DOI:
10.1039/C6RA00103C
(Paper)
RSC Adv., 2016,
6, 26709-26718
Preparation and characterization of laccases immobilized on magnetic nanoparticles and their application as a recyclable nanobiocatalyst for the aerobic oxidation of alcohols in the presence of TEMPO
Received
3rd January 2016
, Accepted 23rd February 2016
First published on 26th February 2016
Abstract
Laccase from Trametes versicolor was immobilized on a modified magnetic nanoparticle (MNP-Laccase) through a covalent attachment method. The morphology, structure, magnetic property and chemical composition of the immobilized laccase (MNP-Laccase) were characterized using scanning electron microscopy (SEM), Fourier transform infrared spectroscopy (FT-IR), alternating gradient force magnetometry (AGFM), energy-dispersive X-ray spectroscopy (EDX) and thermogravimetric analysis (TGA) techniques. The MNPs-Laccase retained the activity and exhibited higher resistance to pH and temperature changes. We have used MNPs-Laccase as a magnetically recoverable nanobiocatalyst for the mild, environmentally friendly and selective aerobic oxidation of benzylic and allylic alcohols to corresponding aldehydes in the presence of TEMPO as a redox mediator at room temperature. The magnetic nanobiocatalyst was easily and rapidly recovered by applying an external magnet device and reused up to 6 reaction runs without considerable loss of reactivity.
1. Introduction
Selective oxidation of benzylic alcohols to aldehydes is a particularly useful but challenging chemical transformation, as these aldehydes are important intermediates in the synthesis of other organic compounds, ranging from pharmaceuticals to plastic additives as well as in the processing of perfume and flavouring compounds and in the preparation of certain aniline dyes in the textile industry.1 Numerous oxidizing reagents in stoichiometric amounts have been traditionally employed to accomplish this transformation with considerable drawbacks such as being very atom inefficient, the use of toxic and/or hazardous reagents and generating environmentally pernicious wastes.2 To solve these drawbacks, the catalytic and selective oxidation with H2O2 or molecular oxygen as the oxidant becomes a promising orientation in this research field.3 Many examples of aerobic oxidation of alcohols catalyzed by transition metals especially palladium,4 platinum,5 ruthenium,6 copper7 and cobalt8 have been reported. Though these catalyst systems could efficiently perform oxidation, they may possibly leave toxic traces of heavy metals in the products. Therefore, it seems that there is still a great interest in developing efficient and non-metallic catalysts for the aerobic oxidation of alcohols from the view point of so-called green and sustainable chemistry.
The development of enzyme-catalyzed aerobic oxidation is highly attractive because of their great potential to remove pollutants and catalyze a great variety of redox processes with no hazardous side effects.9 Laccases (p-benzenediol: oxygen oxidoreductase; [E.C. 1.10.3.2)] are extracellular enzymes that belong to the multicopper polyphenol oxidases. The unique properties of laccases such as mild reaction conditions and substrate selectivity make them attractive for use in chemical synthesis.10 However, despite laccase having intrinsic appreciable stability, the enzyme is often easily inactivated in practical application due to a wide variety of environmental conditions. In addition, it is also difficult to be separated from the reaction system for reuse, which limits the further industrial application of laccase.11 In order to perform enzyme reuse and to improve its stability, laccase has been successfully immobilized on many different types of supports, such as activated carbon,12 silica,13 kaolinite,14 polymer beads and membranes15–17 and porous glass,18 by different mechanism like adsorption, entrapment and covalent attachment. Although these protocols represent considerable advances, the supported laccase biocatalyst is difficult to separate from the reaction mixture by classical methods such as extraction, filtration, or centrifugation. A possible strategy to circumvent this problem is to change the traditional supported matrix to materials that have magnetic properties, thus allowing easy separation of the catalysts by simply applying an external magnetic field. Other significant characteristics of magnetic support are large surface area, mobility, environmental compatibility, and high mass transference. Very recently, this approach has been applied for anchoring laccase on magnetic nanoparticles.19,20 However, to the best of our knowledge there has been no report on the use of supported laccase on magnetic nanoparticles (MNPs-Laccase) as recyclable catalysts in the aerobic oxidation of alcohols. The redox potential of laccase alone is not high enough to oxidize carbon–hydrogen bond of alcohols. To overcome this limitation it was used in combination with one of the redox mediators.21–23
In continuation of our studies on preparation and application of magnetically nanocatalysts,24–26 herein, we report for the first time the synthesis and characterization of MNPs-Laccase as a magnetically separable nanobiocatalyst for the selective aerobic oxidation of benzylic and allylic alcohols to the corresponding aldehydes in the presence of TEMPO as a redox mediator.
