DOI:
10.1039/C5RA27514H
(Paper)
RSC Adv., 2016,
6, 21156-21164
Improved charge separation and transport efficiency in panchromatic-sensitized solar cells with co-sensitization of PbS/CdS/ZnS quantum dots and dye molecules†
Received
23rd December 2015
, Accepted 16th February 2016
First published on 16th February 2016
Abstract
A panchromatic hybrid photoelectrode featuring co-sensitization of PbS quantum dots (QDs) and dye N719 with high charge separation efficiency was designed. In this photoelectrode, PbS QDs and N719 dye molecules exhibit a type-II energy level alignment, enabling efficient charge transfer between the two sensitizers and enhanced charge injection efficiency from sensitizers into TiO2, as confirmed by the significant PL quenching and time-resolved photoluminescence. Furthermore, we show the utility of a cobalt(II/III)-based redox electrolyte in solar cells based on PbS–N719 co-sensitized photoelectrodes, achieving a photovoltaic efficiency of over 2%. This result is comparable to the highest efficiencies obtained in cells of this type, and further verifies the important role of N719 as an intermediary agent in hole extraction from the PbS QDs. Compared to QD-only sensitized cells, co-sensitization significantly enhances the cell performance: the overall energy conversion efficiency by about 40% (from 1.55% to 2.12%) and the fill factor by about 20% (from 0.50 to 0.59). However, this system is still far from being optimal, and pathways towards its improvement are discussed.
1. Introduction
Quantum dot sensitized solar cells (QDSSCs), employing inorganic semiconductor nanocrystals as light harvesters, have undergone tremendous improvement in the last several years, and have emerged as competitive candidates to dye sensitized solar cell (DSSCs).1–5 Compared to dye molecules, QDs possess significant advantages, such as high molar extinction coefficients as large as 105 cm−1 M−1, which is of the same order of that of organometal halide perovskite materials,6,7 easily tunable energy levels via controlling and varying the sizes and shapes, large dipole moments for enhanced charge separation, multiple exciton generation (MEG),8–10 and potentially higher stability and resistivity against oxygen and water. Various semiconductor candidates have been used, including both single component QDs such as CdS,11–13 CdSe,14,15 PbS,16,17 CuInS2,18 SnS,19 Ag2S,20 Cu2ZnSnS4
21 and more complex QDs featuring core–shell structures including CdS/CdSe,22 CdTe/CdSe,23 and PbS/CdS.24 Among these, PbS is one of the most promising material due to its spectrally broad absorption, extending far into the infrared region and its size-tunable band gap.25 In particular, it is a promising candidate for the near-infrared component in a tandem photovoltaic cell structure.
Two designs have been successfully pursued for PbS QD-based photovoltaic cells. The first is based on a QD-solid heterojunction, and has achieved efficiencies approaching 9%.26 The second, using QDs as sensitizers in conjunction with a liquid electrolyte has achieved lower efficiencies of a few percent.16,27,28 The performance of PbS-based QDSSCs has been hampered by several factors: the small band offset between the conduction band of the PbS and that of the TiO2 electrode along with the presence of surface states of PbS promotes recombination at this interface. Moreover, a relatively low photovoltage is obtained upon the use of a sulfide-based electrolyte. A steady improvement in the performance of PbS based QDSSCs has, nevertheless, been observed in recent years. This is in part due to the use of better passivation layers for chemical bath deposited PbS,16 as discussed below or improved colloidal synthesis methods.29 One pathway to increase the driving force for electron injection has been the use of Hg doping, which led to the highest reported efficiency of cells of this type, 5.6%.16 Another pathway for improving performance has been the use an iodide-based electrolyte, increasing photovoltage from the characteristic 400–450 mV to up to 600 mV.30 Yet, it is known that iodide-based electrolytes are eventually corrosive towards the QD sensitizers and are thus not a viable option for long-term operation.31
The most efficient PbS QDSSCs have relied on chemical bath deposition (CBD) growth. In these, PbS QDs are grown by sequential dipping of the electrode in precursor solutions, typically resulting in a high density of surface states in the QD itself and a relatively low coverage of TiO2 surface enabling electron leakage with the redox in the electrolyte.32 To inhibit these recombination processes, surface decoration of both the QDs and the exposed TiO2 is a promising approach. Either inorganic layers or small organic molecules have both proven to be effective for this end.33 Perhaps the most common passivation method is via further CBD growth of CdS and ZnS layers.
