DOI:
10.1039/C5RA28091E
(Paper)
RSC Adv., 2016,
6, 42461-42473
Enhancing antimicrobial properties of poly(vinylidene fluoride)/hexafluoropropylene copolymer membrane by electron beam induced grafting of N-vinyl-2-pyrrolidone and iodine immobilization†
Received
30th December 2015
, Accepted 10th April 2016
First published on 11th April 2016
Abstract
Highly efficient antimicrobial properties were imparted to a solution cast poly(vinylidene fluoride)-co-hexafluoropolypropylene (PVDF-co-HFP) NF membrane by modification with povidone–iodine (PVP–I2) complex using electron beam (EB) induced grafting of N-vinyl-2-pyrrolidone (NVP) and subsequent iodine immobilisation. The changes in the morphology of the PVDF-co-HFP membrane in correlation with preparation procedure (NVP grafting and I2 loading) were monitored by a field emission scanning electron microscope (FESEM) whereas, the variation in chemical composition was investigated with FTIR-ATR, UV-vis/NIR and energy dispersive X-ray (EDX). The free surface energy and hydrophilicity/hydrophobicity of the membranes were studied using contact angle measurements. The antimicrobial activity of I2 loaded membrane was evaluated against Gram negative Escherichia coli and Gram positive Staphylococcus aureus in comparison with pristine and PVP grafted membranes. The pure water permeation flux and bacterial suspension filtration through the membranes were also investigated. The use of radiation grafting with EB was found to be highly effective in modification of PVDF-co-HFP membrane by imparting PVP ligands capable of hosting I2 and conferring it very strong antimicrobial activity. Moreover, the obtained membrane is highly promising for water disinfection applications.
1. Introduction
Recently, antimicrobial materials have attained a special focus apart from other materials due to their vital role in several important applications including packing materials, biomedical products, filters of air-conditioning systems, and membranes for water treatment.1–3 Particularly, membranes-based processes such as reverse osmosis and nanofiltration are often used for the production of drinking water, sea water desalination and wastewater treatment. However, membranes employed in these applications are challenged by bio-fouling derived from accumulation of micro-organisms on their surfaces leading to formation of biofilms that cause more than 45% of all membrane fouling.4 Thus, the performance of the membrane system is adversely affected in a way leading to flux reduction, differential pressure increase and feed pressure rise. This is coupled with a reduction in the treated water quality and an increase in the energy consumption as well as cost of operation.
Various methods have been proposed for fouling remediation such as feed pre-treatment of liquids with disinfectant to limit its fouling propensity, improving the antifouling properties of the membrane, cleaning and backwashing of the membrane and finally optimization of the operating conditions. However, treatment with disinfectants including biocide agents such as chlorine was proven to be inefficient or problematic. Particularly, chlorine generates harmful by-products and is unsuitable for most water treatment applications.5
Alternatively, development of antimicrobial membranes by modification of their surface properties during fabrication is an appealing and safe method to render them intrinsically resistant to microbial colonisation and attaining antifouling properties.6 The membrane surface properties that are usually targeted for modification are hydrophilicity, roughness, and charge. Membrane surface modifications can be achieved by blending,7 graft copolymerisation,8 surface coating,9 incorporation of inorganic additives such as nanoparticles of SiO2, Al2O3 or TiO2,10–12 and introduction of antimicrobial additives including silver particles13,14 during the membrane fabrication.
Among all methods, graft copolymerisation is an attractive technique for introducing antimicrobial properties to membranes. This is due to the technique capability to permanently modify substrate polymers with covalently bonded moieties with variety of grafting densities and chain lengths leaving the modified membranes with advanced and novel functions.
Graft copolymerisation can be initiated by various methods such as chemical initiators, plasma, UV light, electron beam (EB) and γ-ray. Of all methods, radiation induced grafting with EB is a convenient method for modifying the surfaces and bulk properties of the membranes (if needed) without altering their inherent properties.15 Particularly, EB induced grafting method relies on formation of radical sites on the polymer backbone by irradiation with electrons and introduction of various desired functional groups onto these sites through reaction with suitable monomers.16 This method has the advantage of reaction simplicity and ability to easily tune grafting level. Besides, the use of EB, which is on/off switch machine shortens the initiation time to few minutes and adds convenience for the large scale production of the membranes.16 Nevertheless, reports on the use of radiation induced grafting for preparation of antimicrobial materials and membranes are scarce in literature.
Poly(N-vinyl-2-pyrrolidone) (PVP) is a biocompatible polymer of low chemical toxicity and high solubility in water and organic solvents. It has weakly basic nature capable of complex formation not only with different anionic reagents such as dyes and surfactants but also with ionic polymers. Besides, PVP imparts hydrophilicity, antimicrobial and antifouling properties when introduced to hydrophobic polymer surfaces. Hence, PVP and its copolymers have been used for making detergents, hydrogels or membranes in chemical, food, pharmaceutical and cosmetics industries. Particularly, PVP was used as a modifier to induce pore formation,17 and antimicrobial as well as antifouling properties18,19 in membranes involved in water treatment. Specifically, the antimicrobial properties of PVP based materials, was enhanced by complexation with iodine,1,3 and this widened their biomedical applications such as dialysis membranes,20 and medical masks.21
The membrane modification with PVP can be carried out by various methods such as blending,22 dip coating,18,23 photo-grafting,1 plasma grafting,24 and γ-rays-induced grafting.25,26 However, membranes modified with dip coating are challenged by leaching of grafted moiety whereas those obtained by UV and plasma take long time in preparation. On the other hand, membranes obtained by radiation induced grafting with γ-rays achieved high degrees of grafting,25,26 but with prolonged reaction and homopolymer removal times. Besides, γ-rays are obtained from radioactive sources (Cs-137 or Co-60) that could pose an environmental hazard.
