 3 shows that an increase in setting time was observed with the Zn or Cu doping in β-C2Si. The data revealed that the setting time of C2Si–xZn was longer than that of the C2Si–xCu, and shows significantly difference (p < 0.05) in comparison with the pure β-C2Si. Meanwhile, increasing the substitution ratio of Ca by Zn or Cu from 5% to 10% resulted in a minor increase in setting time. This result implies Zn-/Cu-substituting-Ca in β-C2Si may have a deceleration effect on hydration reaction rate. It is reasonable to assume that the ion effect is the main factor to control the hydration reaction with regard to the particle size.
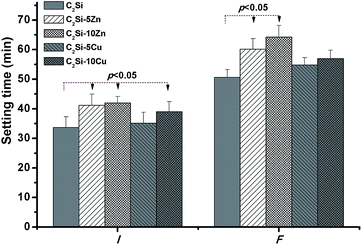 |
| Fig. 3 Bar graph representing the variation in the mean values of setting time of the β-C2Si cements without and with doping Zn/Cu. | |
The effect of Zn or Cu doping on compressive strength with prolonging time is shown in Fig. 4. It is clear to see that the compressive strength increased with the prolongation of setting time. Moreover, with doping of foreign ions, especially with Zn, there was a remarkable effect on decreasing compressive strength. It is well accepted that when β-C2Si reacts with water, a nanoporous and amorphous calcium silicate hydrate (CSH) gel is deposited on the original β-C2Si. As time proceeds, the CSH gels polymerize, harden and form a solid network which is associated with the densification and increase of the mechanical strength.27 The increase of porosity and the slow hydration speed are due to development of microstructure of the paste and are associated with a decrease of the strength.17 The β-C2Si hydrated more rapidly and possessed more compact structure compared with Zn-/Cu-doped pastes due to the CSH gel formed as the main product conformed a dense internal structure, which suggests that some specific foreign ion doping can be especially able to offer lower mechanical strength. This can also be demonstrated from Fig. 4 that the low Zn or Cu substitution ratio was more favorable for compressive strength than those with high Zn or Cu content in the primary powders. It is reasonable to consider that high substitution ratio of Zn or Cu in β-C2Si crystalline network may interfere with the oriented growth of the C–S–H phase, so that Zn or Cu doping would be partly unfavorable for the development of strength in the hydraulic cements.
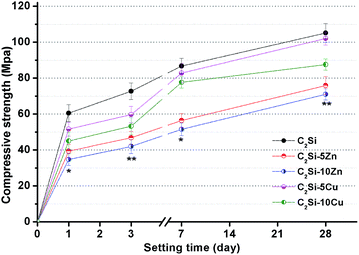 |
| Fig. 4 Mechanical strength of the C2Si cements without and with doping Zn/Cu after setting for different time stages at 37 °C and 100% relative humidity. *p < 0.05, compared with β-C2Si; **p < 0.01, compared with β-C2Si. | |
3.3 Degradation and pH variation in vitro
It is known that insolubility or low solubility is the prerequisite characteristic of the root canal sealer which is of utmost importance and necessary for successful results. It is worth noting that Zn and Cu ions are key parameters controlling the biodegradation of the C2Si–xZn and C2Si–xCu cements in vitro and in vivo.28–30 Saghiri et al.31 modified the particle size of WMTA, which significantly increased the Ca ions release and pH value at all working time, finally remarkably enhanced the mineralized matrix nodule formation to attain required clinical outcomes. As shown in Fig. 5, the degradation rates of the C2Si–xZn or C2Si–xCu cements were both immersion time- and Zn/Cu content-dependent. After 72 h of immersion, the weight loss of β-C2Si, C2Si–5Zn, C2Si–10Zn, C2Si–5Cu and C2Si–10Cu was 10.66%, 10.77%, 9.30%, 12.96% and 11.84%, respectively. Afterwards, the material residue of C2Si–xZn and C2Si–xCu showed slower degradation by 26–42% (32%/42% to 26%/42%) in comparison with the β-C2Si cement after 4 weeks (28 d). This indicates that existence of Zn or Cu in the primary β-C2Si crystalline powders leads to retardation of biodegradation of the hydrated cements, especially Zn incorporation. However, the low Zn or Cu substitution ratio slowed down the degradation rate of the β-C2Si cements compared with high Zn or Cu content in the cements.