2. Results and discussion
2.1. Optimization of the reaction conditions for the preparation of MNPs-Laccase
The effects of activation time and concentration of glutaraldehyde and enzyme in the process of the synthesis of MNPs-Laccase were investigated. It was observed that the best glutaraldehyde concentration and activation time are 2% v/v and 2 h respectively (Tables 1 and 2, entry 1). Based on results, activity of MNPs-Laccase, loading capacity, and activity recovery of MNPs-Laccase reached 46.8 U, 5.08 mg g−1 activated MNPs and 78% respectively (Tables 1 and 2, entry 1). It should be mentioned when the glutaraldehyde concentration was increased up to 25% v/v, the enzyme activity was decreased (Table 2, entry 4). In order to determine the optimum amount of laccase to be immobilized on GMNPs, the amount of support was kept constant and different concentrations of laccase solution were used, from 1 to 7 U mL−1. As shown in Table 3, the best enzyme concentration was 6 U mL−1 (Table 3, entry 6). Therefore, the activation time, glutaraldehyde concentration and amount of laccase are chosen as 2 h, 2% v/v and 6 U mL−1 respectively for all further experiments.
Table 1 The effect of activation time on the preparation of GMNPs in glutaraldehyde concentration 2%
Entry |
Activation time |
Activity of MNPs-Laccase |
Loading capacity mg g−1 |
Activity recovery |
1 |
2 h |
46.8 |
5.08 |
78% |
2 |
6 h |
39.6 |
4.3 |
66% |
3 |
8 h |
34.2 |
3.71 |
57% |
Table 2 Synthesis of MNPs-Laccase in different glutaraldehyde concentrations in 2 h
Entry |
Glutaraldehyde concentration v/v |
Activity of MNPs-Laccase |
Loading capacity mg g−1 |
Activity recovery |
1 |
2% |
46.8 |
5.08 |
78% |
2 |
4% |
38.4 |
4.17 |
64% |
3 |
5% |
34.2 |
3.71 |
57% |
4 |
25% |
14.4 |
1.56 |
24% |
Table 3 The optimization of enzyme concentration in synthesis of MNPs-Laccase
Entry |
Enzyme concentration U mL−1 |
Activity of MNPs-Laccase |
Loading capacity mg g−1 |
Activity recovery |
1 |
1 |
3 |
0.32 |
30% |
2 |
2 |
7.6 |
0.82 |
38% |
3 |
3 |
13.5 |
1.46 |
45% |
4 |
4 |
21.2 |
2.30 |
53% |
5 |
5 |
30 |
3.26 |
60% |
6 |
6 |
46.8 |
5.08 |
78% |
7 |
7 |
45.6 |
4.95 |
76% |
The process of the immobilization of laccase on modified magnetic Fe3O4 nanoparticles is shown in Scheme 1.
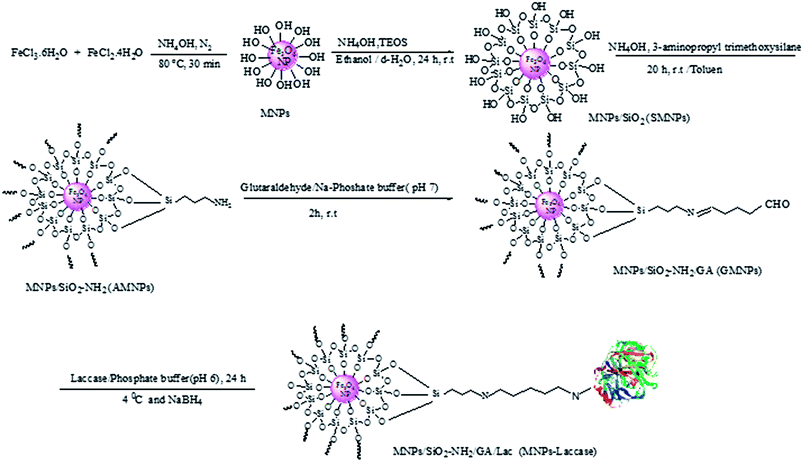 |
| Scheme 1 The process for the preparation of MNPs-Laccase. | |
2.2. Characterization of MNPs-Laccase
MNPs-Laccase was characterized by different techniques. Fig. 1a shows FTIR spectra for MNPs, SMNPs, AMNPs and GMNPs. As can be seen from FT-IR spectrum of MNPs (Fig. 1a) the strong bond at 574 cm−1 corresponds to Fe–O vibrations of the magnetite core and the stretching vibration at 3381 cm−1 is attributed to the O–H bonds which are attached to the surface iron atoms. The bond formation between MNPs and TEOS is confirmed by Fe–O–Si absorption bond that appears at 795 cm−1. The bond at 1061 cm−1 represents Si–O bonds, and bonds at 3386 and 1634 cm−1 correspond to the stretching and bending vibrations of Si–OH.27,28 After surface modification with APTMS, a band at 3100 cm−1, due to N–H stretching appears. The band is not very intense also because it is overlapped with the O–H band (3400 cm−1). The absorption band at 1547 cm−1 can be assigned to the N–H stretching vibration and NH2 bending mode of free NH2 group, which confirms the presence of the –NH2 group. Moreover, a band at about 2930 cm−1, due to C–H stretching of APTMS, appears. The bond formation between free –NH2 group of AMNPs and –CHO group of glutaraldehyde is confirmed by appearing of the bond at 1647 cm−1 assigned to the C
N stretching vibration and decreasing of intensity the bands at 1500 and 1550 cm−1 correspond to N–H vibration of AMNPs.
