DOI:
10.1039/C6RA00108D
(Paper)
RSC Adv., 2016,
6, 28887-28894
Luminescence and energy transfer of a color tunable phosphor: Tb3+ and Eu3+ co-doped ScPO4
Received
3rd January 2016
, Accepted 7th March 2016
First published on 8th March 2016
Abstract
A series of novel emission-tunable ScPO4:xTb3+, yEu3+ phosphors were prepared by a high temperature solid-state reaction. The phase purity was examined using X-ray diffraction refinement. X-ray photoelectron spectroscopy (XPS) and the crystal information, luminescence properties and energy transfer between Tb3+ and Eu3+ are analyzed systematically. The cross relaxation from 5D3 to 5D4 of single doped Tb3+ in the host is investigated. The energy transfer between Tb3+ and Eu3+ has been demonstrated by the decay times, which are ascribed to the dipole–dipole (d–d) mechanism, and the ηT reaches 54.4%. Additionally, the energy transfer critical distance between Tb3+ and Eu3+ was calculated to be about 12.95 Å. The emission color can be adjusted from green to yellow to orange-red by tuning the ratio of Tb3+/Eu3+. The ScPO4:0.03Tb3+, 0.025Eu3+ exhibits good thermal stability, indicating its great potential in w-LED applications.
Introduction
Recently, solid-state lighting based on white light-emitting diodes (w-LEDs) has become a prevailing trend for illumination technology due to their significant advantages such as high luminous efficiency, low energy consumption, long lifetime, and being environmentally friendly. Therefore there is great potential for them to be considered as the next generation of light sources.1–3 At present, in order to acquire the high quality white light, the w-LEDs could be fabricated with phosphors of three individual primary colours: red, blue and green, which can offer suitable correlated colour temperature and high colour rendering index.4
The rare earth (RE) have played a dominating role in the development of modern lighting and display fields because of their broad emission spectrums based on the 4f–4f or 5d–4f transitions. As the increasing demand of the white lighting, the researchers have devoted great efforts to obtain the color tuning of single phased phosphors with different doping via the energy transfer between RE ions.5–7 It is found that Tb3+ can be used as an activator in different hosts, and its emissions mainly attribute to the 5D3–7FJ (blue) and 5D4–7FJ (green). With the increasing concentration of Tb3+, the cross relaxation between the 5D3 and 5D4 energy level takes place owing to the interaction of the Tb3+ ions, which results in the enhanced green emission of 5D4–7FJ.8,9 The Eu3+ ion is also be one of the most frequently used red or orange-red emitting activators doping in the luminescent materials. It has better lumen equivalency and colour rendering for the reason that the emission line of Eu3+ ions is slight host-dependence.10,11 Moreover, researchers have paid more attention to the energy transfer processes involving the Tb3+ and Eu3+ ions in co-doping compounds in order to realize the colour tuning at present.7,12
To our best knowledge, the phosphates are considered as the excellent hosts for luminescent materials because of their good chemical stability and low cost. Especially the compounds YPO4 and ScPO4 represent orthophosphate that are structurally related to the lanthanide orthophosphate and with the properties of LnPO4.13,14 Up to now, there is not any related reports about the photoluminescence (PL) properties and energy transfer from Tb3+ to Eu3+ occurring in this host under UV excitation. Furthermore, the potential application of the ScPO4 phosphors for w-LEDs hasn't been involved. In our work, a series of ScPO4:xTb3+, yEu3+ phosphors have been observed via the high temperature solid-state reaction method. A systematic study on their crystal structure, luminescence properties, thermal stability, quantum efficiency, energy transfer between Tb3+ and Eu3+ and applications in w-LEDs were carried out in detail respectively. White LEDs was fabricated by combing an UV LED chip (λmax = 385 nm) with the ScPO4:0.03Tb3+, 0.01Eu3+ phosphors along with the commercial phosphors BAM:Eu2+. All corresponding LED optical parameters were demonstrated.
Experimental
ScPO4:xTb3+, yEu3+ phosphors investigated in this work were synthesized by a conventional solid-state reaction method. The raw materials include initial rare-earth oxides, Sc2O3 (99.99%), Tb4O7 (99.99%) and Eu2O3 (99.99%), and (NH4)2HPO4 (A.R.). All chemicals were used directly without any further purification and then were intimately mixed and milled with stoichiometric ratios in an agate mortar, and then sintered at 1200 °C for 4 h in the air to obtain the samples. Finally, the samples were furnace-cooled to room temperature, and were crushed and pulverized for various characterizations.
