DOI:
10.1039/C5RA27778G
(Paper)
RSC Adv., 2016,
6, 30337-30344
Shape dependent stripping behavior of Au nanoparticles toward arsenic detection: evidence of enhanced sensitivity on the Au (111) facet†
Received
26th December 2015
, Accepted 1st March 2016
First published on 2nd March 2016
Abstract
The Au (111) face has an advantage in As(III) detection, reported in earlier studies through the methods of simulation and computation, but unfortunately there is no experimental evidence. Here, a comparative study of gold cubes (110), octahedra (111), and rhombic dodecahedra (110) toward the detection of arsenic has been reported for the first time. The Au (111) face was found to exhibit the highest sensitivity compared with Au (100) and (110) surfaces in our experimental research. A reasonable mechanism based on the deposition process and binding energies was provided. The deposition process is a multiple-step, multiple-electron electrochemical reaction and the transfer step of the first accepted electron is the rate-determining step. This work presents an important insight into a new route to realize the improvement of sensitivity in the electrochemical sensing of As(III).
1. Introduction
Nanomaterial modified electrodes have been widely used to enhance the sensing performance of detection of As(III), which has an obvious connection with skin pigmentation and thickening, and various types of cancer of the skin, lungs, bladder, kidney, etc.1–5 For example, arsenite oxidase and multiwalled carbon nanotube modified electrodes have been developed to detect As(III) with linearity up to 500 ppb and a detection limit of 1 ppb.6 The selective detection of an ultratrace amount of arsenite (AsO2−, As3+) using a Prussian blue-modified screen-printed electrode has also been reported by Kumar et al.7 Iridium-modified boron-doped diamond electrodes exhibited high catalytic activity toward As(III) oxidation with a detection limit, sensitivity, and linearity of 20 nM (1.5 ppb), 93 nA μM−1 cm−2, and 0.999, respectively.8 The high adsorptivity of Fe3O4 microspheres toward As(III) and the advantages of room temperature ionic liquid (RTIL) were combined. An Fe3O4–RTIL composite modified screen-printed carbon electrode showed a better electrochemical performance than commonly used noble metals.9 Investigations into platinum (Pt) nanoparticle modified glass carbon electrodes (GCE),10 Pt nanotubes,11 silver electrodes,12 and mercury electrodes13 have been conducted to improve analytical performances.
Among these nanomaterials, Au generally provides a more sensitive response toward As(0) oxidation than the other electrode materials studied. Much research has revealed that gold material modified electrode is the key to the square wave anodic stripping voltammetry (SWASV) detection of arsenic. Hua et al.14 determined total arsenic in seawater on a gold fiber electrode (25 μm) using anodic stripping voltammetry (ASV) with a limit of detection (LOD) of 0.2 ppb. Kopanica and Novotný15 performed the determination of As(III) in natural water using a gold disc electrode by differential pulse anodic stripping voltammetry (DPASV) and obtained a LOD of 0.15 ppb. Hamilton and Ellis16 used a gold film electrode to determine As(III) and antimony selectively in electrolytic copper samples and achieved a LOD of 0.56 ppb. Huang et al.17–19 investigated As(III) detection using a gold microelectrode in ground water without interference from copper ions. Compton et al.20 applied an Au (111) electrode to gain insight into the mechanism of arsenic deposition and stripping. In particular, Takeo Ohsaka21 described the selective electrochemical detection of As(III) using a highly sensitive platform based on an Au (111)-like surface by the partial reductive desorption of n-butanethiol (n-BT). A self-assembled monolayer of n-BT was formed previously, which allowed the selective blockage of the Au (100) and Au (110) by n-BT while the Au (111) domain remains bare. The fabricated electrode had a high sensitivity and selectivity to As(III) and can detect As(III) even in the presence of a high concentration of Cu(II) without any interference. The author believed that the exposed Au (111) surface domain of the present electrode played a key role in the detection of As(III). However, the evidence to clarify the formation of the Au (111) surface domain is deficient and Au (100) and Au (110) have been barely compared in this study. Similarly, Raj et al.22 grew gold nanoelectrode ensembles on a polycrystalline Au electrode which was highly sensitive and showed a linear response for As(III) up to 15 ppb. According to this article, the high sensitivity and the selectivity were attributed to the predominant orientation of the Au (111) confirmed by X-ray diffraction (XRD). XRD is not sufficient here to prove the advantage of Au (111) in the detection of As(III). More evidence is needed. Therefore, direct evidence is significant in proving the superiority of the Au (111) surface domain in the detection of As(III).