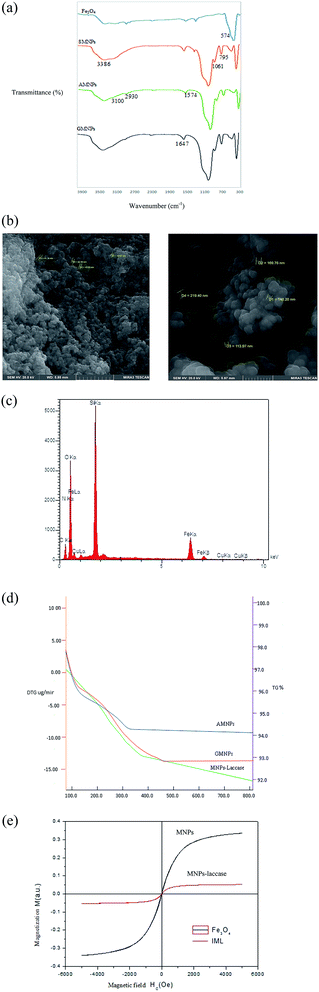 |
| Fig. 1 (a) FT-IR spectra of Fe3O4, SMNPs, AMNPs and GMNPs. (b) SEM images of MNPs (left) and MNPs-Laccase (right). (c) EDX spectrum of MNPs-Laccase. (d) TGA curves of AMNPs, GMNPs and MNPs-Laccase. (e) Magnetic curves of the MNPs and MNPs-Laccase at room temperature. | |
The scanning electron micrograph (SEM) for MNPs and MNPs-Laccase are shown in Fig. 1b. According to the SEM images, the bare MNPs were essentially fine and almost spherical, with the average diameter of 43 nm. MNPs-Laccase average particle size was 161 nm.
EDX microanalysis was performed to provide qualitative determinations of the elemental composition. The EDX spectrum of MNPs-Laccase confirmed the presence of Fe, Si, Cu, N and O (Fig. 1c).
One indication of bond formation between the nanoparticles and the catalyst can be inferred from TGA. The TGA curves of the AMNPs, GMNPs and MNPs-Laccase show the mass loss of the organic materials as they decompose upon heating (Fig. 1d). The weight loss at temperatures below 120 °C is due to the removal of physically adsorbed solvent and surface hydroxyl groups.29 The weight loss of AMNPs appears about 2% at 130–320 °C which is contributed to the thermal decomposition of the 3-aminopropyl groups. The weight loss of about 1.4% between 130 and 460 °C may be associated to the breakdown of the glutaraldehyde moieties. For MNPs-Laccase there is a well defined mass weight loss of 0.9% between 130–800 °C related to the breakdown of laccase moieties. On the basis of these result, the well grafting of APTMS, glutaraldehyde and laccase on the MNPs are verified.
Superparamagnetic particles are beneficial for magnetic separation, the magnetic property of the MNPs and MNPs-Laccase were characterized by AGFM. The room temperature magnetization curves of the MNPs and the MNPs-Laccase are shown in Fig. 1e. As expected, the bare MNPs, showed the higher magnetic value (saturation magnetization, Ms = 0.335 Oe)30 and the Ms value of MNPs-Laccase is decreased due to the silica coating and the layer of the grafted catalyst (Ms = 0.0519 Oe). The MNPs and MNPs-Laccase have a coercivity (Hc) of 10.48 and 12.58 Oe, respectively and the remnant magnetization (Mr) of ∼0.00335 and 0.00079 Oe, respectively. As a result, MNPs-Laccase has a typical superparamagnetic behavior31 and can be efficiently attracted with an external magnet.
2.3. Kinetic study of free laccase and MNPs-Laccase
The catalytic efficiency of an enzyme is determined mainly in terms of two kinetic parameters, namely, the Michaelis constant Km and Vmax. Km represents the substrate concentration at which the reaction rate is at half the maximum rate attainable. Vmax, on the other hand, is the maximum rate attained when all enzyme molecules are bound to the substrate. The value of Km is dependent on the characteristics of both the enzyme and the substrate, as well as on the experimental conditions, such as temperature and pH. Irrespective of procedures used for immobilization, Vmax decreased and Km increased for supported laccases, leading to a reduction of the catalytic efficiency with respect to free enzyme. Similar trends in Km values have been previously observed and were attributed to mass transfer limitations or to the conformational changes of the enzyme with a lower possibility to form a substrate–enzyme complex.19,32 Kinetic parameters of the free and the immobilized laccase, i.e. Km and Vmax value, were determined by using ABTS (0.3–10.0 mM) as substrate and plotting data to a double reciprocal Lineweaver–Burk plot (Table 4 and Fig. 2a). The apparent Km value of the MNPs-Laccase (2 mM) was 1.5 times higher than that of the free one (1.3 mM). The calculated Vmax value for ABTS in the presence of free laccase was 56 mM min−1, while the corresponding value for the MNPs-Laccase was 28 mM min−1 (Table 4).