The crystalline phases of the as-prepared phosphors were identified by X-ray diffraction (XRD; D8 Advance diffractometer, Germany), using Cu-Kα1 radiation (λ = 0.15406 nm) at a tube-voltage of 40 kV and a tube-current of 30 mA with a step size of 0.02 and a scanning rate of 2° min−1. The step scanning rate (2θ ranging from 10° to 100°) used for Rietveld analysis was 2 s per step with a step size of 0.01. Powder diffraction data were obtained by the Rietveld method using the computer software Jana2006.15 The X-ray photoelectron spectroscopy (XPS) was obtained on an ESCALAB 250xi (ThermoFisher, England) electron spectrometer. Diffuse reflection spectra on as-synthesized phosphors powder samples were detected on a UV-vis-NIR spectrophotometer (SHIMADZU UV-3600) attached to an integrating sphere. BaSO4 was used as a reference standard. Room temperature photoluminescence (PL) measurements of all samples were recorded on a JOBIN YVON FluoroMax-3 fluorescence spectrophotometer equipped with a photomultiplier tube operating at 400 V, and using a 150 W Xe lamp as the excitation source. The photoluminescence decay curves and quantum efficiency were determined by a spectrofluorometer (HORIBA, JOBIN YVON FL3-21) with an integral sphere, and the 370 nm pulse laser radiation (nano-LED) was used as the excitation source. The EL spectrum was detected by the FLS 980 Spectrometer.
Results and discussion
The phase purity of all samples is examined by XRD. As illustrates in Fig. 1, the similar XRD patterns of samples ScPO4 (a), ScPO4:0.03Tb3+ (b) and ScPO4:0.03Tb3+, 0.02Eu3+ (c) were all corresponded to the standard pattern of tetragonal ScPO4 (JCPDS 84-336) and no other impurity phase exit. Therefore, the crystal structure of the samples were not significantly influenced by the dopant Tb3+, Eu3+ ions. For further demonstrating the phase purity and the occupancy of Tb3+ and Eu3+ ions in the host ScPO4, the Rietveld refinement of ScPO4 and ScPO4:0.03Tb3+, 0.02Eu3+ phosphors were analyzed via the Jana2006 program as shown in Fig. 2 and ScPO4 was served as an initial structural model. As the results revealed, neither the host nor the phosphors doped with Tb3+ and Eu3+ ions generated any impurity or secondary phases in ScPO4. ScPO4 crystallizes in the tetragonal system with space group I41/amd (141). The lattice parameters of the host were fitted to be a = b = 6.578 (7) Å, c = 5.796(3) Å, V = 250.5(5) Å3, the weighted profile R-factor (Rwp) and the expected R-factor (Rp) are 5.52% and 3.75%, respectively. As doped with Tb3+ and Eu3+, the parameters were a = b = 6.595(7) Å, c = 5.807(4) Å, V = 252.6(2) Å3. The refinement data became Rwp = 5.36% and Rp = 3.59%. Moreover the fractional atomic coordinates, occupancies and isotropic thermal parameters of this sample are summarized in Table 1. The volumetric expansion of the sample with Tb3+ and Eu3+ doping indicates that the Tb3+ and Eu3+ occupied the Sc3+ sites, which can be ascribed to the relationship of the ionic radius of the host cation Sc3+, and doping ions Tb3+, Eu3+ (REu3+ > RTb3+ > RSc3+). Fig. 3 shows the schematic illustration of the crystal structure of ScPO4 viewed from the c axis and the Sc3+ ions locate at the eight coordinated sites while the P5+ ions at the four coordinated sites.14
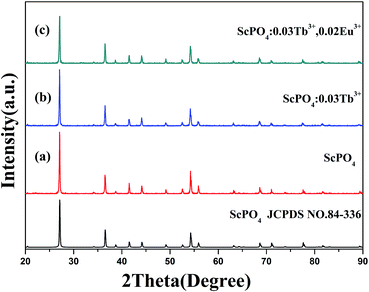 |
| Fig. 1 XRD patterns of as-prepared ScPO4 (a), ScPO4:0.03Tb3+ (b), ScPO4:0.03Tb3+, 0.02Eu3+ (c) phosphors and the standard pattern of JCPDS 84-336. | |