It has been reported that the electrochemical behavior of Au electrodes exhibits a strong relationship with their crystallographic orientation.21 A single crystal Au (111) electrode with a clean, well-defined and well-ordered surface can give a more definite electrochemical behavior for As(III) detection and is suitable to study the deposition mechanism.23 It is thus interesting to use gold nanocubes (100), octahedra (Oct) (111), and rhombic dodecahedra (RD) (110) exclusively to evaluate how different facets affect the electrochemical behavior. In the present work, we have used Au nanoparticles of different crystallographic orientations to detect As(III) and a reasonable mechanism has been suggested based on the binding energy and deposition process. We would expect that the different shapes of Au nanoparticles would affect the detection of As(III) and a new route to realize improved sensitivity would be provided. As far as we know, this is the first time direct evidence is given through a comparison of gold cubes, octahedra, and rhombic dodecahedra toward the detection of arsenic.
First, nanocrystals were synthesized under almost the same reaction conditions, and thus the surfactant effect on the detection can be reduced. Next, to eliminate any possible surfactant effect, electrochemically active areas (ECAs) were calculated in the H2SO4 solution and potential cycling was also employed. Other experimental parameters were optimized. Finally, under the optimal experimental conditions, Au nanoparticle modified GCEs were applied to detect As(III) using SWASV.
2. Experimental section
2.1. Chemical reagents
All reagents were used as received and were analytical-grade. A 1000 mg L−1 As(III) stock solution was supplied by Shanghai Canspec Scientific Instruments Co., Ltd., (China) and kept in the refrigerator to prevent oxidation and exposure to light. Non-acidified standard solutions with concentrations of 10 mg L−1 As(III) were prepared daily. NaBH4 and HAuCl4 were purchased from Sinopharm Chemical Reagent Co., Ltd., Shanghai, China. Cetyltrimethylammonium bromide (CTAB, A.R.), ascorbic acid (AA, A.R.), sodium hydroxide (NaOH, A.R.), and hydrochloric acid (HCl, A.R.) were obtained from Shanghai Chemicals Co. Ltd. The water used for the experiments was high-purity water (18.2 MΩ cm) produced with Milli-Q apparatus.
2.2. Apparatus
A bare 3 mm Glass Carbon (GC) electrode or Au nanoparticle modified GC electrode served as the working electrode; a Pt wire was the counter electrode versus a saturated Ag/AgCl electrode (ChenHua Instruments Co., Shanghai, China), completing the cell assembly. The solutions were deoxygenated by nitrogen bubbling for 10 min before each determination. All experiments were performed under N2 at room temperature. All measurements were performed with a CHI 660D computer-controlled potentiostat (ChenHua Instruments Co., Shanghai, China). The SEM images were taken using a field-emission scanning electron microscope (FESEM, Quanta 200 FEG, FEI Company, USA). The TEM was performed using a JEM-2010 transmission electron microscope operating at 200 kV (quantitative method: Cliff Lorimer thin ratio section).
2.3. Synthesis of gold nanocubes, octahedra, and rhombic dodecahedra
Au nanoparticles have been synthesised by changing the reduction rate by varying the concentration of the Au precursors and pH value.24 A slow reduction reaction rate leads to low supersaturation and results in the formation of Au Oct, which has the lowest surface energy (111) faces; while a faster reduction rate leads to higher supersaturation and forms Au cubes with higher surface energy (100) faces. The procedure for making Au nanoparticles is described below.
Firstly, a volume of 10 mL of aqueous solution containing HAuCl4 (0.25 mmol L−1) and CTAB (75 mmol L−1) was pre-prepared. Concurrently, 0.6 mL of ice-cold NaBH4 (10 mmol L−1) was rapidly injected to the pre-prepared mixture at 30 °C with vigorous stirring. The resulting solution turned brown a few seconds later, indicating the formation of gold particles. The whole solution was aged for two hours to promote the decomposition of the remaining NaBH4 in the solution. Then, 1 mL of the pre-prepared hydrosol was diluted to 100 mL with water as a seed hydrosol. Secondly, a volume of 25 mL of aqueous solution containing HAuCl4 (0.04 mmol L−1), CTAB (16 mmol L−1) and ascorbic acid (AA, 6 mmol L−1) was prepared. Thirdly, 0.3 mL of the seed hydrosol was added to the mixture immediately with vigorous stirring. Then the reaction mixture was left undisturbed at 30 °C overnight, and a light purple colloidal solution was achieved.