A number of polymer substrates such as polypropylene (PP),18,25 polyethylene (PE),20 polysulfone,17 polyether ether ketone (PEEK),27 and poly(vinylidene fluoride) (PVDF),23 were modified with PVP in several occasions. However, there no reports on the use poly(vinylidene fluoride-co-hexafluoropropylene) (PVDF-co-HFP), which is an interesting highly stable copolymer with favourable membrane forming properties suitable for imparting antimicrobial and antifouling properties by radiation induced grafting of NVP. The attractiveness of PVDF-co-HFP as a potential membrane material arises from its low crystallinity, smaller glass transition, higher solubility and greater free volume compared to either pure PVDF or PP, whereas its high stability is due to the incorporation of the fluorinated amorphous phase of HFP into the main constituent VDF blocks.28–37 Such chemical combination made PVDF-co-HFP more hydrophobic than either PVDF or PP,28 and thus PVDF-co-HFP became a potential membrane material for liquid separations.29,30
In the present study, radiation induced grafting of N-vinyl-2-pyrrolidone (NVP) monomer onto solution cast and electron beam (EB) irradiated PVDF-co-HFP film is reported for the first time to prepare antimicrobial membrane containing PVP–I2 after complexation with iodine solution. The use of EB induced grafting is expected to be highly effective in the modification of the surface and bulk properties of this membrane. Besides, it has the advantage of being carried out in a short time and the graft moiety is covalently bonded to membrane matrix rendering the membrane more resistant to ageing by leaching commonly associated with membrane modified with physical methods. The, morphological, chemical, and surface properties of the membranes were examined using FESEM, EDX, FT-IR, UV-vis and contact angle measurements. The effect of iodine immobilisation on the antimicrobial and antifouling properties of the fabricated membranes was also investigated.
2. Experimental
2.1. Materials and reagents
PVDF-co-HFP with an average molecular weight 400
000 g mol−1 was purchased from Sigma-Aldrich Chemical Co. and used to prepare polymer solution. NVP monomer with a purity of ≥97.0%, was obtained from Aldrich and used without any further purification. Solid iodine (R & M chemical company) was used to prepare solution by dissolving in pure (deionised) water (18 mΩ cm) obtained from a water deioniser (Millipore Direct-Q™). Solvents such as N,N-dimethyl acetamide (DMAC), ethanol, methanol, and n-heptane were analytical grade and used as received.
2.2. Fabrication of PVDF-co-HFP membrane
A 17 wt% polymer solution was obtained by dissolving PVDF-co-HFP particles in DMAC at 60 °C for 24 h under continuous stirring until a homogenous polymer solution was obtained after cooling to room temperature. The polymer solution was cast over a glass plate at a room temperature using a casting knife. The cast film was exposed to solvent evaporation for 1 min before it was immersed in a coagulation bath containing water as a non-solvent at room temperature for 1 h. To prevent any possible shrinkage in the membrane, it was immersed in an aqueous methanol solution (50 wt%) for 4 h. Finally, the membrane was dried at room temperature for few days before characterization and storage.
2.3. Modification of PVDF-co-HFP films by EB radiation induced grafting of NVP
The PVDF-co-HFP flat sheet membrane was cut into pieces (6 cm × 6 cm), washed with ethanol, dried at 60 °C in an oven for 24 h and weighed. The membrane was placed in a thin PE plastic bag, which was thermally sealed under vacuum. The membrane was irradiated by an EB at 28 °C with an accelerating voltage of 1 MeV at a beam current of 1 mA. The total dose received by the membrane was 100 kGy at a 10 kGy dose per pass. The irradiated membrane was stored in an ultra-cool freezer at a temperature of −80 °C prior to grafting reaction.
A grafting solution composed of 50 vol% NVP in deionised water was placed in a glass ampoule, which was deaerated by bubbling with N2 for 30 min. The monomer solution was transferred immediately to the irradiated PVDF-co-HFP membrane placed in an evacuated glass ampoule through a vacuum stopcock, which was subsequently sealed. The ampoule was placed in a thermostatic bath at a temperature of 60 °C for 8 h to allow the reaction. After completion of the reaction, the membrane was removed and washed several times with methanol/deionised water mixture for 16 h to remove the excess monomer and homopolymer occluded on the membrane surfaces. The membrane was vacuum dried at 60 °C for 24 h. The degree of grafting (DOG%) was gravimetrically determined as a percentage of the weight increase in PVDF-co-HFP membrane after grafting using the following equation:
|
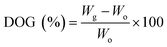 | (1) |
where,
Wo and
Wg are the weights of the pristine and grafted PVDF-
co-HFP membrane, respectively.
2.4. Immobilisation of iodine on PVP grafted films
The PVP grafted PVDF-co-HFP membrane of known weight was immersed in a 10 wt% iodine solution, brought to a temperature of 60 °C and continuously stirred for 12 h under reflux. The membrane was removed, washed with n-heptane several times to remove the free iodine and soaked in fresh n-heptane for 24 h. The membrane sample was washed with an excess of deionised water few times and dried in a vacuum oven at a temperature of 60 °C for 16 h. The sample was eventually weighted and the iodine loading (I2L) in g g−1 was calculated using eqn (2). |
 | (2) |
where, Wd and Wg are the weights of the iodine doped PVP grafted membrane (PVDF-co-HFP/PVP–I2) and the corresponding grafted membrane (PVDF-co-HFP/PVP), respectively. The iodine density (ρI2) in mmol g−1 was obtained from the following equation: |
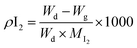 | (3) |
where, MI2 is the molar mass of iodine which equals 253.8 g mol−1. The obtained membrane is called the povidone–iodine immobilized membrane.
2.5. Testing anti-bacterial activity of membranes
The colony of Escherichia coli (E. coli) Gram negative bacterium was used as a reference to determine the concentration of the original number of cells by counting the colony forming unit (CFU). The total CFU was measured by a spread plate method with a standard serial dilution. The colony of E. coli was streaked out and grown on an agar plate and incubated at 37 °C for 18–24 h. The activated E. coli was inoculated into a 20 mL nutrient broth culture. The broth culture was placed in an incubator shaker (News Brunswicks Innova 42) at 37 °C with 300 rpm for 14–16 h. A 1 mL of original bacteria cell suspension was taken and diluted serially (10−1 to 10−7) with a sterile phosphate buffered saline (PBS) of pH 7.2. A 0.2 mL of cell suspension from each diluted value was taken and spread on nutrient agar plates. The agar plates were incubated at 37 °C for 24 h. The concentration of E. coli were measured by manually counted the number of colonies. The reliable result was found at diluted value of 10−6 and was estimated to be 4.3 × 108 CFU mL−1 of original cell (colony) suspension. The number of bacteria per mL of diluted sample was determined using the following equation: |
 | (4) |
Several sterile test tubes containing 10 mL original cell suspension of E. coli with concentrations of 108 CFU mL−1 were prepared and incubated at 37 °C for 24 h. Thereafter, the pristine PVDF-co-HFP, grafted PVDF-co-HFP/PVP, and PVDF-co-HFP/PVP–I2 membranes with dimensions (1 × 3 cm2) were introduced to the test tubes. The membrane having PVP–I2 complex was contacted with original cell suspension incubated at 37 °C for different time periods under continuous shaking at 300 rpm. The concentration (CFU mL−1) of viable cells on the membranes was determined by the spread plate method for all membranes with reference to control E. coli colonies.