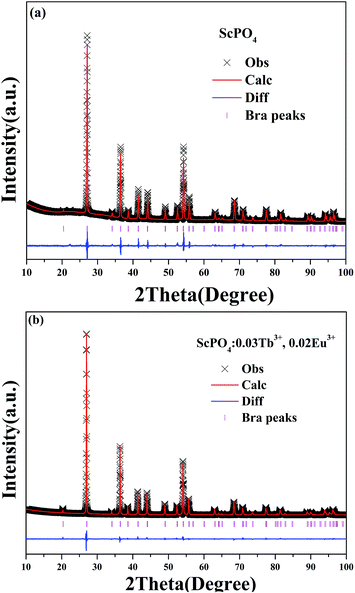 |
| Fig. 2 Powder XRD patterns (×) of (a) ScPO4 host and (b) ScPO4:0.03Tb3+, 0.02Eu3+ samples with their corresponding Rietveld refinement (solid line) and residuals (bottom). | |
Table 1 Fractional atomic coordinates and isotropic displacement parameters (Å2) of ScPO4 obtained from the Jana2006 software using X-ray powder diffraction data at room temperatureab
Atom |
x |
y |
z |
Uiso |
Occ. |
Space group: I41/amd (141); a = b = 6.578(7) Å, c = 5.796(3) Å, V = 250.5(5) Å3; 2θ-interval = 10–100°; Rwp (%) = 5.52, Rp (%) = 3.75, χ2 = 2.58. Space group: I41/amd (141); a = b = 6.595(7) Å, c = 5.807(4) Å, V = 252.6(2) Å3; 2θ-interval = 10–100°; Rwp (%) = 5.36, Rp (%) = 3.59, χ2 = 2.22. |
ScPO4 |
Sc |
0 |
0.75 |
0.625 |
0.0135(2) |
1 |
P |
0 |
0.75 |
0.125 |
0.0324(3) |
1 |
O |
0 |
0.9308(27) |
0.2929(32) |
0.0064(2) |
1 |
![[thin space (1/6-em)]](https://www.rsc.org/images/entities/char_2009.gif) |
ScPO4:0.03Tb3+, 0.02Eu3+ |
Sc |
0 |
0.75 |
0.625 |
0.0075(5) |
0.943 |
Tb |
0 |
0.75 |
0.625 |
0.0075(5) |
0.033 |
Eu |
0 |
0.75 |
0.625 |
0.0075(5) |
0.024 |
P |
0 |
0.75 |
0.125 |
0.0109(1) |
1 |
O |
0 |
0.9310(94) |
0.2938(8) |
0.0138(9) |
1 |
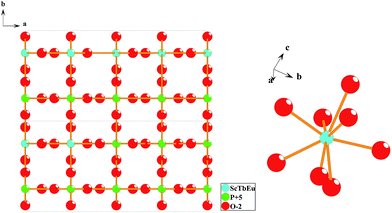 |
| Fig. 3 Schematic illustration of the crystal structure of ScPO4 viewed from the c axis, and the coordination environment around Sc3+. | |
To further determine the chemical states of europium and terbium, the X-ray photoelectron spectroscopy XPS technique was carried out on the sample Sc0.96PO4:0.03Tb3+, 0.01Eu3+ as shown in Fig. 4 The typical survey XPS spectrum reveals a series of photoelectron peaks corresponding to Sc2p1, P2p1, O1s, Tb3d5, Eu3d3 and C1s, respectively. The occurring of C peak is due to the adventitious hydrocarbon of the XPS instrument. The binding energies around 403.06, 133.46, 531.24, 1241.78 and 1136.46 eV correspond well to the Sc3+, P5+, O2−, Tb3+ and Eu3+ ions respectively.16,17 Moreover, there is no other ions including the divalent europium have been detected. We can deeply confirm the successful preparation of Tb3+ and Eu3+ co-doped ScPO4 phosphors combined with the XRD analysis.
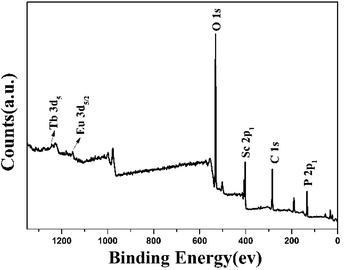 |
| Fig. 4 High-resolution of XPS survey spectrum of ScPO4:0.03Tb3+, 0.01Eu3+. | |
The excitation (PLE) and emission (PL) spectra of the sample ScPO4:0.03Tb3+ are shown in the Fig. 5(a). The PLE spectrum monitored at 550 nm presents strong bands from 200 to 500 nm. The spectrum centered at 230 nm and 275 nm assign to a spin-allowed 4f8–4f75d1 transition with higher energy (230 nm, ΔS = 0) and a spin-forbidden 4f8–4f75d1 transition with lower energy (275 nm, ΔS = 1) of Tb3+ ion, respectively.18,19 The others from 300 to 500 nm correspond to the intra-configurational 4f8–4f8 transitions from 7F6 levels to 5H6 (306 nm), 5D0 (323 nm), 5G2 (358 nm), 5D3 (376 nm) levels.21 It can be expected that the excitation wavelength at 370 nm can be found from the typical commercial chips in the near future. The PL spectra of the as prepared ScPO4:xTb3+ (x ≤ 0.09) consist of five main peaks within the scope of 400 to 700 nm which are due to the transitions of 5D3,4–7FJ (J = 3, 4, 5, 6) as labelled in the Fig. 5(b). It can be seen clearly that 5D4 emissions are stronger than 5D3 emissions. For the Tb3+ ion, there is an efficient cross-relaxation via the resonant energy transfer processes due to the similarity of energy gap between 5D3–5D4 (5915 cm−1) and 7F6–7F0 (6000 cm−1). It can be expressed as follows:20,21