Table 4 Kinetic parameters of free laccase and MNPs-Laccasea
Entry |
Enzyme |
Km [mM] |
Vmax [mM min−1] |
Each experiment was conducted in triplicate. Errors bars 5% were determined. ABTS was used as substrate. |
1 |
Free laccase |
1.3 |
56 |
2 |
MNPs-Laccase |
2 |
28 |
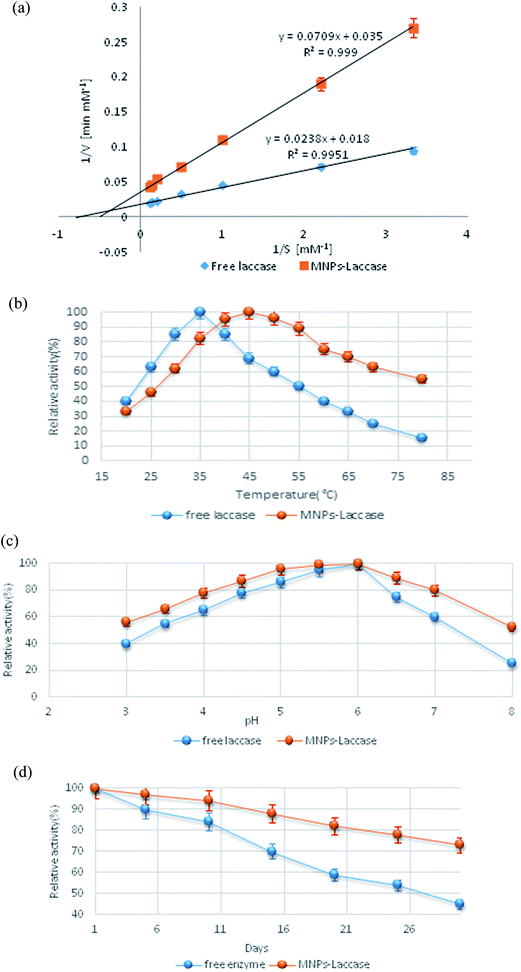 |
| Fig. 2 (a) Determination of Km and Vmax for free laccase and MNPs-Laccase system by Lineweaver–Burk plot method in ABTS aerobic oxidation reaction at T = 25 °C and pH 6. The ABTS concentration varied from 0.3 to 10 mM. (b) Effect of temperature on ABTS aerobic oxidation reaction catalyzed by free laccase and MNPs-Laccase. The relative activity was determined at various temperatures at pH = 6. Experiments were performed in triplicate. The error bars were determined (5%). (c) The effect of pH on the activity of the free laccase and MNPs-Laccase for the ABTS aerobic oxidation reaction (Na–acetate buffer for pHs 3.0–5.0 and Na–phosphate buffer for pHs 6.0–8.0) at 4 °C for 5 h. Experiments were performed in triplicate. The error bars were determined (5%). (d) Storage stability of free laccase and MNPs-Laccase were determined for the ABTS aerobic oxidation reaction in Na–phosphate buffer (100 mM, pH 6) at 4 °C. Experiments were performed in triplicate. The error bars were determined (5%). | |
2.4. Effect of temperature and pH on enzyme activity
The influence of temperature on relative activity of the free laccase and MNPs-Laccase are shown in Fig. 2b. MNPs-Laccase showed the substantial and important differences in trend of temperature activity in the temperature range studied (20–80 °C). As it can be seen in Fig. 2b maximum activities were observed at 35 °C and 45 °C for free laccase and MNPs-Laccase, respectively. Compared with the free laccase, the immobilized preparation gave a significantly broader profile at above 40 °C, the enzyme activity being maintained at over 70% within the temperature range 45–60 °C. Barely, 45 °C was a critical temperature for the free enzyme because after that temperature its activity retained 60%. Finally at 80 °C, the enzyme activity loss was calculated as approximately 45% for MNPs-Laccase and 85% for the free one. The MNPs-Laccase had a higher activity at high temperatures (50–80 °C) than that of the free counterpart. It is well known that the activity of immobilized enzymes, especially in the multipoint interaction, is more resistant against temperature than the free form.
According to the results (Fig. 2c), the free laccase and MNPs-Laccase exhibit maximal activity at pH 6. The MNPs-Laccase shows higher resistance to changes in pH value of the medium. MNPs-Laccase was stable at a broader range of pHs compared to free enzymes. 60% of the initial activity of free laccase was lost when pH was decreased from 6 to 3, while 56% of the relative activity of MNPs-Laccase remained. At an elevated pH value (pH = 8), the relative activity of MNPs-Laccase and free laccase was found to be 52.1% and 25.3%, respectively (Fig. 2c).
2.5. Storage stability
Generally, if an enzyme is in solution, it is not stable during storage, and the activity is gradually reduced. The free laccase and the MNPs-Laccase were stored in Na–phosphate buffer solution (100 mM, pH 6.0) at 4 °C for 30 days, and the storage stability of MNPs-Laccase was determined and compared with free laccase (Fig. 2d). At specific times, the activity was measured by ABTS assay following the warming of the solution at room temperature. The free laccase was found to retain only 45% of its original catalytic activity after 30 days of storage at 4 °C. Under identical storage conditions, MNPs-Laccase had 73% residual activity. There was a significant increase in the storage stability on MNPs-Laccase.