2.6. SEM analysis
The morphology of pristine PVDF-co-HFP, grafted PVDF-co-HFP/PVP, and PVDF-co-HFP/PVP–I2 membranes was investigated using FESEM measurements taken on a MERLIN, ZEISS-GEMINI2 microscope equipped with X-ray dispersion analyser (EDX). The samples were fractured after dipping in a liquid nitrogen and dried under vacuum for 30 minutes prior to mounting on the sample holders and sputtering with platinum. The pore size analysis was performed on the membranes after PVP grafting and I2 immobilisation.
2.7. FTIR-ATR analysis
The chemical composition changes in PVDF-co-HFP after NVP grafting and subsequent iodine loading was monitored using Fourier transform infrared (FTIR) spectroscopy equipped with attenuated total reflection (ATR). The measurements were made on a Perkin Elmer spectrophotometer (Spectrum 400 FT-IR/FT-NIR). The spectra were measured in a wave number range of 4000–650 cm−1 at a transmittance mode with a number of scans of 16 at a resolution of 4 cm−1.
2.8. UV-vis/NIR analysis
The formation of iodine complex in the membrane was detected using a Perkin Elmer UV-vis/NIR spectrometer (Lambda 950) equipped with a diffuse reflectance 60 mm integrating sphere at a wavelength range of 200–700 nm.
2.9. EDX analysis
EDX analysis was performed with FESEM measurement during 50 s on a wide surface of the membrane samples. A 400 magnification of the sample was arbitrarily selected (corresponding to a 198 μm × 198 μm) and the whole picture was analysed.
2.10. Contact angle measurements, surface free energy and solubility parameter
The test liquids analysed were double-distilled water (W), glycerol (G) and diiodomethane (DM, 99% purity, Sigma-Aldrich). Advancing contact angles (θ) of drops of the liquids were measured at 20 °C using a contact angle meter CAM 200 (KSV Instruments Ltd., Helsinki, Finland). Drops of each liquid were deposited on the top surface of each membrane sample (5 to 6 repetitions registering a total of 25 to 30 values for each membrane sample) with a manual dosing system holding a 1 mL syringe (0.5 mm diameter needle). Side view images of the drops were captured at a rate of 10 frames per s. Contact angles were automatically calculated by fitting the captured drop shape to the one calculated from the Young–Laplace equation.
Since the total surface free energy (γs) cannot be calculated directly,38 different indirect methods have been proposed, which based on the contact angle measurement of either 2 or 3 different liquids deposited onto a solid surface.39 In the present study, the 3 liquids method was considered.
According to the Lifshitz–van der Waals–acid–base method, or van Oss et al. method40–42 γ can be divided into the following components:
|
 | (5) |
where, i denotes either solid or liquid phase and the acid–base component (
γAB) breaks down into electron-donor (
γ−) and electron-acceptor (
γ+) interactions. For a solid–liquid system, the following expression is given:
41,42 |
2(γLWsγLWl)1/2 + 2(γ+sγ−l)1/2 + 2(γ−sγ+l)1/2 = γl(1 + cos θ)
| (6) |
where, the three surface tension components (
γLWs,
γ+s and
γ−s) together with the surface free energy of the solid measured (
γs) can be obtained.
From the total surface tension and surface tension components estimated by the method described above, the solubility parameter (δ) of the membrane surfaces was calculated using the following relation:43
where,
ec (MJ m
−3) is the cohesive energy density, which is related to
γs (mJ m
−2) that is calculated as follows:
|
 | (8) |
2.11. Permeation flux test
The membrane pure water permeation flux was measured on a batch basis using a dead-end stainless-steel stirred cell (Sterlitech Model HP4750, Kent, WA) with an active membrane area of 14.6 cm2 and processing volume of 300 mL of pure (deionised) water. The cell is equipped with a magnetic stirrer and capable of standing different applied pressures up to 69 bar. The pressure was built up with a nitrogen gas obtained from a cylinder connected to the cell. The pressure was maintained at 10 bar for 30 minutes and the pure water was collected within 60 minutes and the flux (J) was calculated using eqn (9): |
 | (9) |
where, Qp is the permeate flow rate (L h−1), A is the membrane active area (m2) and t is the permeate time.
2.12. S. aureus filtration
To test the efficiency of bacterial removal, Gram positive S. aureus bacteria was prepared at a high concentration of 106 CFU mL−1 in an aqueous sterile phosphate buffered saline (PBS) (7.13 g L−1 Na2HPO4·12H2O, and 1.36 g L−1 KH2PO4) of pH 7.2 and used as a feed solution that was filtered through individual membrane samples hosted in the permeation cell. The permeate volume was collected and the residual concentration of bacteria was determined using spread agar plate technique. The percent of bacteria rejection (R%) value was calculated by the following equation: |
 | (10) |
where, Cf and Cp are the concentrations of the feed and permeate solutions, respectively.
2.13. Confirmation of membrane disinfection performance
After filtration of bacteria, sample having a size of 1 × 5 cm2 was taken from the tested membranes (i.e., PVDF-co-HFP, PVDF-co-HFP/PVP, and PVDF-co-HFP/PVP–I2). The three membrane strips were introduced to test tubes containing sterilized PBS solution and incubated at 37 °C with shaking at 300 rpm for 24 h. The concentration of bacteria was determined using spread agar plate technique as discussed before.