Co-sensitization by organic dyes is another potential option for passivation,34 with a handful of studies reporting the application of QD-dye co-sensitization. Zaban et al. constructed a CdSe-SQ02 QD-dye co-sensitized structure to obtain enhanced energy power conversion efficiency (PCE) and stability in I−/I3− electrolyte.35 Kamat et al. prepared the TiO2/CdS/Al2O3/JK-216 dye QD-dye co-sensitized photoelectrode and achieved a PCE of 3.14% in cobalt based electrolyte.36 Our group designed the CdS/ZnS/N719 QD-dye co-sensitized photoelectrode and obtained an extremely high PCE of 6.44% in I−/I3− electrolyte.37,38 To fabricate more stable QD-dye co-sensitized system, we constructed CdS/ZnS/N3 dye based solar cell in a cobalt based electrolyte and obtained a PCE of 1.48%. Arof et al. applied LiBOB-based liquid electrolyte in CdS/PbS QD–N3 dye co-sensitized solar cell and gained a PCE of 1.66%.39 More recently, Thampi et al. applied thiolate–disulfide redox electrolyte in CdS QD–N719 dye and PbS QD–N719 dye co-sensitized solar cells, with promising PCEs of 3.93% and 4.18%, respectively.40 Sastre-Santos et al. utilized a phthalocyanine dye ((ZnPcS)2) to passivate CdSe and CdS QDs, increasing the efficiency by 45% for CdSe and 104% for CdS in polysulfide electrolyte.41 Furthermore, the cascade co-sensitization with CdS QDs and zinc phthalocyanines (ZnPcs) dyes was designed and employed in cobalt based electrolyte, achieving significantly improved cell performance.42 Their impressive works proved that QD-dye co-sensitization is a promising strategy to further enhance the overall performance of QDSSCs. Dye molecules can not only improve the light absorption and mitigate recombination on the TiO2–electrolyte interface, but also serve as hole scavengers for the QD sensitizers upon proper band alignment. This can potentially reduce recombination in the QDs as well as provide protection from corrosion by the electrolyte, enabling a more flexible choice of the electrolyte.37,43,44 Additionally, it should be noted that, among all the published QD-dye co-sensitization works, there are scarcely any systems exhibiting panchromatic absorption extending to infrared region.
Here we present a panchromatic co-sensitized QD-dye PbS–N719 system combines the standard CdS and ZnS passivation layers, with a dye sensitizer which is capable of regenerating holes in the QD. Furthermore, this combination allows us to explore an alternative cobalt-based electrolyte redox couple which can potentially increase the open circuit voltage of the cell. We show that the co-sensitized system indeed exhibited significantly reduced recombination, as evidenced by photoluminescence from the PbS QDs, and that the cell can be stably operated with a cobalt(II/III) electrolyte, achieving over 2% efficiency of the cell. Yet, this system is still far from being optimal, and pathways towards its improvement are discussed.
2. Experimental section
2.1 Hybrid film preparation
Mesoscopic TiO2 films were screen-printed on fluorine-doped SnO2 (FTO) conducting glass substrates in a two-step process. First, a ∼7 μm layer of 20 nm colloidal titania (PST-18NR, JGC Catalyst and Chemicals Ltd., Japan) was deposited, followed by a ∼5 μm layer of 400 nm titania particles (PST-400C, JGC Catalyst and Chemicals Ltd., Japan) acting as a scattering layer. Quantum dot sensitized TiO2 films were prepared by the successive ionic layer adsorption and reaction (SILAR) method.15,32 In detail, PbS QDs were grown by immersing TiO2 films in a 0.02 M Pb(CH3COO)2 methanol solution for 1 min and rinsing with pure methanol, followed by immersing in a 0.02 M Na2S methanol solution for 1.5 min and rinsing with pure methanol. This cycle was repeated twice, leading to the formation of ∼2.5 nm PbS nanoparticles. For preparing PbS/CdS QDs, the above as-prepared films were immersed in 0.05 M Cd(CH3COO)2 and 0.05 M Na2S methanol solutions for 1 min and 1.5 min respectively, rinsing with pure methanol between the two immersion steps. The above cycle was repeated 5 times. A similar procedure was used for ZnS coating. The PbS/CdS sensitized films were dipped in 0.1 M Zn(CH3COO)2 and 0.1 M Na2S methanol solutions for 1 min and 1.5 min, respectively. Two deposition cycles were carried out for ZnS. For dye co-sensitization, the PbS/CdS/ZnS sensitized film was immersed in a N719 (5 × 10−4 M) ethanol solution for 20 h.