For the synthesis of Au cubes, 0.150 mL of 25 mmol L−1 HAuCl4 (3.75 × 10−3 mmol) was quickly added to 8 mL of the seed solution and mixed with vigorous stirring for 10 seconds and left undisturbed at 30 °C for 2 hours.
For the synthesis of Au octahedra, similarly, 0.015 mL 25 mmol L−1 HAuCl4 was added to 8 mL of the seed solution, and the reaction procedure was repeated 10 times at a reaction interval of half an hour, by which the same total amount of Au (3.75 × 10−3 mmol) would be grown on Au seeds.
For the synthesis of RD nanocrystals, 0.55 mL of NaOH (0.2 mol L−1) was added before adding HAuCl4.
2.4. Fabrication of Au nanoparticle modified GC electrode
The preparation of Au nanoparticles is described above. The corresponding samples were washed twice using centrifugation before characterization. The Au nanoparticles were dispersed into absolute DI water and the total volume was made up to 1 mL. To fabricate the modified electrode, 10 μL of the suspension was pipetted onto the surface of the glass carbon working electrode and evaporated at room temperature to obtain Au nanoparticle modified GCEs.
2.5. Electrochemical measurements
The Au nanoparticle modified GCEs served as the working electrode for arsenic detection with square wave anodic stripping voltammetry (SWASV) under optimized conditions. The as-prepared Au modified GCEs were dipped in a stirred analyte solution of 0.5 M H2SO4 containing As(III) and kept at −0.2 V for 200 s. The anodic stripping was performed from −0.1 to 0.6 V with the following parameters: frequency, 15 Hz; amplitude, 25 mV; increment potential, 4 mV; vs. Ag/AgCl. After each measurement, the modified electrode was regenerated in a freshly stirred supporting electrolyte by desorption at −0.2 V for 120 s to remove the previous residual As(0) from the electrode surface. For comparison, other modified electrodes were used with the same process and conditions.
3. Results and discussion
3.1. Morphologic and structure characterization of Au nanoparticles
Three types of Au polyhedra, including cubes, OCT, and RD, which were bound by three low-index (100), (111) and (110) facets, respectively, are synthesized. The shapes of the three types of gold nanocrystals can be clearly discerned from their scanning electron microscopy (SEM) images (Fig. S1†). It is obvious that the sizes of the three types of Au polyhedra are similar (Fig. S1†) which can be confirmed by the size distribution in Fig. 1. The selective growth of different low-index crystal surfaces for the various shapes of nanocrystals is confirmed by the corresponding TEM images (Fig. 1).
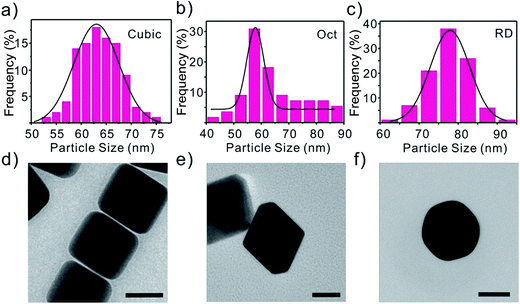 |
| Fig. 1 The particle size distribution of Au NPs: (a) cubes, (b) octahedra, and (c) dodecahedra. TEM of Au polyhedra: (d) cubes, (e) octahedra, and (f) dodecahedra. Scale bars are equal to 50 nm. | |
Furthermore, one can see that all of the three types of Au polyhedra are identical sizes (their edge lengths are around 60–70 nm) with a monodisperse size distribution (Fig. 1). Preparing Au nanocrystals with uniform sizes removes the possible influence of differences in the nanoparticles size on the detection of As(III).25
3.2. Electrochemical characterization of Au nanoparticle modified GCE
The surface modified electrodes are firstly characterized using cyclic voltammetry (CV) (Fig. 2a–c). Although Au nanoparticles are a conductive material, the surface of the nanoparticles would be covered by surface active agent. They could be incorporated into the electrochemical system to hinder the electron exchange between the electrochemical probe and the electrode and result in the impedance conductivity of the matrices. Thus, the anodic and cathodic peaks decreased for the modified electrode compared with the bare GCE. Enhanced impedance spectroscopy (EIS) of 5 mM K3Fe(CN)6 of the modified glassy carbon electrodes were also observed (red line in Fig. 2d–f). This result also indicated that washing Au nanoparticles with clean water is not enough to remove the surface active agent. In EIS, the semicircle diameter of the Nyquist diagram is equal to the surface electron transfer resistance (Ret). The immobilization of Au nanoparticles on the electrode surface can change the Ret value. The obtained result further indicates that the Au nanoparticles have been assembled onto the GCE surface and impede the electron transfer between the electrolyte and the electrode.