|
5D3(Tb3+) + 7F6(Tb3+) − 5D4(Tb3+) + 7F0(Tb3+)
| (1) |
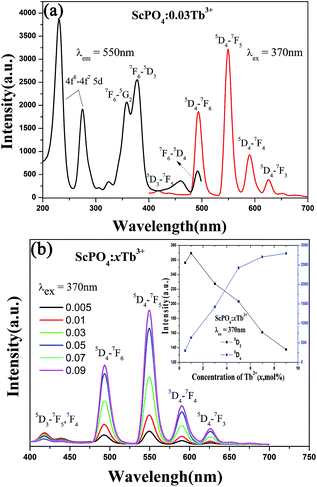 |
| Fig. 5 The spectral patterns of ScPO4:0.03Tb3+ (a); the PL spectra of ScPO4:xTb3+ (x ≤ 0.09) (inset: the 5D3 and 5D4 emission intensity with different concentrations of Tb3+) (b). | |
The Tb3+ levels are involved in several transitions owing to the high degeneracy and have a low energy ground state 7FJ (J = 6, …, 0) and excited states 5D3 and 5D4. Generally speaking, with different doping concentrations of Tb3+ in host matrix, the blue and green emissions can be emitted due to the transitions of 5D3 or 5D4 to 7FJ.22 In our work, when the doping concentration of the Tb3+ is increasing, the positions of the emission transitions have no shifts but the change of their intensities. As revealed in the inset of Fig. 5(b), the emission intensity of 5D3 was strengthened with the increasing of Tb3+ doping concentrations initially and then reduced after reaching its maximum intensity, while the concentration quenching does not take place on the 5D4 emissions even at the high Tb3+ concentrations (x = 0.09). At present the concentration quenching is under further investigation. A diagram of the energy levels of Tb3+ for the cross-relaxation processes is illustrated in the Fig. 6, revealing the energy transfer from the high level of 5D3 to the low energy level of 5D4. A similar phenomenon of Tb3+ have been reported in the host CaSc2O4,7 CaYAlO4,9 Y2O3,23 KSrPO4.24
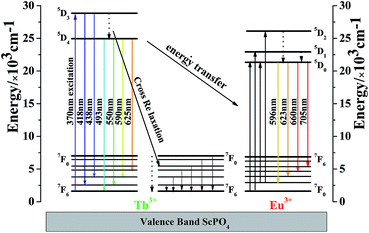 |
| Fig. 6 The energy level structure of Tb3+, Eu3+ and the energy-transfer mechanism among them. | |
As the Eu3+ singly doped ScPO4 host, the PL and PLE spectra are shown in the Fig. 7. It consists of two excitation bands from 200 to 300 nm, which is due to the host absorption centered at 215 nm and the charge-transfer band (CTB) between Eu3+ and O2− at the peak of 265 nm.25–27 The CTB is formed from the transition of 2p electrons of O2− to the empty 4f orbitals of Eu ions. The long wavelength side (300–500 nm) results from the features of f–f transitions of Eu3+ within its 4f6 configuration, corresponded to 7F0–5F3 (300 nm), 7F0–5H6 (325 nm), 7F0–7D4 (362 nm), 7F0–5L7 (384 nm), the strong sharp excitation 7F0–5L6 (397 nm) and the weak one 7F0–5D2 (480 nm) respectively.11,28 Under the excitation of 397 nm, we can find the representative emissions peaks (596 nm, 623 nm, 660 nm, 705 nm) associating with the transitions 5D0–7FJ (J = 1, 2, 3, 4).
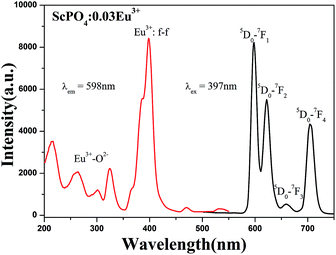 |
| Fig. 7 The spectral patterns of ScPO4:0.03Eu3+. | |
In order to further study the energy absorption of the ScPO4 phosphors, Fig. 8 show the diffuse reflectance spectra of ScPO4 host, ScPO4:0.03Tb3+ and ScPO4:0.03Tb3+, 0.025Eu3+. It can be seen obviously a strong energy absorption band in the 200–350 nm region from the host material. When the Tb3+ doped the host, it exhibit an obvious absorption from 230 to 275 nm assigned to the f–d transition of the Tb3+ ions. Moreover, with the introduction of Eu3+ ions, there is another narrow absorption lines at 397 nm ascribed to the f–f transition of Eu3+ ions. Above results are matching well with the PLE spectra (Fig. 5 and 7) and demonstrates the potential of the ScPO4:Tb3+, Eu3+ phosphors for the solid-state lighting.