2.2 Cell fabrication
The counter electrode was prepared by sputtering platinum onto FTO glass for 20 seconds. Cells were prepared by assembling the counter electrodes and respective hybrid photoanodes using a 20 μm thick Surlyn hot melt ring (DuPont, USA). The injected electrolyte solution consisted of 0.22 M Co(bpy)3(PF6)2, 0.05 M Co(bpy)3(PF6)3, 0.1 M LiClO4, and 0.2 M 4-tert-butylpyridine in acetonitrile.
2.3 Characterization
High resolution transmission electron microscopy (HRTEM) measurements were performed using a JEM-2100 electron microscope (JEOL, Japan). To investigate the optical properties of various light harvesting absorbers, measurements of reflectance and transmittance spectra were carried out using a UV/Vis/NIR Spectrophotometer (Lambda 950, Perkin Elmer, USA). The absorbance spectrum was calculated from the reflectance and transmittance data using bare TiO2 electrodes as reference: %absfilm = (%T + %R)TiO2 − (%T + %R)film.37 The vibrational properties of the sensitized TiO2 films were investigated using a Raman spectrometer (HORIBA JOBIN YVON HR800, France), exciting at 488 nm at room temperature. Steady state and time-resolved photoluminescence spectra were obtained using a fluorescence spectrometer (FLS920, Edinburgh Instruments, UK). This system combines time-correlated single photon counting with spectral scanning and is thus very sensitive, featuring a water Raman Signal to Noise Ratio (SNR) of 25
000
:
1. This makes it possible to measure samples with low concentrations and small volumes. Photovoltaic performance of the devices was measured under illumination of AM 1.5 (100 mW cm−2) in a solar simulator (91192, Oriel, USA) equipped with a 450 W xenon lamp (Newport 69920) and a Keithley 2400 source meter. Incident monochromatic photon-to-current conversion efficiency (IPCE) spectra were measured relative to a standard silicon solar cell (QEX10 PV, PV Measurements Inc., USA).
3. Results and disscussion
3.1 HR-TEM measurement
To characterize the size of deposited PbS QDs, HR-TEM was performed on fragments of the sensitized electrode. Fig. 1 compares TEM images of bare TiO2 with PbS QD-sensitized TiO2 films. The presence of small PbS QDs, having a diameter much smaller than that of the TiO2 nanoparticles, is evident upon comparison of the two images. A histogram of the size of 100 PbS particles, as extracted from the high resolution TEM images is presented in Fig. 1(c). As can be seen, the diameter of deposited PbS QDs is quite homogenous with an average value of 2.5 nm. Notably, though the QDs are uniformly distributed on the surface of TiO2, a significant portion of the TiO2 surface remained uncovered. The low coverage of the TiO2 surface was shown to be one of the main reasons leading to increased recombination between the electrons in the conduction band of TiO2 and the oxidized species in the electrolyte, resulting in poor photovoltaic performance of QDSSCs compared to DSSCs.32 As discussed above, a more complete coverage of the TiO2 surface can be reached upon further coating of the photoanode. Here, this was achieved both by further deposition of CdS, ZnS and by co-sensitization with small organic molecules.34
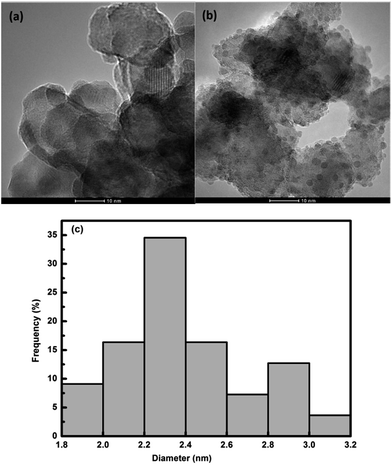 |
| Fig. 1 TEM images for different films: (a) pure TiO2, (b) PbS QD-sensitized TiO2 film (the larger nanoparticles correspond to TiO2, while the smaller ones are PbS QDs), (c) size distribution of PbS QDs. | |
3.2 Absorption properties