 |
| Fig. 2 Cyclic voltammograms (a)–(c) and Nyquist diagrams of electrochemical impedance spectra (d)–(f) for bare, Au nanocube, nanooctahedra and nanorhombic dodecahedra modified GCE and Au nanoparticle modified GCE after 20 cycles of potential cycling in a solution of 5 mM Fe(CN)63−/4− containing 0.1 M KCl. Potential scan rate: 100 mV s−1. The inset shows the equivalent circuit. CAu is a constant-phase element (used here as a nonideal monolayer capacitance of the Au-GCEs), and Rx and C are the resistance and nonlinear capacitor accounting for CTAB in the Au-GCEs. | |
Before comparing the detection sensitivity, the Au nanoparticles were first subjected to the removal of the excess capping agent, CTAB, which would block the active sites on the nanoparticle surfaces.26 After three rounds of centrifugation at 6500 rpm and washing with pure water, the Au nanoparticle dispersions were concentrated to 1 mL and deposited on the surfaces of GCEs. The GCE modification was followed by potential cycling between −0.20 and 1.50 V versus Ag/AgCl for 20 cycles at 100 mV s−1 in 0.5 M H2SO4 in order to further clean the nanocrystal surfaces.
After 20 cycles of potential cycling, the electrochemical characteristics of all three types of Au nanoparticles gradually became stable and the peak current increased (Fig. 3). From Fig. 3, it is certain that after potential cycling, Au nanoparticles are further cleaned. Because the scan rate is different, the CV curves have slight differences to those shown in Fig. S2.† More interestingly, all three types of Au nanoparticles exhibit distinct electrochemical behaviors (Fig. S2a†). As shown in the enlarged CV curves in the region 1.00–1.50 V, the peak positions have a connection with the formation of a monolayer of oxide for the different Au particles (Fig. S2b†). It is obvious that all of the three CV curves are similar to those of the bulk Au single crystal electrodes previously reported.27 From the CV curves of the Au nanoparticles, the electrochemically active areas (ECAs) can be determined, which are calculated by integrating the charges that are needed to form an oxide monolayer on the gold nanoparticle surfaces.28 According to Fig. S2b,† the ECAs are calculated by integrating the oxide adsorption charges assuming 384 μC cm−2 for cubes, 444 μC cm−2 for Oct and 272 μC cm−2 for RD.29 From the calculated results, we find that the ECAs for cubes are larger than those for Oct and RD. This phenomenon can be explained by the fact that the volume of the cubes is smaller than Oct and RD with the same edge length. When the amount of Au is similar, more nanocubes are formed and lead to a higher ECA. To compare the detection activity of the differently shaped Au nanoparticles, the obtained sensitivity toward As(III) needs to be normalized to the corresponding ECA values.
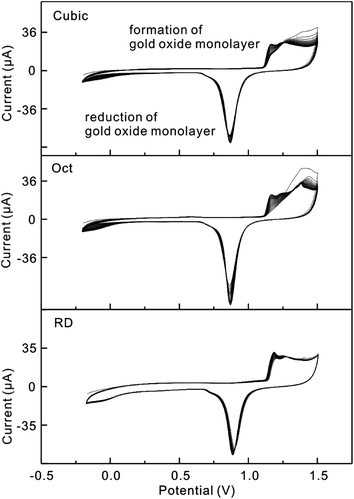 |
| Fig. 3 Cyclic voltammograms of the different Au nanoparticles on glassy carbon electrodes in 0.5 M H2SO4 at a scan rate of 100 mV s−1, 20 cycles. The electrochemical characteristics of all three types of Au nanoparticles gradually became stable and the peak current increased. | |
3.3. Electrochemical detection of arsenic
Prior to discussing the electrochemical performance of the differently shaped Au nanoparticles toward As(III), the experimental parameters (supporting electrolytes, deposition time, and deposition potential) are firstly explored (Fig. 3). Under the optimal experimental conditions, differently shaped Au nanoparticle modified GCEs (Au-GCEs) are applied to the detection of the target As(III) by SWASV.