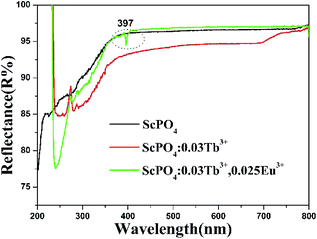 |
| Fig. 8 Diffuse reflectance spectra of ScPO4 host (a), ScPO4:0.03Tb3+ (b), and ScPO4:0.03Tb3+, 0.025Eu3+ (c). | |
As revealed in Fig. 9(a) series of samples with varied Eu3+ concentration and the fixed concentration of Tb3+ are synthesized and their PL spectra are observed schematically upon excitation of 370 nm to investigate their energy transfer (ET). One can see that the intensity of the Tb3+ emission is found to decrease monotonically with increasing of Eu3+ content due to the markedly energy transfer between Tb and Eu. These results provide an evidence for the ET between Tb3+ and Eu3+. Meanwhile, the intensity of the Eu3+ reaches a maximum at y = 0.025 and then occurs concentration quenching resulting in the weakening of emission intensity (Fig. 9(b)). The series of phosphors were eventually realized to tune from green, yellow to orange-red via co-doped Tb3+ and Eu3+. To observe the emission hue after doping Eu3+ concentration more directly, the Commision International de'LEclarirage (CIE) chromaticity coordinates, correlated colour temperature (CCT), quantum efficiency and CIE chromaticity diagram for the ScPO4:0.03Tb3+, yEu3+ and ScPO4:0.03Eu3+ phosphors are measured and are shown in Table 2 and Fig. 10, respectively. As the Table 2 depicts the variation of chromaticity coordinates (x, y) of the ScPO4:0.03Tb3+, yEu3+ which are calculated based on the corresponding emission spectrum. The phosphors can be modulated from green (0.2936, 0.4558) to orange-red (0.4553, 0.3547) as the inset of Fig. 8 shown. Meanwhile, it is found from Table 2 that the colour temperature of the samples was modulated from 6562 K to 2279 K with a decreasing trend and QE values for ScPO4:0.03Tb3+, xEu3+ declined with elevating the x value.
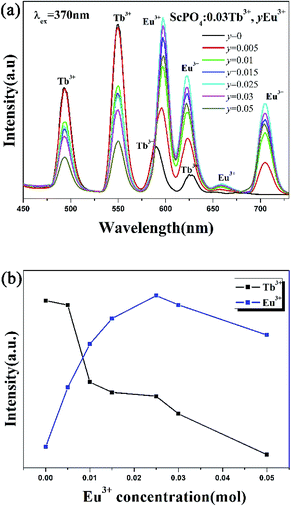 |
| Fig. 9 The PL spectra of ScPO4:0.03Tb3+, yEu3+ (y = 0–0.05) phosphors under N-UV excitation (λex = 370 nm) (a); dependence of the relative emission intensity of Tb3+ (550 nm) and Eu3+ (598 nm) in the ScPO4 phosphors as a function of Eu3+ mol concentration (b). | |
Table 2 The CIE chromaticity coordinates (x, y), correlated colour temperature (CCT) and QE values of ScPO4:0.03Tb3+, yEu3+ and ScPO4:0.03Eu3+ phosphors
Sample no. |
Sample composition (y) |
CIE coordinates (x, y) |
CCT (K) |
QE values (%) |
1 |
0 |
(0.2936, 0.4558) |
6562 |
15.46 |
2 |
0.005 |
(0.3570, 0.4217) |
4833 |
11.3 |
3 |
0.01 |
(0.4027, 0.3888) |
3556 |
7.02 |
4 |
0.015 |
(0.4239, 0.3870) |
3106 |
— |
5 |
0.025 |
(0.4444, 0.3821) |
2704 |
5.8 |
6 |
0.03 |
(0.4433, 0.3735) |
2722 |
— |
7 |
0.05 |
(0.4553, 0.3547) |
2279 |
3.09 |
8 |
— |
(0.6285, 0.3687) |
2045 |
— |
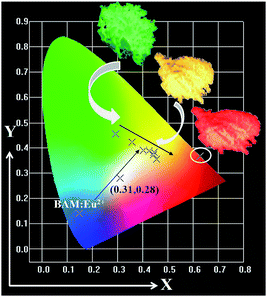 |
| Fig. 10 CIE chromaticity coordinates of ScPO4:0.03Tb3+, yEu3+, ScPO4:0.03Eu3+ and the commercial phosphor BAM:Eu2+ under 370 nm excitation and the digital images of 1, 7 and 8 at 365 nm in UV box. | |
To furtherly verify the existence of energy transfer between Tb3+ and Eu3+ in the samples of ScPO4:0.03Tb3+, yEu3+ (y = 0–0.050), we measured the luminescence decay curves of Tb3+ 550 nm emission and calculated the corresponding lifetimes of Tb3+ with the increasing concentration of Eu3+. Moreover, the energy transfer efficiency is also presented in the inset of Fig. 11(a). In general, the curves all can be well fitted based on the following non-exponential equation:29,30
|
 | (2) |
where
I(
t) stand for the luminescence intensities at time
t. The effective decay lifetimes at 550 nm of Tb
3+ are determined to be 1.34, 1.18, 1.09, 0.839, 0.797, 0.795 and 0.611 ms for
y = 0.005, 0.01, 0.02, 0.025, 0.04, 0.05, respectively. The lifetime of Tb
3+ emission are monotonously decreasing as the increasing concentration of Eu
3+, which demonstrates that the efficient energy transfer from Tb
3+ ions to neighboring Eu
3+ ions occur in the ScPO
4 host. Then the
eqn (3) can be used to estimate the Tb
3+–Eu
3+ ET probability (
PTb–Eu):
31,32 |
 | (3) |
where
τx and
τ0 mean the corresponding lifetimes of the sensitizer (Tb
3+) with and without the activator (Eu
3+) doping for the same sensitizer concentration. The values of the calculated
PTb–Eu are depicted in
Fig. 11(b) obviously. The continuous increasing of the ET probabilities with the different Eu
3+ content demonstrates an enhanced ET process between Tb
3+ and Eu
3+. Furthermore, the energy transfer efficiency
ηT from a donor Tb
3+ to acceptor Eu
3+ would be estimated according to the expression as follows:
33 |
 | (4) |
where
ηT means the energy transfer efficiency;
τs and
τso stand for the lifetimes of Tb
3+ in the absence and the presence of Eu
3+, respectively. As shown from the inset of
Fig. 11(a), the efficiency of energy transfer ascends continually from 11.9% to 54.4% with the increasing of the concentration of Eu
3+. The phenomenon is ascribed to be the decreased distance between the sensitizer ions (Tb
3+) and activator ions (Eu
3+).