3. Results and discussion
The antimicrobial membrane was prepared in 3 steps: (i) fabrication of PVDF-co-HFP membrane, (ii) radiation induced grafting of NVP onto EB irradiated PVDF-co-HFP membrane and (iii) iodine loading on the PVP grafted membrane. A grafted membrane having a degree of grafting of 19.28% was obtained at a monomer concentration of 50 vol% in water and an irradiation dose of 100 kGy, reaction temperature of 60 °C and reaction time of 8 h. The iodine loading was 0.0714 g I2 per g of membrane and the iodine density was 0.28 mmol g−1. The mechanism of radiation induced grafting of NVP onto PVDF-co-HFP membrane and subsequent iodine loading is illustrated in the schematic diagram shown in Fig. 1. The membrane showed an increase in size after grafting with PVP and immobilisation with I2 and this was accompanied by a colour change from white to yellow brownish in the final membrane (as shown in Fig. S1 given in the ESI†).
 |
| Fig. 1 Schematic representation for preparation of antimicrobial membrane by radiation induced grafting of NVP onto PVDF-co-HFP membrane and iodine immobilisation. | |
3.1. Membrane morphological changes
The surface morphology changes in PVDF-co-HFP membranes after grafting and iodine immobilisation are illustrated by FESEM images shown in Fig. 2. The pristine PVDF-co-HFP membrane shows a smooth surface with round nano-sized pores evenly distributed. The grafted PVDF-co-HFP membrane shows some obvious changes in the surface as a result of modification by PVP grafts which are in forms of unevenly distributed grafted layers while retaining majority of nanopores in a distribution pattern similar to the original membrane. The incorporation of iodine in the grafted membrane seems to provoke aggregation of some iodine nanoparticles and this may be due to loading from solution with high I2 concentration. However, the surface retains even pore distribution despite partial blocking of some of these pores by aggregated iodine particles which are randomly scattered on the surface.
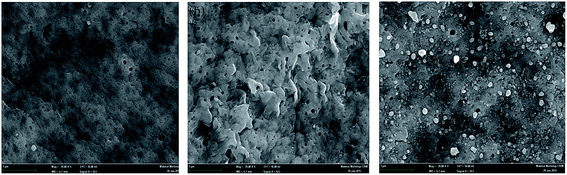 |
| Fig. 2 FESEM images of: (a) pristine PVDF-co-HFP membrane, (b) PVDF-co-HFP/PVP grafted membrane, (c) PVDF-co-HFP/PVP–I2 membrane. | |
The cross-sectional morphology changes in the original PVDF-co-HFP, PVP grafted PVDF-co-HFP and iodine-immobilized membranes are shown in Fig. 3. The original membrane showed a typical asymmetric structure with thin skin and short finger-like pores. The grafted membrane revealed that some PVP chains intruded to the finger-shaped pores along the walls forming grafted layers on the internal surfaces that thicken the pore walls. The iodine treated membrane showed similar invasion of iodine nanoparticles to the pores forming aggregate layers nanoparticles along the walls of the PVP-grafted pore surfaces. These results suggest that PVP grafting took place not only on the surfaces of PVDF-co-HFP membrane but also in its porous structure. These observations confirm the successful incorporation of PVP–I2 complex in the surfaces and pores of PVDF-co-HFP membrane without plugging the latter. The mean pore size of the PVDF-co-HFP film was 74.23 nm (SD + 26.291), which was reduced to 69.66 nm (SD = 16.15) by grafting of PVP and further lowered by I2 immobilization to 59.16 nm (SD = 26.5) as indicated from pore size distribution presented in Fig. S2 given in the ESI.†
 |
| Fig. 3 FESEM morphological cross-section of: (a) original PVDF-co-HFP membrane, (b) PVDF-co-HFP/PVP grafted membrane, (c) PVDF-co-HFP/PVP–I2 membrane. | |
3.2. Membrane chemical changes
Fig. 4 shows FTIR-ATR spectra of prepared PVDF-co-HFP membrane and its PVP grafted counterpart and PVP homopolymer solution as a reference. The PVDF-co-HFP membrane showed characteristic peaks ca. stretching vibrations for CF2 at 1170 cm−1 and CH2 at 2955 cm−1 for PVDF fraction. This was coupled with a stretching vibration at 3050 cm−1 for CH3 neighbouring CH in PP component of the membrane. The PVP grafted membrane showed new absorption peaks at 1663 and 1290 cm−1, which are assigned for C
O and C–N of pyrrolidone ring, respectively. These new features resemble the characteristic peaks shown in the spectrum of pure PVP. Such observations provide a strong evidence for the successful formation of PVP graft copolymer membrane.
 |
| Fig. 4 FTIR-ATR spectra of: (a) original PVDF-co-HFP membrane, (b) PVDF-co-HFP/PVP grafted membrane and (c) liquid PVP homopolymer. | |
The change in the chemical composition on the film surface after grafting of PVP and iodine immobilisation was also monitored with UV-vis/NIR and the obtained spectra are presented in Fig. 5. Compared to the original PVDF-co-HFP membrane and its PVP grafted counterpart, the absorption peak at 291 nm is characteristic for PVP grafted on the membrane surface.44 Moreover, the peak at 366 nm represents the iodine-immobilised confirming the formation of PVP–I2 complex in the membranes.45 The chemical structure of the PVP–I2 complex is strongly believed to be yielded from formation of the hydrogen bond between the tri-iodide ions (H+ I3−) and the oxygen atoms in pyrrolidone groups1 as depicted from the mechanism in Fig. 1.
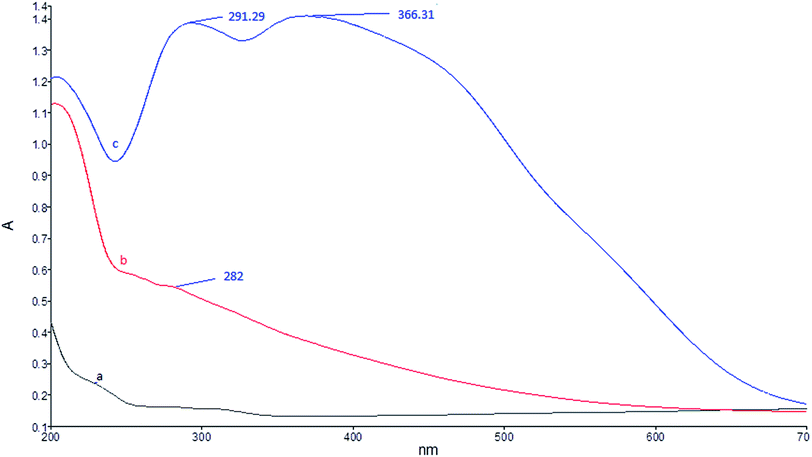 |
| Fig. 5 UV-vis absorption spectra of: (a) original PVDF-co-HFP membrane, (b) PVDF-co-HFP/PVP grafted membrane, (c) PVDF-co-HFP/PVP–I2 membrane. | |
To obtain additional information on the variation in chemical compositions of the membrane caused by PVP grafting and subsequent iodine-immobilisation, EDX analysis was performed and the obtained data is shown in Fig. 6 and Table 1. The associated SEM images are shown in Fig. S3 given in the ESI.† As can be seen, the elements of nitrogen and iodine appeared after PVP grafting and subsequent treatment with iodine. This was coupled with a successive reduction in the fluorine on the surface by grafting and iodine-immobilisation. Both carbon and oxygen increased with PVP grafting and then slightly reduced after iodine treatment. These observations provide further evidence of the efficient NVP grafting and iodine loading (Fig. 6).