To figure out how the QD and co-sensitized structures affect the light harvesting efficiency, the absorption spectra of various light harvesting absorbers were measured as illustrated in Fig. 2. PbS QD-sensitized TiO2 films exhibit an absorption onsets at about 1150 nm (∼1 eV), which corresponds reasonably with the expected band gap of quantum confined PbS at the larger end of the distribution presented in Fig. 1(c). Further deposition of CdS blueshifted the absorption edge but redshifted the onset of significant absorption in the spectral range of 700–1000 nm.28 The former is likely attributed to cation exchange of the outer layer of PbS to CdS along with CdS growth due to the fact that the solubility product constant, Ksp, of CdS (8 × 10−27) is close to that of PbS (1 × 10−28).38 The latter is attributed to a reduction of the quantum confinement in PbS due to significant delocalization of the electron into the CdS conduction band. ZnS deposition resulted only in redshift of the absorption curve, indicating that cation exchange didn't occur, due to the much larger Ksp of ZnS (2.5 × 10−22). The enhanced absorption reveals the presence of electronic coupling between ZnS and the PbS QDs similar to that in the PbS/ZnS nanocrystals,46 where tunneling of the QD electron wave function into the surrounding ZnS shell reduces the confinement energy. As can be seen from Fig. 2, deposition of ZnS on the PbS/CdS (PbS/CdS/ZnS) sensitized films caused even more obvious enhancement of the absorption than the PbS/CdS and PbS/ZnS sensitized film, which was attributed to a further reduction in the quantum confinement of PbS QDs. Further deposition of N719 on TiO2/PbS/CdS/ZnS film led to a slight increase of the absorption in the range 800–1100 nm. Overall, all samples exhibited panchromatic absorption, making them promising candidates to serve as efficient light harvesting absorbers.
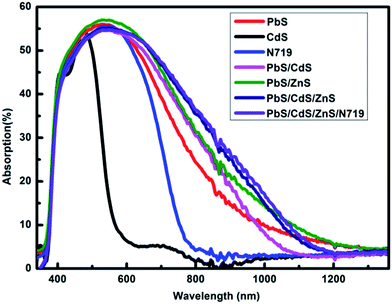 |
| Fig. 2 Absorption spectra of TiO2 films with different sensitizers including PbS, CdS, N719, PbS/CdS, PbS/ZnS, PbS/CdS/ZnS and PbS/CdS/ZnS/N719. | |
Based on the analysis from HRTEM measurement and absorption properties, we derive an approximate energy level alignment of the sensitized films, as shown in Fig. 3. Hyun et al. has investigated the relationship between the energy levels for PbS QDs and its sizes, from which the valence band and conduction band edges of PbS QDs would vary along a continuous hyperbola. Therefore, the valence band and conduction band edges of PbS QDs we used in our research were estimated to be ∼−5.4 eV and ∼−3.9 eV respectively, according to its size of 2.5 nm displayed in the TEM images.27 This is also in consistent with the absorption onsets of pure PbS QD-sensitized TiO2 films. Meanwhile, the bandgap and band alignment of CdS in this work is in accordance with those of the bulk material.47 As we show below, the slightly higher conduction band of PbS than that of TiO2 enabled the efficient electron injection from the former to the latter. Meanwhile the even higher conduction band of CdS also led to efficient electron injection from CdS to the TiO2 photoanode, which enables it to not only serve as passivating layer but also to contribute to light absorption. Importantly, the PbS/CdS interface formed here exhibited a type-I energy level alignment. Similar results were also obtained for ZnS deposition, where the conduction band position of ZnS is even higher. Finally, the HOMO of N719 was found to be higher than the valence band of both PbS and CdS, which demonstrates that N719 could serve as hole scavenger of the QDs. The TiO2/PbS/CdS/ZnS/N719 structure is thus expected to improve the QD regeneration efficiency by rapidly scavenging the hole from the QD to the N719.48 This schematic energy level diagram plotted here is also supported by findings of Raman spectroscopy, PL and time-resolved PL spectra as shown below.