21 As is well-known to us that the energy transfer between a sensitizer and an activator take place
via two interaction mechanism: exchange interaction or electric multipolar interaction. If the critical distance between the donor ions and acceptor ions is shorter than 5 Å, the exchange interaction occurs in the host.
34 Then in our work, the critical distance
Rc for energy transfer was well estimated by the following formula suggested by Blasse:
35 |
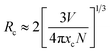 | (5) |
where
V is the volume of a unit cell,
N is the number of available sites for the dopant in the unit cell, and
xc is the critical concentration (the total concentration of Tb
3+ and Eu
3+ ions) at the point that the quenching occurs. Accordingly, the crystallographic parameter for ScPO
4 are
V = 250.12 Å,
N = 4 and
xc is about 0.055, which was derived from the quenching point (Tb
3+ content is 0.03, Eu
3+ content is 0.025) in the
Fig. 11. Therefore, basing on the
eqn (5),
Rc was calculated to be about 12.95 Å and it is much greater than 5 Å which illustrates the electric multipolar interaction plays a great role in the process of energy transfer between Tb
3+ and Eu
3+.
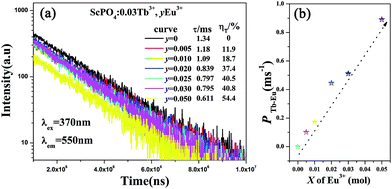 |
| Fig. 11 Decay curves, lifetime and ηT of ScPO4:0.03Tb3+, yEu3+ under the excitation of 370 nm (a). Energy transfer probability (PTb–Eu) as a function of x value of Eu3+ concentration (b). | |
In the light of Dexter's energy transfer theory of electric multipolar interaction and Reisfeld's approximation, the energy transfer present in the ScPO4 can be furtherly deduced by the following equation:36,37
|
 | (6) |
where
η0 and
η mean the luminescence quantum efficiencies of Tb
3+ in the absence and presence of Eu
3+ doping, respectively;
C is the concentration of Eu
3+, and
n = 6, 8 and 10 assigning to the dipole–dipole (d–d), dipole–quadrupole (d–q), and quadrupole–quadrupole (q–q) interactions, respectively.
38 Then the value of
η0/
η can be approximately computed by the ratio of related emission intensities as:
|
 | (7) |
In which the Iso is the intensity while only doping Tb3+ and Is is the intensities with different Eu3+ contents at fixed the concentration of Tb3+. Then in our work, the Iso/Is versus Cn/3 (n = 6, 8, 10) plots are depicted in Fig. 12. From the figure we can clearly see the linear fitting behaviour and compare the values of the fitting accuracy R2, so the results reveal that the energy transfer from Tb3+ to Eu3+ follows the dipole–dipole (d–d) interaction only when n = 6.
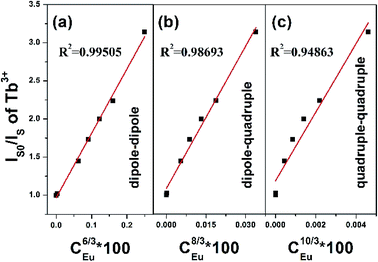 |
| Fig. 12 Dependence of Iso/Is of Tb3+ on CEu6/3 (a), CEu6/3 (b), CEu6/3 (c). | |
Fig. 13 gives the temperature-dependent emission spectra of ScPO4:0.03Tb3+, 0.025Eu3+. As the inset shows the trend of the relative emission intensity versus temperature, it can be concluded that the as-prepared samples exhibits an obvious temperature quenching which is due to the temperature-dependence of the electron–phonon interactions in both the ground state and excited state of the luminescence center and thermally activated photo-ionization of lanthanide.39,40 Moreover, as the increasing temperature up to 150 °C, the PL intensities of the characteristic peaks of Tb3+ and Eu3+ decreased to 79% and 79.5% of the initial value at room temperature, respectively. As we know, the high power LEDs lamp often works at 150 °C, which means the present phosphor possesses good thermal stability potentially.