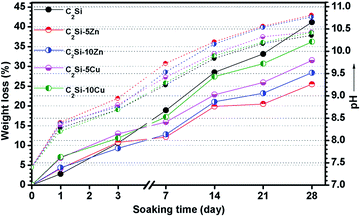 |
| Fig. 5 Weight loss of the cements and changes in pH value (dot-lines) during soaking in Tris buffer. | |
Fig. 5 also shows the time-dependent pH changes of the cement-immersed media. It is clear that the pH values in all solutions increased quickly from the initial value of 7.40 to 8.21–8.40 within 24 h owing to the quick reaction of free CaO with water that producing calcium hydroxide (CH) and releasing hydroxide ions (OH−). Particularly, the pH values strongly increased in solution within 7 d, mainly due to the production of C–S–H and calcium hydroxide (CH) byproducts from the β-C2Si reacted with water. Afterwards, the pH increased mildly with prolonging time possibly due to slow reaction of β-C2Si with water as in the process. Furthermore, the pH level of C2Si–xZn increased more quickly than the pure β-C2Si and C2Si–xCu. These results also indicate that C2Si–xZn reacts slower with water than the β-C2Si and C2Si–xCu.
3.4 Bioactivity in vitro
It is well known that the β-C2Si-based cements owns excellent bioactivity like apatite mineralization.4,7,27 Apatite-mineralization of biomaterials helps to the integration with host bone tissue and enhances osteogenic cell activity of biomaterials, including proliferation and differentiation.32 The essential condition for biomaterial to bond with tissue is the formation of a surface hydroxyapatite (HA) layer in the body environment. It can be seen from Fig. 6 that globular structures with typical bone-like apatite morphology that were about 1–2 μm in diameter were deposited on the surface of all cement discs after soaking for 1 d, and furthermore the needle-like morphology similar to those formed in bioactive materials under physiological conditions were deposited onto the sample surfaces after 7 d of immersion in SBF, implying good in vitro bioactivity. Moreover, more deposited apatite on the surface of C2Si–xCu was observed in SBF for 1 d (Fig. 6J and M).
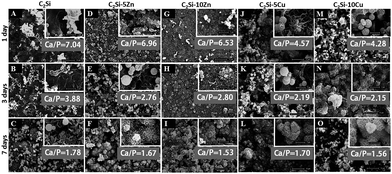 |
| Fig. 6 SEM images of the surface of the pure β-C2Si cement (A–C), C2Si–5Zn cement (D–F), C2Si–10Zn cement (G–I), C2Si–5Cu cement (J–L),and C2Si–10Cu cement (M–O) after soaking in SBF for 1–7 days. Bar: 10 μm. | |
Fig. 7 shows the changes in ions concentrations in SBF during soaking the samples for 1–28 d. The doping of foreign ions into β-C2Si, especially doping Zn, showed a rapid release profile of Ca2+ from SBF in the early 2 weeks, and then a very slow increase in Ca concentration (Fig. 7A). As for the Si concentration, the β-C2Si showed the fastest release rate of Si but the C2Si–xCu showed a slowest increase in Si concentration (Fig. 7B). Zn2+ or Cu2+ ions were released mildly from the C2Si–xZn and C2Si–xCu cements with increasing soaking time. Meanwhile, the increases of Zn or Cu concentration ions were consistent with the increase of Zn or Cu substitution ratio in the C2Si powders (Fig. 7C and D). Previous investigation confirmed that the smaller was particle size of Ca-silicate-based cements, the higher was the specific surface area of the powers, and thus this surface reaction would contribute to a better hydration and resulting in more Ca ion release.31 The crystalline network of C2Si–xZn or C2Si–xCu powders, in which Zn or Cu ions can occupy some Ca sites was stable compared with the pure C2Si. The tendency towards hydration process was probably retarded by Zn/Cu doping. Therefore, the structure stability and chemical compatibility of Zn/Cu-doped-C2Si coupled with difference of Ca and Si concentration in SBF.