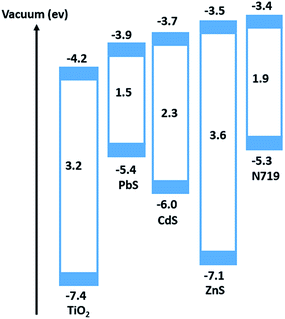 |
| Fig. 3 Obtained energy level of PbS QDs and the energy diagram for the hybrid TiO2/PbS/CdS/ZnS/N719 photoanode. | |
3.3 Raman spectroscopy
Raman spectra of TiO2 films with different sensitizers are shown in Fig. 4. Typically, all the photoanodes exhibit distinguishable Raman intensity at ∼149 cm−1, ∼238 cm−1, ∼448 cm−1, ∼611 cm−1, which can be attributed to the Eg Raman mode of anatase TiO2, a Raman mode of rutile TiO2, the B1g mode of rutile TiO2, and the Eg Raman mode of rutile TiO2, respectively.49 The resonantly excited longitudinal optical (LO) phonon of CdS QDs located at ∼300 cm−1 is also evident in the observed Raman spectrum.50 The Raman modes of PbS QDs were not observed in our work, indicating the as-prepared PbS QDs were wholly Raman silent, which is consistent with previous reports.51,52 As for the QDs only sensitized films, further deposition of passivating layers (including TiO2/PbS/CdS, TiO2/PbS/ZnS, TiO2/PbS/CdS/ZnS) associated with increased thickness of the sensitizers (Fig. 4(a)), obviously lowered the relative intensity of the Raman peaks originating from the TiO2 and CdS QDs. N719 co-sensitization introduced additional Raman peaks between 1200 cm−1 and 2000 cm−1 associated with molecular vibrations of the dye co-sensitizer (Fig. 4(b)). Notably, the Raman spectrum of N719 bound to the TiO2/PbS/CdS/ZnS film differs from that of N719 directly bound to TiO2 (Fig. 4(b)). Clear frequency shifts, intensity variations, and broadening of the bipyridine ring stretching modes (1480–1615 cm−1) as well as the C–C inter-ring and C–O stretching modes (1270–1320 cm−1) of the N719 dye for the film with co-sensitization could be observed. This behavior, especially the increased damping of the dye vibrations inferred from the Raman peak broadening, is a characteristic feature of the efficient grafting of the dye molecules on the TiO2/QD surface.53,54 Moreover, at ∼1370 cm−1 (identified with the symmetric COO− stretching mode) a broad Raman band appeared for the TiO2/PbS/CdS/ZnS/N719 film while a narrow band appeared on TiO2/N719 light harvesting absorbers.55 All these changes are a strong indication that the dye molecules were bound to the QDs through its carboxylate group.45,56 Overall, the Raman spectrum indicates that dye molecules adsorbed both directly on TiO2 and on top of the QD sensitizers.
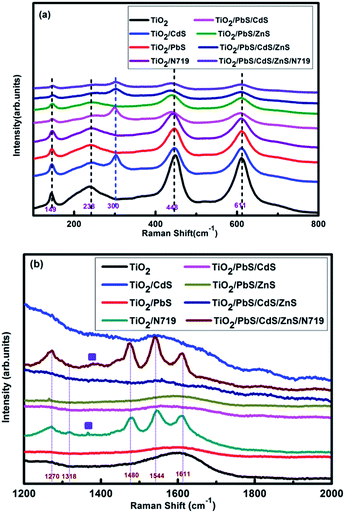 |
| Fig. 4 Raman spectra of all the different light harvest absorbers at (a) 100–800 cm−1, (b) 1200–2000 cm−1 of TiO2 films sensitized by pure N719 and hybrid structure of PbS/CdS/ZnS/N719. | |
3.4 Charge transfer process and energy level alignment
To understand the charge transfer processes among the sensitizers, PL spectra of different light harvesting absorbers were achieved and shown in Fig. 5. The PbS QDs sensitized TiO2 samples exhibit a near-infrared PL peak at about 1200 nm stemming from the band-edge emission of the PbS QDs. Upon further coating the PbS surface with either CdS or ZnS, significant red shifts of the band-edge PL were observed: ∼93 nm for TiO2/PbS/CdS and ∼212 nm for TiO2/PbS/ZnS, respectively. This is in good agreement with the absorption measurements shown above.28 In addition, the fact that the red shift of the PL band for the TiO2/PbS/CdS is smaller than that of TiO2/PbS/ZnS, serving as another