2.6. The catalytic application of MNPs-Laccase in the aerobic oxidation of benzylic/allylic alcohols in the presence of TEMPO
MNPs-Laccase was tested as magnetically separable heterogeneous nanobiocatalyst for the aerobic oxidation of benzylic/allylic alcohols to the corresponding aldehydes in the presence of TEMPO as a mediator in Na–phosphate buffer (0.1 M, pH 6)/MeCN (25
:
1) at room temperature. A series of alcohols including benzyl alcohol, 4-methoxybenzyl alcohol, 3-methoxybenzyl alcohol, 3,4-dimethoxybenzyl alcohol, 3,4,5-trimethoxybenzyl alcohol, 4-chlorobenzyl alcohol, 3-chlorobenzyl alcohol and cinnamyl alcohol were oxidized. The results are summarized in Table 5. For benzyl alcohols with p-methoxy, m-methoxy, 3,4-dimethoxy and 3,4,5-trimethoxy groups high conversions and selectivities could be easily obtained (Table 5, entries 1–5). While benzyl alcohols with p-Cl and m-Cl groups gave a mixture of corresponding aldehyde and carboxylic acid (Table 5, entries 6–7).
Table 5 MNPs-Laccase catalyzed aerobic oxidation of benzylic/allylic alcohols in presence of TEMPOa
The formation of carboxylic acids from p-chloro and m-chloro benzyl alcohols is probably due to stereoelectronic effects exerted by the chlorine substituent on the oxoammonium ion intermediate.33 Cinnamyl alcohol as a model for allylic alcohol can be transformed into the corresponding aldehyde under the same reaction as well (Table 5, entry 8).
On the basis of the previously reported mechanism for the aerobic oxidation of alcohols in the presence of homogenous laccase/TEMPO system23,34 and our study about electrochemical behaviors of TEMPO, TEMPO/MNPs-Laccase and TEMPO/MNPs-Laccase/4-methoxybenzyl alcohol in Na–phosphate butter solution (pH 6) at room temperature by cyclic voltammetry (Fig. 3a), the mechanism shown in Fig. 3b is proposed. In this route, the actual oxidant is the oxoammonium ion, easily generated from TEMPO on oxidation by MNPs-Laccase. Following this preliminary oxidation, a nucleophilic attack of the lone-pair of the alcohol onto the TEMPO-oxoammonium ion takes place to form an adduct. Deprotonation of the adduct at the α-C–H benzylic bond, either intra- (from N–O−) or intermolecularly (from the base form of the buffer, i.e. B) gives rise to the carbonylic product and to the reduced form of TEMPO (i.e., N–OH). MNPs-Laccase would regenerate TEMPO from the generated hydroxylamine. Then, either acid-induced disproportionation of TEMPO, or further oxidation of it by MNPs-Laccase, would form the oxoammonium ion once again. The MNPs-Laccase itself is finally oxidized by oxygen; this synthetic procedure represents an oxidation of alcohols by air, catalyzed by the MNPs-Laccase/TEMPO system.
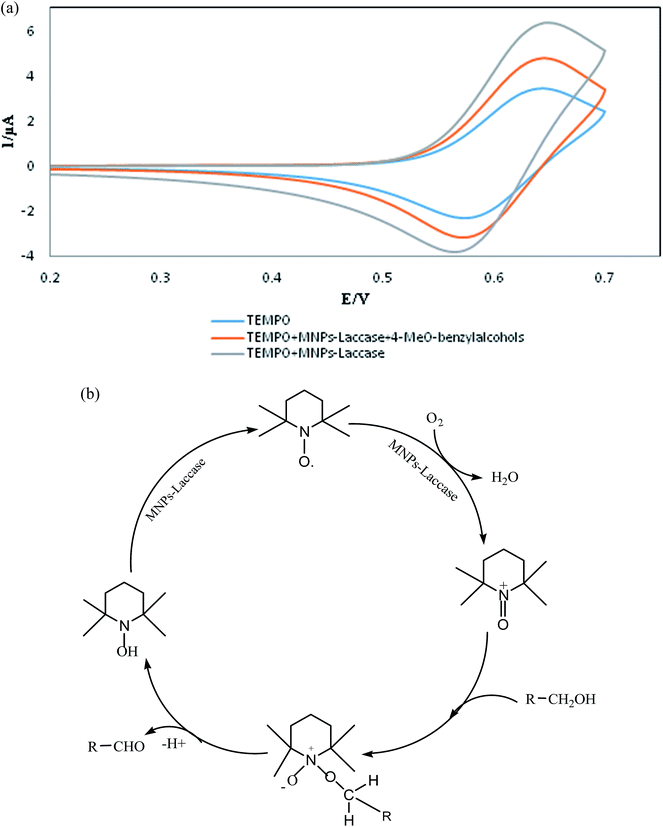 |
| Fig. 3 (a) Cyclic voltammetry of TEMPO, TEMPO/MNPs-Laccase system and TEMPO/MNPs-Laccase/4-methoxybenzyl alcohol system in Na–phosphate butter solution (pH 6) at room temperature, scan rate was 10 mV s−1. (b) Proposed mechanism. | |
2.7. Recovery and reuse of MNPs-Laccase
For practical purposes, it is important that the immobilized laccase system possess not only a high catalytic activity, but also that this activity can be preserved even after multiple cycles. To investigate this issue, we turned our attention to the reusability of MNPs-Laccase. The recovery and reuse of MNPs-Laccase was examined for the aerobic oxidation of 4-methoxybenzyl alcohol in the presence of TEMPO under above mentioned reaction conditions (General procedure). After 24 h, the MNPs-Laccase was easily and rapidly separated from the mixture by exposure to an external magnet and decantation of the reaction solution. The recycled enzyme retained more than 85% of its initial activity after 6 subsequent reaction runs (Fig. 4). The decrease in activity may come from the eventual laccase leakage, desorption of a very small amount of residue laccase adsorbed strongly on the supports, and/or conformational changes in the enzyme tertiary structure during the storage period.