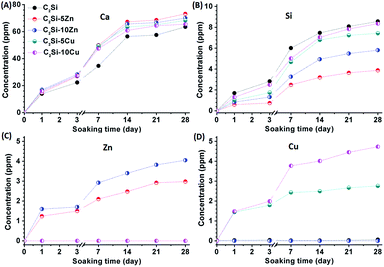 |
| Fig. 7 Changes in ions concentrations in SBF during soaking pure β-C2Si, C2Si–xZn and C2Si–xCu for 1–28 d. (A) Ca, (B) Si, (C) Zn, (D) Cu. | |
These results suggest that the C2Si–xZn and C2Si–xCu paste possess excellent apatite-mineralization ability in simulated biological solution. In general, HA layer deposited on the material surface under the physiological conditions plays an essential role in the formation, growth and maintenance of the tissue–biomaterial interface, indicating bioactivity in vitro.24 Our results (SEM observation and ICP analysis) suggest that the β-C2Si could induce the formation of a HA layer in vitro and moreover, with part substitution of Ca by Zn or Cu in β-C2Si, it showed that low levels of Zn or Cu release from these materials may potentially assist in accelerating HA deposition.
3.5 Cell viability
The adhesion and growth of MC3T3-E1 cells on pure β-C2Si and Zn/Cu-doped C2Si samples were shown in Fig. 8 and 9. It is seen that the osteogenic cells could adhere well on the cements and grew well after 1 and 5 d (Fig. 8). Fig. 9 indicates the negative control exhibited low OD values with increasing incubation time. In contrast, the β-C2Si, C2Si–xZn and C2Si–xCu samples in the 3.125–25 mg ml−1 showed an increasing OD values from 1 to 5 d with an increasing incubation time. In particular, the OD values for the wells with 50–100 mg ml−1 cements were increased to nearly 2-fold higher than those in 3.125–25 mg ml−1 from 1 to 3 d, however, the OD values for 50–100 mg ml−1 cements were significantly (p < 0.05) lower than those in 3.125–25 mg ml−1 after 5 d of incubation. It was worth noting that the OD values of the C2Si–10Zn and C2Si–5Cu in 3.125–25 mg ml−1 were significantly (p < 0.05) higher than other samples in 3 and 5 d of incubation.
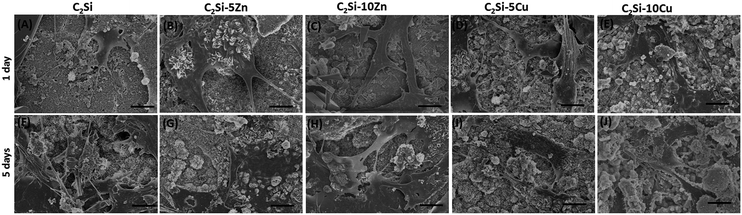 |
| Fig. 8 MC3T3-E1 cells morphology on the pure β-C2Si, C2Si–xZn and C2Si–xCu samples for 1 day (A–E) and 5 days (F–J), respectively. Bar: 10 μm. | |
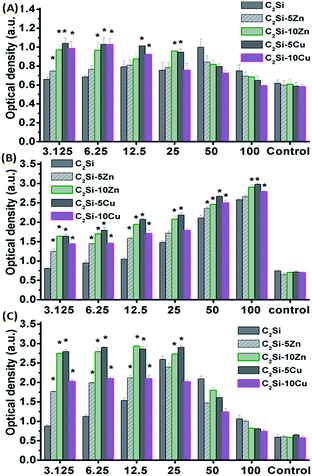 |
| Fig. 9 CCK8 assay for MC3T3-E1 cells attachment to pure β-C2Si, C2Si–xZn and C2Si–xCu at (A) 24 h, (B) 72 h, (C) 120 h with serial dilution made of the ionic products of paste dissolution (100.0, 50.0, 25.0, 12.5, 6.25, 3.125 mg ml−1). Cells cultured on the plate without specimen were as a control. | |
In this study, the CCK8 assay revealed that the dissolution extracts of Zn/Cu-doped β-C2Si cements in 3.125–50 mg ml−1 were not cytotoxic against MC3T3-E1 cells and showed an increasing stimulatory effect on cell proliferation, which demonstrates that calcium, silicate, zinc and copper ions are essential elements affecting the cell proliferation.28–30,33–35 Previous studies revealed that the dissolution extracts β-C2Si-based cements stimulated osteoblast-like cell proliferation and gene expression.9,17,27,36,37 However, the significantly lower cell viability of the cements in 100 mg ml−1 was showed in 5 d of incubation which might be associated with the higher pH value, Zn/Cu acceleration, or overhigh cells density which inhibit cell proliferate continuously. Indeed, Xiong et al.6 reported that the high content Zn-doped silicate-based cements was detrimental to proliferation of osteoblasts in the initial 3 d, but beneficial in the following 4 d. It is for this main reason that the different Zn content incorporation between the two cements.