The electrochemical behavior of Au nanoparticle modified GCEs is investigated. Fig. 4 shows the square wave anodic stripping voltammetry (SWASV) response of Au-GCEs toward As(III) over a concentration range of 10–1000 ppb in 0.5 M H2SO4. Strong and well-defined peaks at 0.2 V vs. Ag/AgCl can be clearly observed, which increase in a linear manner against As(III) concentrations, while there is no obvious peak for GCEs (Fig. S3†). It is clear in Fig. 5a that two significantly different sensitivities exist: one is at low As(III) levels (100–400 ppb) and another is at higher levels (500–1000 ppb). In the first linear region, the response increased rapidly with the increase of As(III) concentration. Further increases lead to the equilibration of the As(III) on the electrode surface with that in the supporting electrolyte. This gradually saturates the adsorption level and does not increase the current peak any more. In fact, this result agreed with the research reported before.30–32 The same phenomenon can be found in the Au RD modified GCEs (Fig. 5c). The reasonable explanation is that only one As(0) monolayer was electrodeposited on the Au electrode in 0.5 M aqueous H2SO4 at −0.2 V, and when the active sites have been occupied by As(III), it is hard to electrodeposit As(III) on the sites again.
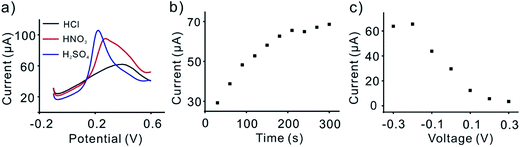 |
| Fig. 4 Optimum experimental conditions. Influence of (a) supporting electrolytes, (b) deposition time, and (c) deposition potential on the voltammetric response of Au nanoparticle modified GCE. Data were evaluated by SWASV of 1 ppm As(III). | |
 |
| Fig. 5 Typical SWASV response of (a) Au nanocube modified GCE, (b) Au octahedra modified GCE, and (c) nanorhombic dodecahedra modified GCE for the analysis of As(III) in different concentration ranges. (d), (e), and (f) are the corresponding linear calibration plots of peak current against As(III) concentrations. The black line refers to the baseline. Supporting electrolyte: 0.5 M H2SO4. Deposition potential, −0.2 V; deposition time, 120 s; amplitude, 25 mV; increment potential, 4 mV; frequency, 15 Hz. | |
Note that in order to exclude the effect of the electrochemically active surface areas of the Au nanoparticles and obtain accurate results, the ECAs of the Au nanoparticles are calculated and have been subtracted (Fig. 6). To make the results easier to compare, one sensitivity exists for one shape of the Au nanoparticles used to modify the GCEs. The obtained sensitivities of Au nanocubes, Au nanoOct and Au nanoRD toward As(III) were all explored with approximate values of 0.0157, 0.4787, and 0.1816 μA ppb−1 cm−2, respectively. A reasonable mechanism based on the binding energies was provided below. The interference of Cu(II) on the detection of As(III) was carefully investigated for the Au modified GCEs. We found that Cu caused a significant interference. This result does not agree well with earlier studies. A reasonable explanation needs further study.21,33,34 Also, there is no comparable superiority in sensitivity. This may result from the surfactant effect, although a lot of effort has been made. Another reason is that the modified Au nanoparticles may have a gap with the GCEs and resist electron transfer compared with electrolytically deposited Au particles. A lot of hard work needs to be done to improve the sensitivity.
 |
| Fig. 6 Plot of sensitivity versus concentration for the detection of arsenic using gold nanocubes, octahedra, and rhombic dodecahedra modified GCEs. | |
4. Reasonable mechanism
Square wave anodic stripping voltammetry (SWASV) is the most promising technique, which consists of a specified period of preconcentration of As(0) produced from the reduction of As(III) deposited on the electrode surface followed by the anodic stripping of As(0).35 There are two stable conformations: (a) the As(III) species in solution binds to the gold by means of the arsenic atom (Au–As mode as shown in Fig. 7) and (b) the As(III) species in solution binds to the gold by means of the oxygen atom (Au–O mode). Due to the high negative charge in the gold atom and oxygen that destabilizes the Au–O bonding, the Au–As mode may play an important part in the Au⋯As(OH)3 interaction.36
 |
| Fig. 7 (a) Top views and (b) side views of the most stable structure of As(OH)3 on Au (111) (left), Au (110) (middle), and Au (100) (right) surfaces. | |
The sensitivity toward the detection of As(III) corresponded to the amount of As(0) deposited. A reasonable mechanism20 is provided as follows:
As(III)(aq) + e− → As(II)(aq) rds |
As(II)(aq) + e− → As(I)(aq) equilibration |
As(I)(aq) + e− → As(0)(ads) equilibration |
The deposition process is a multiple-step, multiple-electron electrochemical reaction and the transfer step of the first accepted electron is the rate-determining step (rds).37 The sensitivity corresponds to the amount of As(0) deposited on the electrode. Thus a longer deposition time leads to a larger amount of As(0) deposited on the Au nanoparticle surface and results in a higher sensitivity. Besides, at −0.2 V on the Au electrode in 0.5 M aqueous H2SO4, As(III) can deposit only one layer on the surface of the Au material electrode.37 Therefore, when the electrode is covered by one As(III) layer, a longer deposition time is no longer efficient to increase the sensitivity. This mechanism agreed well with our experimental result. It can also explain why the sensitivity will decrease, even though the current peak does not increase any more when the concentration of As(III) reaches a certain level.