 |
| Fig. 6 FESEM-EDX chemical compositions of: (A) original membrane, (B) PVDF-co-HFP/PVP grafted membrane (C) PVDF-co-HFP/PVP–I2 membrane. | |
Table 1 EDX elemental analysis of membrane in different stages
Elements |
Weight (%) PVDF-co-HFP |
Weight (%) PVDF-co-HFP/PVP |
Weight (%) PVDF-co-HFP/PVP–I2 |
C |
42.6 |
48.11 |
44.86 |
N |
— |
0.27 |
— |
O |
2.95 |
5.66 |
4.16 |
F |
54.45 |
45.95 |
40.50 |
I |
— |
— |
10.48 |
Total |
100 |
100 |
100 |
Based on the previous evidences, it can be deduced that irradiation of PVDF-co-HFP membrane with EB led to the formation of active radicals across the membrane. Grafting reaction was initiated when the irradiated membrane was brought into contact with NVP monomer at a temperature (60 °C) that allowed the thermal decomposition of trapped radicals. Grafting took place initially on the surface and then progressively across the membrane as well as inside the pores using the small amount of monomers diffused through nano-porous structure leading to pores with thicker walls. Upon iodine-immobilisation of PVP grafted membrane, the tri-iodide is likely to be formed in the solution in a way binding the partial positive nitrogen and the partial negative oxygen of the pyrrolidone ring by hydrogen bonds forming of PVP–I2 complex on the surfaces and inside the pores of the membrane.
3.3. Hydrophilicity, surface energy, cohesive energy density and solubility parameter
The pristine PVDF-co-HFP, PVDF-co-HFP/PVP grafted and PVDF-co-HFP/PVP–I2 membranes were subjected to contact angle measurements with different liquids and the obtained images are shown in Fig. 7 and the results are summarised in Table 2. For the three membranes, the contact angle values were higher for water than glycerol and diiodomethane, which recorded the lowest values. Moreover, the PVP grafted membrane was found to be more hydrophilic than the povidone-iodine membrane. The incorporation of PVP brings a weak basic moiety and hydrophilic character to the membrane. On contrary, complexation of the grafted PVP with iodine reduced the hydrophilicity of the membrane as a result of fewer interactions with water molecules caused by the involvement of nitrogen and oxygen of the pendant lactam in hydrogen bonds with I2.
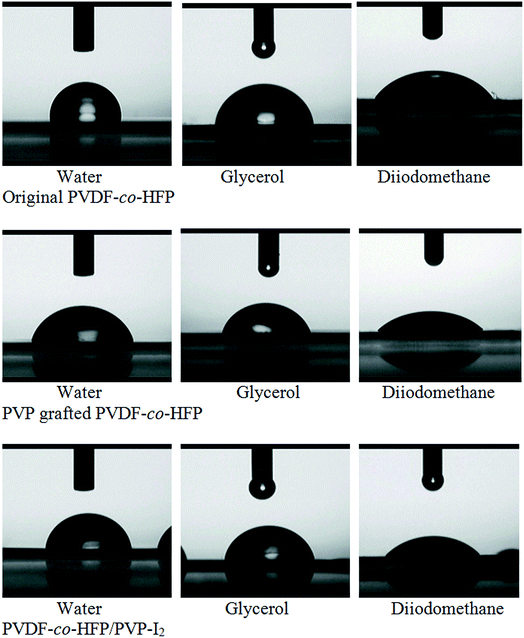 |
| Fig. 7 Contact angle images of original PVDF-co-HFP, PVDF-co-HFP/PVP grafted and PVDF-co-HFP/PVP–I2 membranes with different liquids. | |
Table 2 Contact angles of water (θw), glycerol (θg) and diiodomethane (θd) of the top membrane surface
Membrane samples |
θw (°) |
θg (°) |
θd (°) |
PVDF-co-HFP |
96.19 ± 1.37 |
81.58 ± 1.11 |
58.58 ± 0.19 |
PVDF-co-HFP/PVP |
73.12 ± 0.82 |
68.82 ± 1.50 |
47.19 ± 1.02 |
PVDF-co-HFP/PVP–I2 |
83.78 ± 2.48 |
79.18 ± 1.26 |
47.47 ± 0.79 |
As stated earlier, the surface free energy (γs) and its components (γLWs; γABs, γ+s and γ−s), surface polarity, cohesive energy density (ec) and solubility parameter (δ) of the top membrane surface were calculated from the water contact angles of the three different liquids and the results are presented in Table 3. The contact angles of diiodomethane, for which the LW components of the surface energy dominate, are different for the pristine PVDF-co-HFP and the two modified membranes. Both γLWs and γABs of the pristine membrane are lower than those of the modified membranes. This is mostly due to the electron-donor (γ−s) contribution, which is much smaller for the pristine PVDF-co-HFP membrane. Moreover, γ+s was found to be lower than γ−s in the three membranes. A significant increase in γ−s was detected after modification of PVDF-co-HFP membrane. This is due to the incorporation of PVP and the subsequent immobilisation of I2.
Table 3 Surface free energy (γs) and its components (γLWs; γABs, γ+s and γ−s), surface polarity, cohesive energy density (ec) and solubility parameter (δ) of the top membrane surface calculated by the water contact angles of 3 different liquids according to the Lifshitz–van der Waals/acid–base method or van Oss, Good, and Chaudhury method.40–42
Membrane sample |
γLWs (mJ m−2) |
γ−s (mJ m−2) |
γ+s (mJ m−2) |
γABs (mJ m−2) |
γs (mJ m−2) |
Polarity (%) |
ec (MJ m−3) |
δ (MJ 1/2 m−3/2) |
PVDF-co-HFP |
28.23 |
1.06 |
0.24 |
1.01 |
29.24 |
3.44 |
243.42 |
15.60 |
PVDF-co-HFP/PVP |
30.39 |
20.24 |
0.09 |
2.65 |
33.05 |
8.03 |
292.50 |
17.10 |
PVDF-co-HFP/PVP–I2 |
31.02 |
14.76 |
1.01 |
7.70 |
38.72 |
19.90 |
370.97 |
19.26 |
Other than the hydrophilicity, the polarity of the modified PVDF-co-HFP membranes was found to be enhanced after PVP grafting and increased further after immobilisation of I2 following the order: PVDF-co-HFP < PVDF-co-HFP/PVP < PVDF-co-HFP/PVP–I2.