3.6 Endodontic space sealing ex vivo
The β-C2Si-containing pastes have been used in endodontic therapy as a root filling material, and clinical studies have shown a high prevalence of healing after using this material system.12,38 Preventing leakage is essential to maintain a successful sealing of the root canal system. Another significant advantage of Ca-silicate-based cement is excellent apatite mineralization activity which may help to integrate with biomaterials on bone tissue.32 The in vitro apical microleakage was evaluated by dye penetration, as shown in Fig. 10. After placing specimens into Rhodamine B dye, penetration occurred in a linear pattern between the filing materials and the dentinal walls. As can be seen in Fig. 10A–G, all of the longitudinally sectioned root canal cements formed bond tightly with the cave wall, without forming gaps or voids in the interface zone between cement and tooth. Comparing the penetration depth of each material, the pure β-C2Si showed the most penetration value, but the C2Si–xZn showed the less microleakage distance, especially 5% Zn substitution. It can be seen from Fig. 10H that microleakage distances of β-C2Si, C2Si–xZn and C2Si–xCu filling materials were 2.27 ± 0.18 mm, 0.83 ± 0.14 to 1.14 ± 0.19 mm, 1.5 ± 0.18 to 1.67 ± 0.13 mm, indicating a significant difference (p < 0.05) between the pure β-C2Si and C2Si–xZn or C2Si–xCu. However, there were no significant differences (p > 0.05) between C2Si–xZn and C2Si–xCu for microleakage distances.
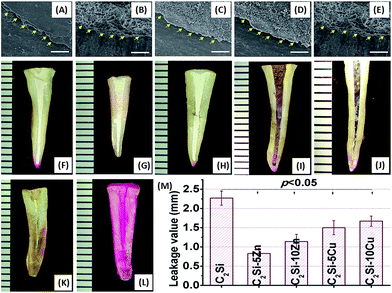 |
| Fig. 10 Sagittal sectional views (SEM observation and optical images) of leakage pattern. (A and F) Pure C2Si; (B and G) C2Si–5Zn; (C and H) C2Si–10Zn; (D and I) C2Si–5Cu; (E and J) C2Si–10Cu; (K) positive control; (L) negative control; (M) dye leakage values of cement. Arrows showing the interface of the cave wall and paste. Bar: 10 μm. | |
There was no microleakage in the positive control group. These results suggest that the mild hydration of the foreign ion-doped C2Si cements is more favorable for the enhancement of the anti-microleakage. This is possible that a slow hydration reaction and self-curing process benefit for the densification of the cement, but contrarily, the fast hydration is negatively effect the antileakage of the β-C2Si cement due to the porous nature in its internal structure.