Meanwhile, differently shaped nanoparticles have different crystal faces which correspond with the binding energy.3,24,38 The binding energies between As(III) and the gold (111), (110), and (100) surface planes reduce gradually.29,38 As(III) needed a lower energy to adsorb onto the Au (111) surface and the electron more easily transported from the Au-GCEs to As(III). Note that the first accepted electron for the As(III) deposition is the rate-determining step, and there is more As(0) deposited on the (111) surface. Under the same experimental conditions, Au Oct (111) has a higher sensitivity than Au RD (110) and Au cubes (100). To clarify this effect, more studies are needed.
5. Conclusion
Direct evidence of the enhanced sensitivity of the Au (111) facet through a comparative study of gold nanocubes, nanooctahedra, and nanorhombic dodecahedra toward the detection of arsenic has been reported. To compare the detection activity of the differently shaped Au nanoparticles, the obtained sensitivity toward As(III) needs to be normalized to the corresponding ECA values. Au octahedra nanoparticles were found to exhibit the highest sensitivity, and then we proposed mechanisms based on binding energies and the deposition process. The Au–As mode plays an important part in the Au⋯As(OH)3 interaction. This mechanism based on the Au deposition process also accounts for the phenomenon that the sensitivity will decrease when the concentration of As(III) reaches a certain level. The electrochemical behavior of Au modified electrodes toward the detection of As(III) exhibits a strong relationship with their crystallographic orientation. A new way might be provided to enhance the sensitivity toward the detection of arsenate.
Acknowledgements
The authors acknowledge the financial support from the National Key Scientific Program-Nanoscience and Nanotechnology (2011CB933700 and 2013CB934300), the National High Technology Research and Development Program of China (863 Program) (2013AA065602), and the National Natural Science Foundation of China (21475133, 21277146, and 61474122).
References
- J. Wei, Z. Guo, X. Chen, D. D. Han, X. K. Wang and X. J. Huang, Ultrasensitive and Ultraselective Impedimetric Detection of Cr(VI) Using Crown Ethers as High-Affinity Targeting Receptors, Anal. Chem., 2015, 87(3), 1991–1998 CrossRef CAS PubMed.
- Z. G. Liu, Y. F. Sun, W. K. Chen, Y. Kong, Z. Jin, X. Chen, X. Zheng, J. H. Liu, X. J. Huang and S. H. Yu, Facet-Dependent Stripping Behavior of Cu2O Microcrystals Toward Lead Ions: A Rational Design for the Determination of Lead Ions, Small, 2015, 11(21), 2493–2498 CrossRef CAS PubMed.
- X. Z. Yao, Z. Guo, Q. H. Yuan, Z. G. Liu, J. H. Liu and X. J. Huang, Exploiting Differential Electrochemical Stripping Behaviors of Fe3O4 Nanocrystals toward Heavy Metal Ions by Crystal Cutting, ACS Appl. Mater. Interfaces, 2014, 6(15), 12203–12213 CAS.
- W. Z. Jia, L. Su and Y. Lei, Pt nanoflower/polyaniline composite nanofibers based urea biosensor, Biosens. Bioelectron., 2011, 30(1), 158–164 CrossRef CAS PubMed.
- H. Xu, L. P. Zeng, S. J. Xing, G. Y. Shi, J. S. Chen, Y. Z. Man and L. T. Jin, Highly ordered platinum-nanotube arrays for oxidative determination of trace arsenic(III), Electrochem. Commun., 2008, 10(12), 1893–1896 CrossRef CAS.
- K. B. Male, S. Hrapovic, J. M. Santini and J. H. T. Luong, Biosensor for Arsenite Using Arsenite Oxidase and Multiwalled Carbon Nanotube Modified Electrodes, Anal. Chem., 2007, 79(20), 7831–7837 CrossRef CAS PubMed.
- J.-M. Zen, P.-Y. Chen and A. S. Kumar, Flow Injection Analysis of an Ultratrace Amount of Arsenite Using a Prussian Blue-Modified Screen-Printed Electrode, Anal. Chem., 2003, 75(21), 6017–6022 CrossRef CAS PubMed.