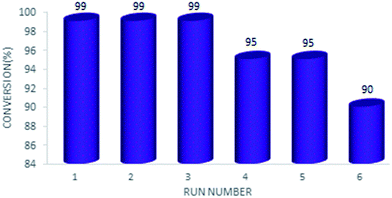 |
| Fig. 4 The recycling experiment of MNPs-Laccase for the aerobic oxidation of 4-methoxybenzyl alcohol (20 mM) using TEMPO at room temperature for 24 h. | |
3. Experimental
3.1. Materials
FeCl2·4H2O, FeCl3·6H2O, tetraethyl orthosilicate (TEOS), 3-aminopropyltrimethoxysilane (APTMS), ammonium hydroxide (25%, w/w), toluene, acetonitrile, ethanol were obtained from Merck (Darmstadt, Germany). 2,2′,6,6′-Tetramethyl-1-piperidinyloxy free radical (TEMPO), 2,2′-azinobis(3-ethylbenzthiazolin-6-sulfonate) (ABTS), glutaraldehyde (25% aqueous solution) was used as bridging agent for the grafting of enzyme to the magnetic support were obtained from Sigma-Aldrich Co. Llc. (St. Louis, America). Laccase (E.C. 1.10.3.2) from Trametes versicolor was also purchased from Sigma and used without further purification. Benzylic and allylic alcohols were distilled before use. Sodium acetate buffer (100 mM) at pH 5 was used for preparing solutions for the activity assay. All other chemicals were of analytical grade and used without further purification.
3.2. Instrumentation
The SEM image was obtained by TESCAN. Weight loss measurements were conducted in a thermal gravimetric analyzer (PerkinElmer Pyris diamond TGDTA) under a N2 atmosphere from 30 °C to 800 °C at a heating rate of 10 °C min−1. The Fourier transform infrared spectroscopy (FT-IR) spectra were obtained with a FT-IR analyzer (Bruker, vector 22). The magnetic measurements were carried out in an Alternating Gradient Force Magnetometer (AGFM, Meghnatis Daghigh Kavir Co., Made in Iran) at room temperature. UV-vis spectra were recorded analyticjena E250 spectrophotometer equipped with a thermostat lauda Ecoline staredition RE 104 and 1 cm quartz cell. The cyclic voltammetry data was obtained with μautolab type 3 equipped with GPES software.
3.3. Large-scale synthesis of Fe3O4 magnetic nanoparticles (denoted as MNPs)
2.147 g of FeCl2·4H2O and 5.835 g of FeCl3·6H2O (molar ratio 1
:
2) were dissolved in 100 mL deionized water under nitrogen protection, the reaction temperature was increased to 80 °C then aqueous ammonia (10 mL, 25%) was added into the solution drop by drop. After being stirred for 30 min, the obtained black precipitate was separated by external magnet and washed several times with deionized water and sodium chloride solution (20 mM). The magnetic nanoparticles were dried under reduced pressure.
3.4. Synthesis of silica-coated magnetic nanoparticles (denoted as SMNPs)
The silica shell onto particles was synthesized via hydrolysis of TEOS in basic solution via Stöber's method with minor modification. Briefly, synthesized magnetite nanoparticles suspended in ethanol (400 mL) and deionized water (100 mL) and sonicated for 30 min, followed by the addition of aqueous ammonia (12.5 mL, 25%). 10 mL of tetraethyl orthosilicate (TEOS) was then added slowly to the reaction solution under mechanical stirring. The resulting dispersion was mechanically stirred for 24 h at room temperature. The SMNPs were collected by magnetic separation and washed several times with ethanol and deionized water, and dried under reduced pressure. The product was stored at 4 °C to use.
3.5. Amination of the silica-coated magnetic nanoparticles (denoted as AMNPs)
10 g of dry SMNPs were dispersed into toluene (250 mL) to produce a homogeneously mixed solution, followed by sonication the mixture for 30 min, followed by the addition of ammonia (100 mL, 25%). Then 30 mL of 3-aminopropyl trimethoxysilane (APTMS) was added into the above solution under mechanical stirring at room temperature for 20 h. The final product was magnetically separated, washed with toluene and 100 mM of Na–phosphate buffer (pH 7.0) several times and dried under reduced pressure.