indication of partial cation exchange of the Pb2+ in the PbS QDs with Cd2+, as supported also by the absorption spectrum. Interestingly, further deposition of ZnS on TiO2/PbS/CdS led to a small blue shift of the PL peak. This is probably a result of the increased thicknesses of TiO2/PbS/CdS/ZnS which enlarged the interphase strain between PbS and CdS layers, leading to an enhancement of quantum confinement.57 Notably, the PL peak intensity was remarkably increased upon over-coating with CdS or ZnS. We attribute this to enhanced luminescent quantum yields compared to bare TiO2/PbS, which reflects the fact that ZnS or CdS act as effective passivation layers for surface states in PbS QDs. Additionally, the CdS or ZnS shell physically protect the active PbS core from the surrounding medium, therefore the sensitivity of the emission properties for PbS QDs towards oxygen or water molecules may be reduced. Therefore, the TiO2/PbS/ZnS and TiO2/PbS/CdS core/shell systems will likely exhibit enhanced stability against photodegradation. At the same time, both CdS and ZnS shells could reduce the number of surface dangling bonds acting as trap states for charge carriers.58
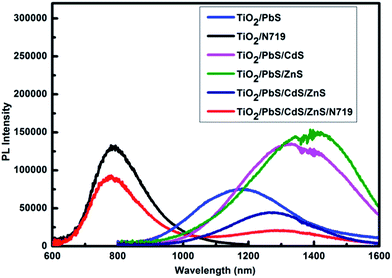 |
| Fig. 5 PL emission spectra for different light harvesting absorbers. | |
Surprisingly, the PL of the TiO2/PbS/CdS/ZnS film was lower than that of the TiO2/PbS, TiO2/PbS/CdS and TiO2/PbS/ZnS films. The likely origin of this effect is associated with a strain-induced change of the band alignment upon ZnS growth on top of the CdS layer. The effects of deposition of the CdS and ZnS passivation layers on the band alignment are schematically described in Fig. 6. Upon deposition of CdS on PbS the conduction band edge of PbS QD is shifted downward because of Fermi-level alignment between CdS and PbS.59 This results in a smaller electron injection driving force between PbS and TiO2 and a concomitant increase in the PL. Further deposition of ZnS onto the PbS/CdS QDs is ascribed to the upward shifted conduction band of PbS (as shown in Fig. 6) due to the interphase strain between the PbS and the CdS layers.57 Since this increases the driving force for electron injection into the TiO2, PL is quenched. Further confirmation of this effect is presented in the time-resolved PL measurements below.
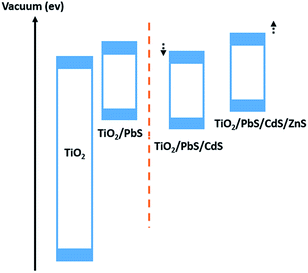 |
| Fig. 6 The schematic variation of energy level alignment of PbS relative to bare TiO2 in films sensitized by pure PbS QDs, PbS/CdS QDs and ZnS coated PbS/CdS QDs, respectively. | |
Notably, as for TiO2/PbS/CdS/ZnS/N719 film, a significant reduction of the PL is observed both for the QD band edge emission and for the N719 emission (relative to the TiO2/N719 sample). This remarkable quenching of both the QD emission and the N719 emission indicates a fast photoinduced charge transfer between the PbS QDs and the N719 dye due to the type-II band alignment between the two moieties. Furthermore, it should be pointed out that even in the presence of ZnS coating as an intervening dielectric layer between the two sensitizers, strong photoinduced charge transfer was operative. This reveals that at least for 1–2 deposition steps, ZnS deposition is a good compromise which introduces electronic passivation without suffering a significant penalty to the photoinduced charge transfer probability.