- T. A. Ivandini, R. Sato, Y. Makide, A. Fujishima and Y. Einaga, Electrochemical Detection of Arsenic(III) Using Iridium-Implanted Boron-Doped Diamond Electrodes, Anal. Chem., 2006, 78(18), 6291–6298 CrossRef CAS PubMed.
- C. Gao, X.-Y. Yu, S.-Q. Xiong, J.-H. Liu and X.-J. Huang, Electrochemical Detection of Arsenic(III) Completely Free from Noble Metal: Fe3O4 Microspheres-Room Temperature Ionic Liquid Composite Showing Better Performance than Gold, Anal. Chem., 2013, 85(5), 2673–2680 CrossRef CAS PubMed.
- S. Sanllorente-Méndez, O. Domínguez-Renedo and M. J. Arcos-Martínez, Determination of Arsenic(III) Using Platinum Nanoparticle-Modified Screen-Printed Carbon-Based Electrodes, Electroanalysis, 2009, 21(3–5), 635–639 CrossRef.
- H. Xu, L. Zeng, S. Xing, G. Shi, J. Chen, Y. Xian and L. Jin, Highly ordered platinum-nanotube arrays for oxidative determination of trace arsenic(III), Electrochem. Commun., 2008, 10(12), 1893–1896 CrossRef CAS.
- A. O. Simm, C. E. Banks and R. G. Compton, The Electrochemical Detection of Arsenic(III) at a Silver Electrode, Electroanalysis, 2005, 17(19), 1727–1733 CrossRef CAS.
- G. Forsberg, J. W. O'Laughlin, R. G. Megargle and S. R. Koirtyihann, Determination of arsenic by anodic stripping voltammetry and differential pulse anodic stripping voltammetry, Anal. Chem., 1975, 47(9), 1586–1592 CrossRef CAS.
- C. Hua, D. Jagner and L. Renman, Automated-Determination of Total Arsenic in Sea-Water by Flow Constant-Current Stripping Analysis with Gold Fiber Electrodes, Anal. Chim. Acta, 1987, 201, 263–268 CrossRef CAS.
- M. Kopanica and L. Novotný, Determination of traces of arsenic(III) by anodic stripping voltammetry in solutions, natural waters and biological material, Anal. Chim. Acta, 1998, 368(3), 211–218 CrossRef CAS.
- T. W. Hamilton, J. Ellis and T. M. Florence, Determination of arsenic and antimony in electrolytic copper by anodic stripping voltammetry at a gold film electrode, Anal. Chim. Acta, 1980, 119(2), 225–233 CrossRef CAS.
- Z. G. Liu, X. Chen, Z. Guo, J. H. Liu and X. J. Huang, Facile Electrodeposition of MoOx onto Gold Microwire Electrode: Application to Voltammetric Determination of As(III) under Mild Conditions, Chem. Lett., 2015, 44(7), 940–942 CrossRef CAS.
- Z. G. Liu, X. Chen, Y. Jia, J. H. Liu and X. J. Huang, Role of Fe(III) in preventing humic interference during As(III) detection on gold electrode: spectroscopic and voltammetric evidence, J. Hazard. Mater., 2014, 267, 153–160 CrossRef CAS PubMed.
- Z. G. Liu, X. Chen, J. H. Liu and X. J. Huang, Robust electrochemical analysis of As(III) integrating with interference tests: a case study in groundwater, J. Hazard. Mater., 2014, 278, 66–74 CrossRef CAS PubMed.
- Z. Jia, A. O. Simm, X. Dai and R. G. Compton, The electrochemical reaction mechanism of arsenic deposition on an Au (111) electrode, J. Electroanal. Chem., 2006, 587(2), 247–253 CrossRef CAS.
- M. R. Rahman, T. Okajima and T. Ohsaka, Selective Detection of As(III) at the Au(III)-like Polycrystalline Gold Electrode, Anal. Chem., 2010, 82(22), 9169–9176 CrossRef CAS PubMed.
- B. K. Jena and C. Retna Raj, Gold Nanoelectrode Ensembles for the Simultaneous Electrochemical Detection of Ultratrace Arsenic, Mercury, and Copper, Anal. Chem., 2008, 80(13), 4836–4844 CrossRef CAS PubMed.
- X. Dai and R. G. Compton, Gold Nanoparticle Modified Electrodes Show a Reduced Interference by Cu(II) in the Detection of As(III) Using Anodic Stripping Voltammetry, Electroanalysis, 2005, 17(14), 1325–1330 CrossRef CAS.