3.6. Immobilization of laccase on AMNPs (donated as MNPs-Laccase)
The AMNPs (10 g) was stirred at room temperature for 2 h with glutaraldehyde solution (2% v/v glutaraldehyde solution (25%, v/v) in 100 mM Na–phosphate buffer pH 7). Glutaraldehyde-activated AMNPs (GMNPs) was separated by magnetic decantation, extensively washed with Na–phosphate buffer (100 mM pH 7) and dried under reduced pressure. 10 mL of laccase solution (6 U mL−1, 6.52 mg mL−1 in 100 mM Na–phosphate buffer pH 6) was added to the synthesized GMNPs under shaking at 200 rpm for 24 h at 4 °C. Then the product was separated magnetically and washed with the 100 mM of Na–phosphate buffer (pH 7.0) and dried under reduced pressure at 4 °C. The eluate containing residual laccase was collected for the determination of protein simultaneously and the amount of protein in liquid solution was determined by the Bradford method.35
3.7. Activity assays of free laccase and MNPs-Laccase
The activity of the free laccase and MNPs-Laccase was assayed spectrophotometrically with ABTS as substrate (5 mM) in 100 mM Na–acetate buffer (pH 5) by measuring absorbance increase at 420 nm at a temperature of 25 °C. Suitable amount of free laccase or MNPs-Laccase in Na–acetate buffer (100 μL) was added to the mixture and the initial rate was immediately measured as increase in optical density at 420 nm.36 The molar extinction coefficient for the oxidation of ABTS at 420 nm is 3.6 × 104 M−1 cm−1. One unit of activity is defined as the amount of enzyme required to oxidize 1 μmol of ABTS per minute. The activity recovery of the MNPs-Laccase is calculated from the formula: R% = Ai/Af × 100 where R is the activity recovery of the MNPs-Laccase (%), Ai the activity of MNPs-Laccase (U), and Af is the activity of the same amount of free laccase in solution as that immobilized on activated MNPs (U).
3.8. Determination of kinetic parameters of free laccase and MNPs-Laccase
The kinetic parameters, Michaelis constant (Km) and maximum reaction velocity (Vmax), was calculated using Lineweaver–Burk plots plot from the intercepts at x and y axes, respectively by measuring initial rates of the reaction with varying concentration of ABTS ranging 0.3–10.0 mM in Na–phosphate buffer (100 mM, pH 6) at 25 °C. The medium was magnetically stirred for 5 min. The free laccase and MNPs-Laccase were removed and absorbances were measured as the same procedure as for activity assay, using laccase (0.3 mg), MNPs-Laccase (1 mg).
3.9. Thermal and pH dependence
Thermal activities of the free laccase and MNPs-Laccase were tested by measuring the activities after staying in Na–phosphate buffer at pH 6.0 at different temperatures (20–80 °C). The relative activity was calculated as the ratio between the activity at each temperature and the maximum attained. To determine the resistance to pH changes, the activities of the free laccase and MNPs-Laccase were determined by measuring the activities after they were put in different buffer solutions (pH 3–8) at 4 °C for 5 h. The relative activities were determined as the ratio of the obtained activity at the corresponding pH and the maximum attained.
3.10. Storage stability assay
Laccase (0.5 mg of laccase and 1 mg of MNPs-Laccase) was incubated in Na–phosphate buffer 100 mM (pH 6) at 4 °C. At different times (1–30 days), aliquots were taken and the activity was determined by the ABTS method described. For each sample, laccase activity was expressed as relative percentage activity respect to that at time zero.
3.11. General procedure for biocatalytic aerobic oxidation of benzylic and allylic alcohols to corresponding aldehydes
A mixture of benzyl/allyl alcohols (20 mM), MNPs-Laccase (10 U) and TEMPO (6 mM) were added to Na–phosphate buffer (pH 6, 3 mL) containing 4% MeCN that was purged with O2 for 30 min prior to the addition of the catalysts. The mixture was stirred at room temperature for 24 h (Table 5). The catalyst was separated by an external magnet and the mixture was decanted and washed with diethyl ether (2 × 5 mL). The organic solution was dried with anhydrous sodium sulfate and the product yields were determined with GC.
4. Conclusion
In summary, we have synthesized and characterized magnetic core–shell nanoparticle-immobilized laccase from Trametes versicolor (MNPs-Laccase). The thermal, pH and storage stabilities of MNPs-Laccase were effectively improved compared with its free form. Moreover, catalytic efficiency of MNPs-Laccase was made using TEMPO as a mediator for the aerobic oxidation of benzylic/allylic alcohols to the corresponding aldehydes in quantitative yield. The MNPs-Laccase can be easily and quickly recovered from the reaction medium by means of an external magnetic field. More importantly, MNPs-Laccase was stable enough to perform at least six recycling experiments with a conversion higher than 85%.
Acknowledgements
We are grateful to the University of Kurdistan Research Councils for partial support of this work. We are also thankful to Dr R. Shakeri, Department of Biochemistry, University of Kurdistan, for her valuable suggestions.
References
- M. Musawir, P. N. Davey, G. Kelly and I. V. Kozhevnikov, Chem. Commun., 2003, 1414 RSC.
- G. Tojo and M. Fernández, Oxidation of alcohols to aldehydes and ketones, Springer, Berlin, 2006 Search PubMed.