To further investigate the charge separation and charge transfer processes, time-resolved PL was performed as shown in Fig. 7. Transient curves were fitted using the two exponential lifetime model as follows:35
R(t) = α1e−t/τ1 + α2e−t/τ2 + α0. |
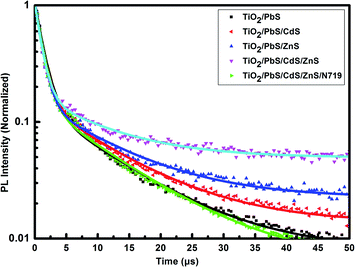 |
| Fig. 7 Time-resolved PL spectra in the time range of 50 μs measured for light harvesting absorbers of TiO2/PbS, TiO2/PbS/CdS, TiO2/PbS/ZnS, TiO2/PbS/CdS/ZnS and TiO2/PbS/CdS/ZnS/N719. The spectra in the time range of 2 μs measured for all the samples were shown in the inset. | |
As can be seen, all samples show a more or less similar fast component, on the order of several microseconds, accounting for about 90% of the emission decay. The longer decay transient is composed of two components: an intermediate decay term of about 11 microseconds and one which is longer than the instrument response and appears as a constant. The most dramatic effect, representing improved passivation going from bare PbS through CdS and ZnS coatings to the dual CdS/ZnS coating is the increase of the long component at the expense of the intermediate one. Upon binding of N719 the long component decreases even below its amplitude for bare PbS, indicating emission quenching due to charge separation, where dye molecules extract holes in the QDs after excitation due to the type-II energy level alignment.
Based on the investigations of Raman spectroscopy, steady state and time-resolved PL spectra, the main processes of charge transfer in TiO2/PbS/CdS/ZnS and TiO2/PbS/CdS/ZnS/N719 films are demonstrated in Fig. 8. Photoinduced free electron–hole pairs are produced (process 1), followed by the electron injection from the conduction band of QDs into TiO2 (process 3). This is, however, hampered by various recombination channels. These include recombination between electrons in the conduction band and holes in the valence band in the PbS QDs (process 2). This internal recombination can be either non-radiative or radiative, of which the portion of the radiative recombination can be detected by PL measurements. Another recombination channel involves electrons in the conduction band of TiO2 and holes left in the QD (process 4). When CdS and ZnS were deposited on PbS surface, process 2 is slowed down and meanwhile the non-radiative recombination in PbS is also decreased. When N719 was further adsorbed to the TiO2/PbS/CdS/ZnS film, additional charge transfer processes occur. Under illumination, photoinduced free electron–hole pairs are produced in both QDs and N719, therefore the electrons from the excited state of N719 would be transferred to TiO2 and possibly to QDs (noted as process 5 and 6, respectively). At the same time, fast scavenging of holes from the QDs by N719 can occur, due to the type-II band alignment (process 7), thus regenerating the QDs. Consequently, both the recombination channels of process 2 and process 4 are suppressed. Reduction of radiative recombination is directly evidenced by the PL quenching after N719 deposition. On the other hand, PbS/CdS/ZnS QD also physically separated the photo-injected electrons in TiO2 and holes in the dyes, so the charge recombination process between TiO2 and dyes (process 8) is likely inhibited.