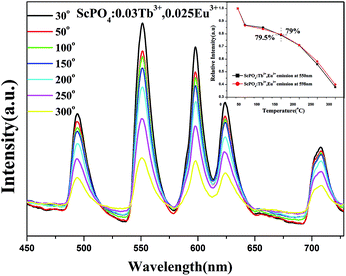 |
| Fig. 13 Temperature-dependent PL spectra of ScPO4:0.03Tb3+, 0.025Eu3+. The inset shows the emission intensity versus temperature. | |
In order to further demonstrate the potential application of ScPO4:0.03Tb3+, xEu3+, a phosphor-converted LED device was fabricated via using N-UV LED chips (λmax = 385 nm) combining a blue-emitting BAM:Eu2+ with yellow-emitting ScPO4:0.03Tb3+, 0.01Eu3+ phosphor as the inset given in Fig. 14. The EL spectrum of the fabricated LED device was shown in Fig. 14 under forward-basis current of 2 mA. The emission bands centered at 456 nm and 516 nm are attributed to the BAM:Eu2+ phosphor, while the other bands are the emission peaks of ScPO4:0.03Tb3+, 0.01Eu3+. The CIE coordinates, correlated color temperature (CCT) and color rendering index (Ra) of this fabricated w-LED lamp are determined to be (0.31, 0.28) as shown in Fig. 10, 7314 K and 72.5, respectively. The CRI value (Ra) is determined from the full set of the first eight CRIs shown in Table 3.
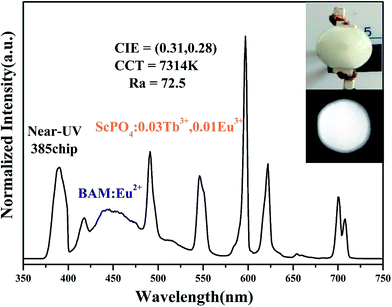 |
| Fig. 14 EL spectrum of a white-emitting N-UV-LED chip (385 nm) comprising of BAM:Eu2+ (blue) and ScPO4:0.03Tb3+, 0.01Eu3+ (yellow) phosphors driven by a 2 mA current. | |
Table 3 Full set of 14 CRIs and the Ra of the fabricated w-LED
Ra |
R1 |
R2 |
R3 |
R4 |
R5 |
R6 |
R7 |
R8 |
R9 |
R10 |
R11 |
R12 |
R13 |
R14 |
72.5 |
72.5 |
84.2 |
81.8 |
69.6 |
71.4 |
73.5 |
82.5 |
44.8 |
51.9 |
57.3 |
54.5 |
75.6 |
89.2 |
66.1 |
Conclusions
In summary, a single-phased, colour tunable emitting phosphors ScPO4 single doped with Tb3+ and co-doped with Tb3+ and Eu3+ ions have been synthesized by the conventional high temperature solid-state reaction. As for the samples with solely doped Tb3+, the energy transfer from 5D3 to 5D4 and the cross relaxation phenomenon occurs which strengthen the energy transition from the high energy level 5D3 to the low energy level 5D4. The ET process is verified via the decay curves with the energy transfer efficiency (the maximum is 54.4%) and the energy level structure, which is well matched with the dipole–dipole (d–d) mechanism. The ET probability (PTb–Eu) was calculated to give another proof for the existing of ET between Tb3+ and Eu3+. The emission colours of the obtained phosphors could be tuned from green (0.2936, 0.4558) to orange-red (0.4553, 0.3547) by controlling the doping of Eu3+. The white LEDs packaged by an UV LED chip with the ScPO4:0.03Tb3+, 0.01Eu3+ phosphors along with the commercial phosphors BAM:Eu2+ generate white light (0.31, 0.28) with color rendering index (Ra = 72.5) and a correlated color temperature (7314 K).
Acknowledgements
We gratefully thank the financial support from the National Natural Science Foundation of China (Grant No. 51472223), the Program for New Century Excellent Talents in University of Ministry of Education of China (Grant No. CET-12-0951) and the Fundamental Research Funds for the Central Universities (Grant No. 2652015008).
Notes and references
- S. Ye, F. Xiao, Y. X. Pan, Y. Y. Ma and Q. Y. Zhang, Mater. Sci. Eng., R, 2010, 71, 1 CrossRef.
- R. Zhang, H. Lin, Y. L. Yu, D. Q. Chen, J. Xu and Y. S. Wang, Laser Photonics Rev., 2014, 8, 158 CrossRef CAS.
- D. Chen, J. Eur. Ceram. Soc., 2014, 34, 4069 CrossRef CAS.
- S. Pimputkar, J. S. Speck, S. P. DenBaars and S. Nakamura, Nat. Photonics, 2009, 3, 180–182 CrossRef CAS.