3.7 Antibacterial activity in vitro
The primary objective of this study was to confirm the antibacterial activity of C2Si–xZn and C2Si–xCu. Bacterial infection after root canal treatment remains a significant one of the complications.39 Therefore, choosing a filling material with a high antibacterial activity helps to decrease or limit the growth of any remaining bacteria, even after treatment in infected defects.40 Various bacterial strains can be encountered during re-infection following root canal therapy. E. faecalis, which is responsible for most of human enterococcal infections, is the dominant enterococcus species and commonly associated with secondary root canal infection owing to failed endodontic therapy.1 Recent studies has shown that E. faecalis was selected as the model bacterium for various root filling materials antimicrobial efficacy study.41 P. gingivalis, as anaerobic microorganism detected in periodontal–endodontic combined lesions or necrotic pulp, was a major pathogenesis of periodontal disease.42
The antibacterial activity of Zn- or Cu-doped β-C2Si was carried out with E. faecalis and P. gingivalis using the diameter of inhibition zone by agar diffusion. The inhibition zone reflected magnitude of susceptibility of the microorganism. Strains that were susceptible to disinfectants exhibited larger inhibition zone, whereas those that were resistant exhibited smaller inhibition zone. It is seen from Fig. 11 that C2Si–xCu markedly inhibited the growth of P. gingivalis, and had greater antimicrobial effect than C2Si–xZn and C2Si. Moreover, there was a similar antimicrobial effect on E. faecalis between C2Si–xCu and C2Si–xZn. In addition, the more Zn/Cu is present in the cements, the lower is the survival of the bacteria. These results suggest that Cu ion substitution in β-C2Si has a considerable role on inhibiting P. gingivalis viability, but the incorporation of Zn or Cu into β-C2Si was found to have low antibacterial potential on E. faecalis.
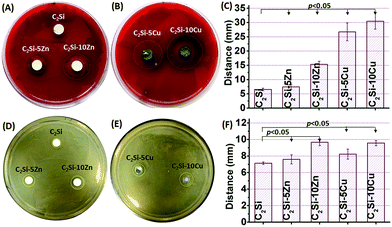 |
| Fig. 11 Antimicrobial activity of pure β-C2Si, C2Si–xZn and C2Si–xCu cement against P. gingivalis (A–C) and E. faecalis (D–F), respectively. | |
It is widely known that the antibacterial activity of Ca-based biomaterial is mainly based on several factors, including high pH and calcium ion release dissolved from the materials.43,44 In this study, during the hydration process, the C2Si–xZn and C2Si–xCu reacted with water to show a fast increase in CH, which released hydroxyl ions leading to the creation of an alkalic environment for bacteria to die. Saghiri et al. reported that by nano-sizing (40–100 nm) calcium silicate-based particles, the increased exposed surface area of the material would effectively elevate the pH and increase the calcium ion release in solution.31 Previous study has demonstrated that Cu-doped mesoporous bioactive glass which was a biodegradable material, can release Cu and Ca ions as the main antibacterial elements.28 Moreover, Chung et al. reported that Zn ions can inhibit the growth of S. mutans at a concentration of 10.0 ppm.20 In the present study, the Zn or Cu ions are revealed from C2Si–xZn and C2Si–xCu to show growth inhibition of the typical oral microorganisms causing pulpal necrosis and periapical lesions. Our results demonstrate that the level of Zn or Cu ions release from root filling materials is critical to act as antibacterial agent to inhibit the growth of bacterial strains.
4. Conclusion
In summary, we demonstrated that a small level of Zn or Cu ion substituting into β-C2Si cement could adjust their physicochemical properties and has a considerable effect on the good bioactivity and reasonable degradability compared to pure β-C2Si. Our studies also demonstrated that the new self-setting cements with Zn or Cu doping displayed good in vitro bioactivity, excellent anti-microleakage, and antimicrobial activity against P. gingivalis and E. faecalis. It is believed that such endodontic biomaterial based on Zn or Cu-doped β-C2Si is promising for endodontic treatment.
Acknowledgements
This work is supported by the Zhejiang Provincial Natural Science Foundation of China (LY15H140004), the National Science Foundation of China (81301326, 51372218), Health Bureau of Zhejiang Province (2013KYA108, 2014KYA260).
References
- P. N. R. Nair, Crit. Rev. Oral Biol. Med., 2004, 15, 348–381 CAS.
- M. Torabinejad and T. R. P. Ford, Endod. Dent. Traumatol., 1996, 12, 161–178 CrossRef CAS PubMed.