- R. A. Sheldon, I. W. C. E. Arends and A. Dijksman, Catal. Today, 2000, 57, 157 CrossRef CAS.
- J. Muzart, Tetrahedron, 2003, 59, 5789 CrossRef CAS.
- M. Besson and P. Gallezot, Catal. Today, 2000, 57, 127 CrossRef CAS.
- K. Yamaguchi and N. Mizuno, Chem.–Eur. J., 2003, 9, 4353 CrossRef CAS PubMed.
- J. M. Hoover, B. L. Ryland and S. S. Stahl, J. Am. Chem. Soc., 2013, 135, 2357 CrossRef CAS PubMed.
- J. J. Zhu, K. Kailasam, A. Fischer and A. Thomas, ACS Catal., 2011, 1, 342 CrossRef CAS.
- R. D. Schmid and V. B. Urlacher, Modern Biooxidations: Enzymes, Reactions and Applications, Wiley-VCH, Weinheim, 2007 Search PubMed.
- S. R. Couto and J. L. T. Herrera, Biotechnol Adv., 2006, 24, 500 CrossRef PubMed.
- C. O. Fagain, Enzyme Microb. Technol., 2001, 33, 137 Search PubMed.
- S. Davis and R. G. Burns, Appl. Microbiol. Biotechnol., 1992, 37, 474 CrossRef CAS.
- A. Salis, M. Pisano, M. Monduzzi, V. Solinas and E. Sanjust, J. Mol. Catal. B: Enzym., 2009, 58, 175 CrossRef CAS.
- X. Hu, X. Zhao and H. M. Wang, Chemosphere, 2007, 66, 1618 CrossRef CAS PubMed.
- A. D. Annibale, S. R. Stazi and V. J. Vinciguerra, J. Biotechnol., 2000, 77, 265 CrossRef PubMed.
- C. Jolivalt, S. Brenon and E. J. Caminade, J. Membr. Sci., 2000, 180, 103 CrossRef CAS.
- M. Catapane, C. Nicolucci, C. Menale, L. Mita, S. Rossi, D. G. Mita and N. Diano, J. Hazard. Mater., 2013, 249, 337 CrossRef PubMed.
- P. P. Champagne and J. A. Ramsay, Bioresour. Technol., 2010, 101, 2230 CrossRef CAS PubMed.
- L. Shi, F. Ma, Y. Han, X. Zhang and H. Yu, J. Hazard. Mater., 2014, 279, 203 CrossRef CAS PubMed.
- X. Zheng, Q. Wang, Y. Jiang and J. Gao, Ind. Eng. Chem. Res., 2012, 51, 10140 CrossRef CAS.
- E. Solomon, U. M. Sundaram and E. Machonkin, Chem. Rev., 1996, 96, 2563 CrossRef CAS PubMed.
- R. Ten-Have and P. J. M. Teunissen, Chem. Rev., 2001, 101, 3397 CrossRef CAS PubMed.
- M. Fabbrini, C. Galli, P. Gentili and D. Macchitella, Tetrahedron Lett., 2001, 42, 7551 CrossRef CAS.
- A. Rostami, B. Atashkar and D. Moradi, Appl. Catal., A, 2013, 467, 7 CrossRef CAS.
- A. Rostami, Y. Navasi, D. Darvishi and A. Ghorbani-Choghamarani, Catal. Commun., 2014, 43, 16 CrossRef CAS.
- A. Rostami and B. Atashkar, J. Mol. Catal. A: Chem., 2015, 398, 170 CrossRef CAS.
- D. M. Alba, Z. H. Luan and J. Klinowski, J. Phys. Chem., 1996, 100, 2178 CrossRef.
- C. L. Lu, Z. C. Cui, C. Guan, J. Q. Guan, B. Yang and J. C. Shen, Macromol. Mater. Eng., 2003, 288, 717 CrossRef.
- M. Z. Kassaee, H. Masrouri and F. Movahed, Appl. Catal., A, 2011, 395, 28 CrossRef CAS.
- K. Naka, A. Narital, H. Tanakal, Y. Chujo, M. Morita, T. Inubushi, I. Nishimura, J. Hiruta, H. Shibayama, M. Koga, S. Ishibashi, J. Seki, S. Kizaka-Kondoh and M. Hiraoka, Polym. Adv. Technol., 2008, 19, 1421 CrossRef CAS.
- R. C. Ohandley, Modern Magnetic Materials: Principles and Applications, Wiley, New York, 2000 Search PubMed.
- D. Spinelli, E. Fatarella, A. D. Michelea and R. Pognia, Process Biochem., 2013, 48, 218 CrossRef CAS.
- M. Guazzaroni, T. Bozzini and R. Saladino, ChemCatChem, 2012, 4, 1987 CrossRef CAS.
- F. D. Acunzo, P. Baiocco, M. Fabbrini, C. Galli and P. Gentili, Eur. J. Org. Chem., 2002, 4195 CrossRef.
- M. M. Bradford, Anal. Biochem., 1976, 72, 248 CrossRef CAS PubMed.
- B. S. Wolfenden and R. L. Wilson, J. Chem. Soc., Perkin Trans., 1982, 2, 805 RSC.
|
This journal is © The Royal Society of Chemistry 2016 |