- H. P. Ji, Z. H. Huang and Z. G. Xia, J. Phys. Chem. C, 2015, 119, 2038 CAS.
- Z. Liang, F. Mo and X. Zhang, Ceram. Int., 2014, 40, 7501 CrossRef CAS.
- Z. Hao, J. Zhang and X. Zhang, J. Electrochem. Soc., 2009, 156, H193 CrossRef CAS.
- D. Geng, M. Shang and D. Yang, J. Mater. Chem., 2012, 22, 23789 RSC.
- D. Geng, G. Li and M. Shang, Dalton Trans., 2012, 41, 3078 RSC.
- F. Du, Z. Rui and Y. Huang, Dalton Trans., 2011, 40, 11433–11440 RSC.
- Y. M. Peng, Y. K. Su and R. Y. Yang, Mater. Res. Bull., 2013, 48, 1946 CrossRef CAS.
- C. Zhang, H. Liang and S. Zhang, J. Phys. Chem. C, 2012, 116, 15932 CAS.
- J. L. Boldú O, E. Munoz P and M. M. Abraham, J. Chem. Phys., 1985, 83, 6113–6120 CrossRef.
- W. O. Milligan, D. F. Mullica and G. W. Beall, Inorg. Chim. Acta, 1982, 39, 60 Search PubMed.
- V. Petricek, M. Dusek and L. Palatinus, Z. Kristallogr. - Cryst. Mater., 2014, 229, 345–352 CAS.
- K. W. Chae, T. R. Park, C. I. Cheon, N. I. Cho and J. S. Kim, J. Lumin., 2011, 131, 2597–2605 CrossRef CAS.
- C. Zeng, Y. Hu, Z. Xia and H. Huang, RSC Adv., 2015, 5 Search PubMed.
- Z. Xia, J. Zhuang and L. Liao, Inorg. Chem., 2012, 51, 7202–7209 CrossRef CAS PubMed.
- J. Yang, C. Zhang, C. Li, Y. Yu and J. Lin, Inorg. Chem., 2008, 47, 7262–7270 CrossRef CAS PubMed.
- C. Liu, D. Hou and J. Yan, J. Phys. Chem. C, 2014, 118, 3220–3229 CAS.
- J. Zhong, W. Zhao and E. Song, J. Lumin., 2014, 154, 204 CrossRef CAS.
- X. Li, Y. Zhang and D. Geng, J. Mater. Chem. C, 2014, 2, 9924 RSC.
- H. Jiu, Y. Fu and L. Zhang, Micro Nano Lett., 2012, 7, 947 Search PubMed.
- Y. M. Peng, Y. K. Su and R. Y. Yang, Opt. Mater., 2013, 35, 2102 CrossRef CAS.
- B. Han, H. B. Liang, H. Y. Ni, Q. Su, G. T. Yang, J. Y. Shi and G. B. Zhang, Opt. Express, 2009, 17, 7138 CrossRef CAS PubMed.
- X. X. Li, Z. Chen and Y. H. Wang, J. Alloys Compd., 2009, 472, 521 CrossRef CAS.
- P. Dorenbos, J. Lumin., 2005, 111, 89 CrossRef CAS.
- H. Lai, A. Bao and Y. Yang, J. Nanopart. Res., 2008, 10, 1355 CrossRef CAS.
- Y. Y. Zhang, Z. G. Xia and H. K. Liu, Chem. Phys. Lett., 2014, 593, 189 CrossRef CAS.
- R. Y. Mi, C. L. Zhao and Z. G. Xia, J. Am. Chem. Soc., 2014, 97, 1802 CAS.
- M. Xie, T. Ye, Y. Huang, H. Liang and Q. Su, ChemInform, 2011, 42 Search PubMed.
- D. Wen, J. Feng, J. Li, J. Shi, M. Wu and Q. Su, J. Mater. Chem. C, 2015, 3, 2107–2114 RSC.
- J. Chen, Y. G. Liu, L. F. Mei, Z. Y. Wang, M. H. Fang and Z. H. Huang, J. Mater. Chem. C, 2013, 12, 1 Search PubMed.
- D. Geng, G. Li and M. Shang, J. Mater. Chem., 2012, 22, 14262 RSC.
- G. Blasse, Philips Res. Rep., 1969, 24, 131 CAS.
- D. L. Dexter, J. Chem. Phys., 1953, 21, 836 CrossRef CAS.
- Y. Jia, H. Qiao and Y. Zheng, Phys. Chem. Chem. Phys., 2012, 14, 3537 RSC.
- Z. G. Xia and R. S. Liu, J. Phys. Chem. C, 2012, 116, 15604 CAS.
- J. Zhou and Z. G. Xia, J. Mater. Chem. C, 2014, 2, 6978–6984 RSC.
- H. Luo, J. Liu and X. Zheng, J. Mater. Chem., 2012, 22, 15887–15893 RSC.
|
This journal is © The Royal Society of Chemistry 2016 |