- G. S. Sachdeva, L. T. Sachdeva, M. Goel and S. Bala, Int. Endod. J., 2015, 48, 902–910 CrossRef CAS PubMed.
- X. Y. Yang, M. Liu, Y. Zhao, H. Y. Jia, S. Z. Xu, X. G. Li, X. Y. Chen, F. Zhang, C. Y. Gao and Z. R. Gou, J. Mater. Chem. B, 2014, 2, 3830–3838 RSC.
- T. H. Huang, S. J. Ding, T. Z. Hsu, Z. D. Lee and C. T. Kao, J. Mater. Sci.: Mater. Med., 2004, 15, 767–771 CrossRef CAS PubMed.
- K. Xiong, J. Zhang, H. S. Shi, J. Q. Liu, H. Wu, H. Y. Li and J. D. Ye, RSC Adv., 2015, 5, 8329–8339 RSC.
- W. N. Liu and J. Chang, J. Biomater. Appl., 2012, 27, 171–178 CrossRef PubMed.
- W. Y. Zhao, J. Y. Wang, W. Y. Zhai, Z. Wang and J. Chang, Biomaterials, 2005, 26, 6113–6121 CrossRef CAS PubMed.
- B. C. Wu, C. K. Wei, N. S. Hsueh and S. J. Ding, Int. Endod. J., 2015, 48, 268–276 CrossRef CAS PubMed.
- C. Mao, X. Chen, G. Miao and C. Lin, Biomed. Mater., 2015, 10, 025005 CrossRef PubMed.
- J. Y. Sun, L. Wei, X. Y. Liu, J. Y. Li, B. E. Li, G. C. Wang and F. H. Meng, Acta Biomater., 2009, 5, 1284–1293 CrossRef CAS PubMed.
- C. Prati and M. G. Gandolfi, Dent. Mater., 2015, 31, 351–370 CrossRef CAS PubMed.
- R. J. Mailloux, R. Hamel and V. D. Appanna, J. Biochem. Mol. Toxicol., 2006, 20, 198–208 CrossRef CAS PubMed.
- G. W. Guo and Y. X. Liang, Brain Res., 2001, 888, 221–226 CrossRef CAS PubMed.
- Z. G. Gou and J. Chang, J. Eur. Ceram. Soc., 2004, 24, 93–99 CrossRef CAS.
- M. Sun, A. Liu, C. Ma, H. Shao, M. Yu, Y. Liu, S. Yan and Z. Gou, RSC Adv., 2016, 6, 586–596 RSC.
- Z. G. Gou, J. Chang, W. Y. Zhai and J. Y. Wang, J. Biomed. Mater. Res., Part B, 2005, 73, 244–251 CrossRef PubMed.
- Y. Huang, X. J. Zhang, H. H. Mao, T. T. Li, R. L. Zhao, Y. J. Yan and X. F. Pang, RSC Adv., 2015, 5, 17076–17086 RSC.
- S. Eckhardt, P. S. Brunetto, J. Gagnon, M. Priebe, B. Giese and K. M. Fromm, Chem. Rev., 2013, 113, 4708–4754 CrossRef CAS PubMed.
- R. J. Chung, M. F. Hsieh, C. W. Huang, L. H. Perng, H. W. Wen and T. S. Chin, J. Biomed. Mater. Res., Part B, 2006, 76, 169–178 CrossRef PubMed.
- J. T. Kim, S. H. Baek, S. H. Lee, E. K. Park, E. C. Kim, I. S. Kwun and H. I. Shin, J. Med. Food, 2009, 12, 118–123 CrossRef CAS PubMed.
- M. Frangoulis, P. Georgiou, C. Chrisostomidis, D. Perrea, I. Dontas, N. Kavantzas, A. Kostakis and O. Papaclopoulos, Plast. Reconstr. Surg., 2007, 119, 837–843 CrossRef CAS PubMed.
- G. F. Hu, J. Cell. Biochem., 1998, 69, 326–335 CrossRef CAS PubMed.
- T. Kokubo and H. Takadama, Biomaterials, 2006, 27, 2907–2915 CrossRef CAS PubMed.