For the three membranes γABs was an order of magnitude smaller than γLWs and therefore both the total surface free energy (γs) and γLWs showed the following order: PVDF-co-HFP < PVDF-co-HFP/PVP < PVDF-co-HFP/PVP–I2. In other words, the PVDF-co-HFP/PVP–I2 membrane exhibits the highest total surface free energy. Consequently, the cohesive energy density (ec) and the solubility parameter (δ) follow the same order. Thus, the affinity and the degree of swelling of the membrane top surface layers in a given solvent of known δ value can be predicted based on the solubility parameter approach (i.e. the solubility parameter difference, Δδ).46
3.4. Antimicrobial properties of PVDF-co-HFP/PVP–I2 membrane
The antimicrobial activities of pristine PVDF-co-HFP, PVDF-co-HFP/PVP and PVDF-co-HFP/PVP–I2 against E. coli are shown in Fig. 8 whereas the counts of E. coli on the membranes and the corresponding viable cell% are presented in Table 4. The concentration of E. coli in the suspension was 4.3 × 108 CFU mL−1.
 |
| Fig. 8 Measurement of antimicrobial activity of E. coli of (a) original PVDF-co-HFP, (b) PVDF-co-HFP/PVP (c) PVDF-co-HFP/PVP–I2 membranes. | |
Table 4 The counts of E. coli on original, PVP grafted and iodine loaded membrane samples
Membrane samples |
E. coli counted (CFU mL−1) after 10 min |
V.Ca (%) |
V.C = [(CFUmeasured/CFUcontrol) × 100]. |
PVDF-co-HFP |
4.3 × 108 |
100 |
PVDF-co-HFP/PVP |
3.15 × 108 |
73.26 |
PVDF-co-HFP/PVP–I2 |
0.00 |
0.00 |
The original PVDF-co-HFP membrane showed no change in E. coli concentration i.e. 4.3 × 108 CFU mL−1, which was reduced to 3.15 × 108 CFU mL−1 in the grafted PVDF-co-HFP/PVP membrane. On the other hand, the PVDF-co-HFP/PVP–I2 membrane exhibited an extraordinarily zero E. coli concentration at the same contact time of 10 min as evident from Fig. 8. Moreover, the variation of the contact period in the sequence of 2, 4, 6, 8, and 10 minutes also showed a zero E. coli concentration i.e. no cells were grown on the nutrient agar plates. Based on these results, it can be deduced that the original membrane shows no signs of anti-bacterial activity based on the control reference of E. coli and 100% of V.C. Unlikely, the V.C of the grafted membrane was 73.26% and this means that grafting of poly-PVP has introduced a partial anti-bacterial activity against E. coli. Interestingly, the V.C reached 0% by loading the iodine to PVP grafted membrane and this indicates that all E. coli cells were killed once touched PVDF-co-HFP/PVP–I2 membrane. This confirms that the povidone–iodine complex membrane has an excellent anti-microbial activity against Gram negative E. coli. This behaviour can be attributed to sterilisation of the membrane with iodine species such as IO−, I−, and I3−, which are available in the aqueous media and penetrate into the bacterial cell leading halogen-induced denaturation, deformation, and disfigure of protein. Similar observation was made for a polypropylene (PP) film modified by polydopamine and PVP–I2,18 PP modified by photo-grafting of NVP followed by iodine loading1 and micro-sized silica gel particles modified with PVP–I2.3
3.5. Pure water flux
Fig. 9 shows the pure water flux for PVDF-co-HFP, PVDF-co-HFP/PVP, and PVDF-co-HFP/PVP–I2 membranes at same pressure (10 bar). The PVDF-co-HFP membrane showed water flux of 105 L m−2 h, which was remarkably and unexpectedly increased to 210 L m−2 h. Similarly, the pure water flux of the I2 containing membrane was increased but to lesser extent (125 L m−2 h). Particularly, the decrease in the mean pore size of pristine PVDF-co-HFP membrane from 74.23 nm (SD = 26.31) to 69.66 nm (SD = 16.15) and 59.16 nm (SD = 26.5) by respective incorporation of PVP grafts and immobilision of I2 was expected to lower the pure water flux. Such unexpected higher flux behaviour indicates that the chemical changes took place in the membranes dominated the variation in the pore size. This unusual behaviour may be attributed to the increase in the hydrophilicity imparted to the membrane by incorporation of PVP grafts that was further lowered by immobilisation of I2 inside the pores (as depicted from SEM images in Fig. 2) of the membranes as a result of complexation of I2 with N and O of pyrrolidone rings. These observations are in harmony with contact angles (θw) results for the same three membranes stipulated in Table 2, which showed that θw was reduced from 96.19° to 73.12° after PVP grafting and then increased to 83.78 after iodine treatment. Thus, it can be suggested that an imparted water transport mechanism was introduced to the modified membranes through diffusive water flux associated with the water activity change in the hydrophilic volume fraction. This is coupled with the convective flow from the applied hydrostatic water pressure that is similar to pristine membrane. Thus the water transport is mostly determined by portioning between these two types of water flow rather than the small variation in pore size.
 |
| Fig. 9 Pure water flux in different membranes at constant pressure of 10 bar. | |
3.6. Bacteria rejection
The feed solution of S. aureus with concentration of 106 CFU mL−1 (that is similar to the sample taken from domestic wastewater) was calculated using a serial dilution of 10−4 and filtered through the three membranes and the obtained microbial activities are presented in Fig. 10A–D. As can be seen, the initial feed solution showed a high count of bacterial colonies, which was greatly reduced in the permeate obtained from PVDF-co-HFP and PVDF-co-HFP/PVP membranes while completely removed after filtrated through PVDF-co-HFP/PVP–I2 membrane. This observation provides a strong evidence for presence of bacterial rejection. Table 5 presents the rejection rate (R) of the three membranes. For instance, PVDF-co-HFP membrane was able to reject the bacteria colonies by 85%, which was increased to 96% by the incorporation of PVP grafts into the membrane. On the other hand, immobilisation of I2 in the grafted membrane led to a 100% rejection and a complete bacterial removal. The bacterial rejection observed in these membranes, which have 10-folds smaller nominal pore size than S. aureus size (600 nm) can be generally attributed to size exclusion. However, the variation in the level of bacterial rejection suggests that the rejection was also affected by diffusion-controlled mass transfer depending on the type of chemical modifications and their impacts on the membranes dynamics during the test. The complete rejection of S. aureus in the third membrane was affected by denaturation and distortion of bacteria's cells by the presence of I2. However, such finding is not unusual to membranes behaviour in many biotechnology applications, where membranes with smaller pore sizes outperform those with bigger pore sizes (Robert 2010). It can be concluded that the reduction in the bacterial colonies in the permeate solution in all the membranes is caused by nanofiltration, whereas it additionally involved a complete disinfection in I2 containing membrane.