- H. L. Wu, H. R. Tsai, Y. T. Hung, K. U. Lao, C. W. Liao, P. J. Chung, J. S. Huang, I. C. Chen and M. H. Huang, A comparative study of gold nanocubes, octahedra, and rhombic dodecahedra as highly sensitive SERS substrates, Inorg. Chem., 2011, 50(17), 8106–8111 CrossRef CAS PubMed.
- D.-D. Han, Z.-G. Liu, J.-H. Liu and X.-J. Huang, The size effect of Pt nanoparticles: a new route to improve sensitivity in electrochemical detection of As(III), RSC Adv., 2015, 5(48), 38290–38297 RSC.
- C. M. Sánchez-Sánchez, J. Solla-Gullón, F. J. Vidal-Iglesias, A. Aldaz, V. Montiel and E. Herrero, Imaging Structure Sensitive Catalysis on Different Shape-Controlled Platinum Nanoparticles, J. Am. Chem. Soc., 2010, 132(16), 5622–5624 CrossRef PubMed.
- A. Hamelin, Cyclic voltammetry at gold single-crystal surfaces. Part 1. Behaviour at low-index faces, J. Electroanal. Chem., 1996, 407(1–2), 1–11 CrossRef.
- D. Chen, Q. Tao, L. W. Liao, S. X. Liu, Y. X. Chen and S. Ye, Determining the Active Surface Area for Various Platinum Electrodes, Electrocatalysis, 2011, 2(3), 207–219 CrossRef CAS.
- J. Wang, J. Gong, Y. Xiong, J. Yang, Y. Gao, Y. Liu, X. Lu and Z. Tang, Shape-dependent electrocatalytic activity of monodispersed gold nanocrystals toward glucose oxidation, Chem. Commun., 2011, 47(24), 6894–6896 RSC.
- J. C. Ndamanisha and L. P. Guo, Nonenzymatic glucose detection at ordered mesoporous carbon modified electrode, Bioelectrochemistry, 2009, 77(1), 60–63 CrossRef CAS PubMed.
- K. Gibbon-Walsh, P. Salaun and C. M. G. van den Berg, Determination of arsenate in natural pH seawater using a manganese-coated gold microwire electrode, Anal. Chim. Acta, 2012, 710, 50–57 CrossRef CAS PubMed.
- J. F. Huang and H. H. Chen, Gold-nanoparticle-embedded Nafion composite modified on glassy carbon electrode for highly selective detection of arsenic(III), Talanta, 2013, 116, 852–859 CrossRef CAS PubMed.
- B. K. Jena and C. R. Raj, Gold nanoelectrode ensembles for the simultaneous electrochemical detection of ultratrace arsenic, mercury, and copper, Anal. Chem., 2008, 80(13), 4836–4844 CrossRef CAS PubMed.
- G. M. Alves, J. M. Magalhaes, P. Salaun, C. M. van den Berg and H. M. Soares, Simultaneous electrochemical determination of arsenic, copper, lead and mercury in unpolluted fresh waters using a vibrating gold microwire electrode, Anal. Chim. Acta, 2011, 703(1), 1–7 CrossRef CAS PubMed.
- Z. G. Liu and X. J. Huang, Voltammetric determination of inorganic arsenic, TrAC, Trends Anal. Chem., 2014, 60, 25–35 CrossRef CAS.
- D. Cortes-Arriagada, M. P. Oyarzun, L. Sanhueza and A. Toro-Labbe, Binding of Trivalent Arsenic onto the Tetrahedral Au-20 and Au19Pt Clusters: Implications in Adsorption and Sensing, J. Phys. Chem. A, 2015, 119(26), 6909–6918 CrossRef CAS PubMed.
- L. J. Bu, T. A. Gu, Y. X. Ma, C. Chen, Y. M. Tan, Q. J. Xie and S. Z. Yao, Enhanced Cathodic Preconcentration of As(0) at Au and Pt Electrodes for Anodic Stripping Voltammetry Analysis of As(III) and As(V), J. Phys. Chem. C, 2015, 119(21), 11400–11409 CAS.
- C.-Y. Chiu, P.-J. Chung, K.-U. Lao, C.-W. Liao and M. H. Huang, Facet-Dependent Catalytic Activity of Gold Nanocubes, Octahedra, and Rhombic Dodecahedra toward 4-Nitroaniline Reduction, J. Phys. Chem. C, 2012, 116(44), 23757–23763 CAS.
Footnote |
† Electronic supplementary information (ESI) available. See DOI: 10.1039/c5ra27778g |
|
This journal is © The Royal Society of Chemistry 2016 |