- M. Bohner and G. Baroud, Biomaterials, 2005, 26, 1553–1563 CrossRef CAS PubMed.
- W. N. Ha, D. P. Bentz, B. Kahler and L. J. Walsh, J. Endod., 2015, 41, 1146–1150 CrossRef PubMed.
- D. Correa, A. Almirall, R. Garcia Carrodeguas, L. A. dos Santos, A. H. De Aza, J. Parra and J. A. Delgado, J. Biomed. Mater. Res., Part A, 2014, 102, 3693–3703 CrossRef PubMed.
- J. Ye, J. J. He, C. J. Wang, K. Yao and Z. R. Gou, Biotechnol. Lett., 2014, 36, 961–968 CrossRef CAS PubMed.
- X. Y. Chen, X. L. Sun, X. Y. Yang, L. Zhang, M. Lin, G. J. Yang, C. Y. Gao, Y. B. Feng, J. Yu and Z. R. Gou, J. Mater. Chem. B, 2013, 1, 1316–1325 RSC.
- L. Bi, M. N. Rahaman, D. E. Day, Z. Brown, C. Samujh, X. Liu, A. Mohammadkhah, V. Dusevich, J. D. Eick and L. F. Bonewald, Acta Biomater., 2013, 9, 8015–8026 CrossRef CAS PubMed.
- M. A. Saghiri, A. Asatourian, J. Orangi, M. Lotfi, J. W. Soukup, F. Garcia Godoy and N. Sheibani, Dent. Traumatol., 2015, 31, 196–201 CrossRef CAS PubMed.
- P. Mazon, D. Garcia Bernal, L. Meseguer Olmo, F. Cragnolini and P. N. De Aza, Ceram. Int., 2015, 41, 6631–6644 CrossRef CAS.
- M. L. Zhang, C. T. Wu, K. L. Lin, W. Fan, L. Chen, Y. Xiao and J. Chang, J. Biomed. Mater. Res., Part A, 2012, 100, 2979–2990 CrossRef PubMed.
- R. J. Friederichs, H. F. Chappell, D. V. Shepherd and S. M. Best, J. R. Soc., Interface, 2015, 12, 20150190 CrossRef PubMed.
- M. Y. Shie, S. J. Ding and H. C. Chang, Acta Biomater., 2011, 7, 2604–2614 CrossRef CAS PubMed.
- L. J. Chen, P. Zhu, R. Y. Liu, Y. L. Zhang, T. Sun, Y. J. Liu and L. Q. Shao, J. Mech. Behav. Biomed. Mater., 2015, 44, 10–22 CrossRef CAS PubMed.
- X. M. Zhang, C. T. Wu, J. Chang and J. Sun, RSC Adv., 2015, 5, 72536–72543 RSC.
- P. Neelakantan, M. Nandagopal, H. Shemesh and P. Wesselink, Int. J. Adhes. Adhes., 2015, 60, 104–108 CrossRef CAS.
- P. N. R. Nair, S. Henry, V. Cano and J. Vera, Oral Surg. Oral Med. Oral Pathol. Oral Radiol. Endod., 2005, 99, 231–252 CrossRef CAS PubMed.
- A. Sugawara, K. Asaoka and S. J. Ding, J. Mater. Chem. B, 2013, 1, 1081–1089 RSC.
- X. Li, B. Han, X. Wang, X. Gao, F. Liang, X. Qu and Z. Yang, J. Mater. Chem. B, 2015, 3, 8884–8891 RSC.
- B. Gomes, R.
C. Jacinto, E. T. Pinheiro, E. L. R. Sousa, A. A. Zaia, C. C. R. Ferraz and F. J. Souza, Oral Microbiol. Immunol., 2005, 20, 211–215 CrossRef CAS PubMed.
- M. Parirokh and M. Torabinejad, J. Endod., 2010, 36, 16–27 CrossRef PubMed.
- S. Range, D. Hagmeyer, O. Rotan, V. Sokolova, J. Verheyen, B. Siebers and M. Epple, RSC Adv., 2015, 5, 43172–43177 RSC.
|
This journal is © The Royal Society of Chemistry 2016 |