 |
| Fig. 10 Measurement of antimicrobial activity of S. aureus in: (A) initial feed solution and permeate solutions obtained from (B) PVDF-co-HFP membrane, (C) PVDF-co-HFP/PVP and (D) PVDF-co-HFP/PVP–I2 membranes. | |
Table 5 Rejection rate of S. aureus through different membranes
Membrane samples |
R (%) |
PVDF-co-HFP |
85 |
PVDF-co-HFP/PVP |
96 |
PVDF-co-HFP/PVP–I2 |
100 |
3.7. Confirmation of disinfection performance and antifouling properties
The antimicrobial effect of PVDF-co-HFP/PVP–I2 membrane against S. aureus was investigated in comparison with PVDF-co-HFP membrane. The original membrane was found to contain cells of S. aureus, while I2 immobilised membrane was totally free from any bacteria cells as evident from Fig. 11. This confirms that the I2-containing membrane obtained in this study has strong antimicrobial properties.
 |
| Fig. 11 Measurement of antimicrobial activity examined after filtration process through: (A) PVDF-co-HFP and (B) PVDF-co-HFP/PVP–I2 membranes. | |
To further confirm the antifouling properties of I2-containing membrane, the pure water permeate flux obtained after filtration of S. aureus suspension having a concentration of 106 CFU mL−1 was investigated in the three membranes under a constant pressure of 10 bar and the results are presented in Fig. 12. As can be noticed, the water permeation flux through PVDF-co-HFP/PVP–I2 membrane showed the highest value compared to pristine and grafted membranes. Moreover, the water permeation flux of original and grafted membranes was remarkably lower compared to that of pure water under the same pressure as shown in Fig. 11. The high hydrophobic nature of the pristine PVDF-co-HFP membrane seemly promoted fouling with bacteria leading to sever reduction in the pure water flux. Similarly, the grafted membrane was also subjected to fouling effect but to lesser extent due to the hydrophilicity imparted by PVP grafts. This observation is going along with literature, which previously suggested that bacteria adhere better to both hydrophobic and highly hydrophilic charged surfaces.47 On contrary, the presence of I2 in PVDF-co-HFP/PVP–I2 membrane prevented the deposition and the adhesion of the bacteria to the surfaces and consequently inhibited the subsequent biofilm formation. Interestingly, this trend compensated for the reduction in hydrophilicity of the I2 containing membrane and allowed higher water flux than the grafted membrane. Therefore, the biofouling was substantially eliminated and the membrane attained excellent antifouling properties suitable for application in water disinfection.
 |
| Fig. 12 Pure water flux after filtration of S. aureus suspension in different membranes at constant pressure of 10 bar. | |
4. Conclusions
An antimicrobial membrane containing surface and core modified with PVP–I2 complex was fabricated by EB induced grafting of NVP on a solution cast and EB-irradiated PVDF-co-HFP membrane followed by iodine loading. The membrane was successfully grafted with PVP as confirmed by FTIR and 19.28% DOG was attained. UV-vis and EDX proved that iodine was complexed in the subsequent treatment. The iodine loading density reached a value of 0.28 mmol g−1 of the membrane. An even iodine immobilisation was achieved not only on the surface but also inside the membrane nano-pores as revealed by SEM analysis. The contact angle measurements showed that the hydrophilicity of the membrane changed in the order: PVDF-co-HFP < PVDF-co-HFP/PVP–I2 < PVDF-co-HFP/PVP. Other related properties such as polarity, total surface energy, cohesive energy density and solubility parameter were also found to follow the order: PVDF-co-HFP < PVDF-co-HFP/PVP < PVDF-co-HFP/PVP–I2. The antimicrobial activity of the PVDF-co-HFP/PVP–I2 membrane against E. coli was investigated and showed a complete inhibition of the bacterial growth (100% bacteria killing) in a very short time (2 min). The pure water permeation flux was enhanced by incorporation of hydrophilic PVP grafts in the pristine membrane and I2 loading in the grafted membrane with such enhancement was remarkably higher in the former than the latter. The antimicrobial activity investigation on the surfaces of the used membranes showed that the I2-containing membrane has excellent antifouling properties. The presence of disinfectant I2 in membrane rendered its surface resistant to bacteria deposition and thus biofouling was substantially eliminated. It can be concluded that the membrane prepared in this study is a promising candidate in disinfection and biofouling resistance during water treatment. Moreover, EB induced grafting of NVP used in this study is a highly effective method in imparting hydrophilicity and antimicrobial properties to strongly hydrophobic polymers such as PVDF-co-HFP membranes.
Acknowledgements
The authors from Universiti Teknologi Malaysia (UTM) wish to acknowledge the financial support from the Research University fund (Grant # 09H46). A. I. Shawky wishes to acknowledge the financial support provided by Malaysia-Japan International Institute of Technology (MJIIT).
References
- C. M. Xing, J. P. Deng and W. T. Yang, J. Appl. Polym. Sci., 2005, 97, 2026–2031 CrossRef CAS.
- K. Fujiwara, Nucl. Instrum. Methods Phys. Res., Sect. B, 2007, 265, 150–155 CrossRef CAS.
- B. Gao, Z. Wang, Q. Liu and R. Du, Colloids Surf., B, 2010, 79, 446–451 CrossRef CAS PubMed.
- R. Komlenic, Filtrat. Separ., 2010, 47, 26–28 CrossRef.
- T. Nguyen, F. A. Roddick and L. Fan, Membranes, 2012, 2, 804–840 CrossRef CAS PubMed.
- W. Sun, J. Liu, H. Chu and B. Dong, Membranes, 2013, 3, 226–241 CrossRef CAS PubMed.
- J.-H. Kim and K.-H. Lee, J. Membr. Sci., 1998, 138, 153–163 CrossRef CAS.
- B. Van der Bruggen, J. Appl. Polym. Sci., 2009, 114, 630–642 CrossRef CAS.
- M. S. Rahaman, H. Therien-Aubin, M. Ben-Sasson, C. K. Ober, M. Nielsen and M. Elimelech, J. Mater. Chem. B, 2014, 2, 1724–1732 RSC.
- M. Khayet, J. P. G. Villaluenga, J. L. Valentin, M. A. López-Manchado, J. I. Mengual and B. Seoane, Polymer, 2005, 46, 9881–9891 CrossRef CAS.
- T.-H. Bae and T.-M. Tak, J. Membr. Sci., 2005, 249, 1–8 CrossRef CAS.
- L. Yan, Y. S. Li and C. B. Xiang, Polymer, 2005, 46, 7701–7706 CrossRef CAS.
- A. Dror-Ehre, H. Mamane, T. Belenkova, G. Markovich and A. Adin, J. Colloid Interface Sci., 2009, 339, 521–526 CrossRef CAS PubMed.
- K. Zodrow, L. Brunet, S. Mahendra, D. Li, A. Zhang, Q. Li and P. J. J. Alvarez, Water Res., 2009, 43, 715–723 CrossRef CAS PubMed.
- M. M. Nasef and E.-S. A. Hegazy, Prog. Polym. Sci., 2004, 29, 499–561 CrossRef CAS.
- M. M. Nasef and O. Güven, Prog. Polym. Sci., 2012, 37, 1597–1656 CrossRef CAS.
- B. Vatsha, J. C. Ngila and R. M. Moutloali, Phys. Chem. Earth, 2014, 67–69, 125–131 CrossRef.
- J. Jiang, L. Zhu, H. Zhang, B. Zhu and Y. Xu, ACS Appl. Mater. Interfaces, 2013, 5, 12895–12904 CAS.
- N. Riyasudheen, M. J. Paul and A. Sujith, Chem. Eng. Technol., 2014, 37, 1021–1029 CrossRef CAS.
- N. A. Maziad, F. Z. Mahmoud, A. A. Hassan and A. A. Taha, Polym.-Plast. Technol. Eng., 2005, 44, 961–979 CrossRef CAS.
- S. Aoki, K. Fujiwara, T. Sugo and K. Suzuki, Radiat. Phys. Chem., 2013, 84, 242–245 CrossRef CAS.
- P. Wang, J. Ma, Z. Wang, F. Shi and Q. Liu, Langmuir, 2012, 28, 4776–4786 CrossRef CAS PubMed.
- Q. Bi, Q. Li, Y. Tian, Y. Lin and X. Wang, J. Appl. Polym. Sci., 2013, 127, 394–401 CrossRef CAS.
- H. Chen and G. Belfort, J. Appl. Polym. Sci., 1999, 72, 1699–1711 CrossRef CAS.
- T. Sehgal and S. Rattan, Indian J. Pure Appl. Phys., 2010, 48, 823–829 CAS.
- Z.-M. Liu, Z.-K. Xu, J.-Q. Wang, J. Wu and J.-J. Fu, Eur. Polym. J., 2004, 40, 2077–2087 CrossRef CAS.
- S. Zereshki, A. Figoli, S. S. Madaeni, S. Simone, M. Esmailinezhad and E. Drioli, Sep. Purif. Technol., 2010, 75, 257–265 CrossRef CAS.
- C. Feng, R. Wang, B. Shi, G. Li and Y. Wu, J. Membr. Sci., 2006, 277, 55–64 CrossRef CAS.
- M. C. García-Payo, M. Essalhi and M. Khayet, Desalination, 2009, 245, 469–473 CrossRef.
- M. C. García-Payo, M. Essalhi and M. Khayet, J. Membr. Sci., 2010, 347, 209–219 CrossRef.
- A. Manuel Stephan and D. Teeters, Electrochim. Acta, 2003, 48, 2143–2148 CrossRef CAS.
- N. T. K. Sundaram and A. Subramania, J. Membr. Sci., 2007, 289, 1–6 CrossRef CAS.
- A. Manuel Stephan, N. G. Renganathan, S. Gopukumar and T. Dale, Mater. Chem. Phys., 2004, 85, 6–11 CrossRef.
- J.-H. Cao, B.-K. Zhu and Y.-Y. Xu, J. Membr. Sci., 2006, 281, 446–453 CrossRef CAS.
- Y. J. Hwang, S. K. Jeong, K. S. Nahm and A. Manuel Stephan, Eur. Polym. J., 2007, 43, 65–71 CrossRef CAS.
- G. C. Li, P. Zhang, H. P. Zhang, L. C. Yang and Y. P. Wu, Electrochem. Commun., 2008, 10, 1883–1885 CrossRef CAS.
- X. Tian and X. Jiang, J. Hazard. Mater., 2008, 153, 128–135 CrossRef CAS PubMed.
- M. Khayet, C. Y. Feng and T. Matsuura, J. Membr. Sci., 2003, 213, 159–180 CrossRef CAS.
- B. Jańczuk and E. Chibowski, J. Colloid Interface Sci., 1983, 95, 268–270 CrossRef.
- C. J. Van Oss, R. J. Good and M. K. Chaudhury, J. Colloid Interface Sci., 1986, 111, 378–390 CrossRef CAS.
- C. J. van Oss, M. K. Chaudhury and R. J. Good, Adv. Colloid Interface Sci., 1987, 28, 35–64 CrossRef CAS PubMed.
- C. J. Van Oss, M. K. Chaudhury and R. J. Good, Chem. Rev., 1988, 88, 927–941 CrossRef CAS.
- M. Khayet, G. Chowdhury and T. Matsuura, AIChE J., 2002, 48, 2833–2843 CrossRef CAS.
- J. Wan, Z. Ma, M. Han, J. Hong and G. Wang, Solid State Commun., 2002, 121, 251–256 CrossRef CAS.
- P. R. Bhagat, A. K. Pandey, R. Acharya, A. G. C. Nair, N. S. Rajurkar and A. V. R. Reddy, Talanta, 2008, 74, 1313–1320 CrossRef CAS PubMed.
- S. Mandal and V. G. Pangarkar, J. Membr. Sci., 2002, 201, 175–190 CrossRef CAS.
- R. Bernstein, S. Belfer and V. Freger, Environ. Sci. Technol., 2011, 45, 5973–5980 CrossRef CAS PubMed.
Footnote |
† Electronic supplementary information (ESI) available. See DOI: 10.1039/c5ra28091e |
|
This journal is © The Royal Society of Chemistry 2016 |
Click here to see how this site uses Cookies. View our privacy policy here.