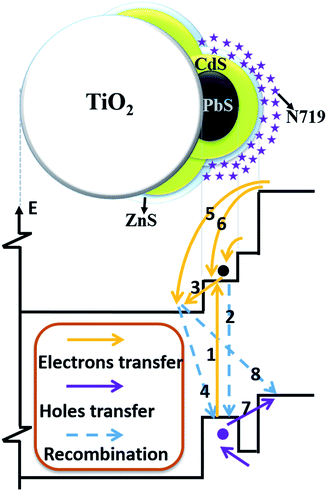 |
| Fig. 8 Schematic energy diagram of carrier generation, transfer, and recombination in the TiO2/PbS/CdS/ZnS/N719 film. The arrows indicate the main processes occurring in the sensitized films under light illumination. The solid lines show the main processes of carriers (electrons and holes) generation and transfer, while the dash lines indicate the recombination between electrons and holes in the TiO2/PbS/CdS/ZnS/N719 film. | |
3.5 Photoelectrochemical solar cells
The above analysis is further verified by the J–V curves and IPCE curves of the various fabricated cells, as shown in Fig. 9. The photovoltaic parameters including short-circuit photocurrent density (Jsc), open-circuit voltage (Voc), fill factor (FF), overall energy conversion efficiency (η) and Jsc calculated from IPCE spectra are listed in Table 1, which clearly shows that both the inorganic layer passivation and the dye co-sensitization dramatically improved the performance of the PbS sensitized solar cells. In detail, the PbS-sensitized solar cell without any passivation exhibits low overall efficiency of only 0.31%. This is mainly due to the extremely poor Jsc of 1.46 mA cm−2, which is induced by various recombination channels, as shown in Fig. 8. The direct recombination from the PbS QDs to the electrolyte redox species probably constituted a significant electron loss pathway, ascribed to the presence of surface states in the QDs acting as recombination centers.60 Additional recombination channels include recombination between electrons in the conduction band and holes in the valence band in PbS itself occurred (Fig. 8 process 2) and direct electron recombination from TiO2 to electrolyte redox species due to the low surface coverage by PbS QDs.32 By coating the TiO2/PbS surface with either CdS or ZnS blocking layer, a considerable enhancement in both Jsc and Voc was achieved, demonstrating the suppression of charge recombination in the PbS QDs as well as the improved passivation of the interface with the electrolyte redox species. This resulted in elevation of the power conversion efficiency to 1.10% (TiO2/PbS/CdS) and 0.45% (TiO2/PbS/ZnS). It is also noted that the Jsc of TiO2/PbS/CdS solar cell is higher than that of TiO2/PbS/ZnS solar cell, which is due to the additional light absorption by CdS QDs, while the FF of later cell is higher than that of the previous one, demonstrating better passivation effect by ZnS layer. Further deposition of a ZnS layer on top of the TiO2/PbS/CdS photoanode improved the cell performance, especially in Jsc, which is almost doubled from 4.54 to 6.51 mA cm−2. This is because ZnS layer not only serves as an effective blocker of charge recombination from QDs to the electrolytes (due to its wide band gap), but also leads to larger electron injection driving force for the TiO2/PbS/CdS/ZnS electrode than that of the TiO2/PbS/CdS and TiO2/PbS ones as discussed in Fig. 6.
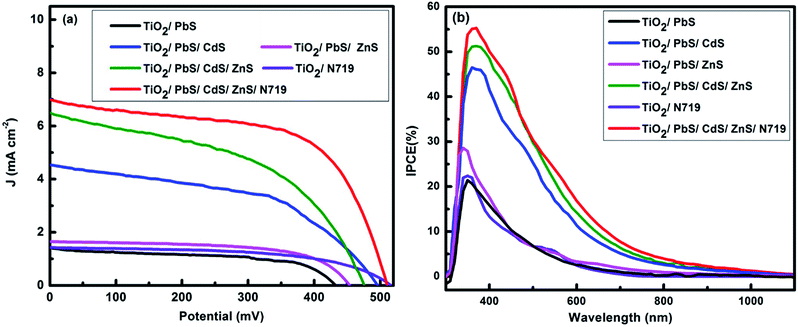 |
| Fig. 9 (a) Photocurrent density–voltage (J–V) characteristics under AM 1.5 illumination (100 mW cm−2) and (b) incident photon-to-current conversion efficiency (IPCE) action spectra of solar cells fabricated with TiO2 photoelectrodes with different sensitizers. | |
Table 1 Photovoltaic characteristics of cells fabricated with TiO2 photoelectrodes with different sensitizers under AM 1.5 illumination (100 mW cm−2)
Photoanodes |
η (%) |
Voc (mV) |
FF |
Jsc (mA cm−2) |
Jsc from IPCE (mA cm−2) |
TiO2/PbS |
0.31 |
433 |
0.49 |
1.46 |
1.29 |
TiO2/PbS/CdS |
1.10 |
497 |
0.49 |
4.54 |
4.22 |
TiO2/PbS/ZnS |
0.45 |
455 |
0.60 |
1.65 |
1.52 |
TiO2/PbS/CdS/ZnS |
1.55 |
479 |
0.50 |
6.51 |
5.48 |
TiO2/N719 |
0.41 |
517 |
0.55 |
1.43 |
1.21 |
TiO2/PbS/CdS/ZnS/N719 |
2.12 |
513 |
0.59 |
7.00 |
6.22 |
Most importantly, a prominent improvement of all photovoltaic parameters was achieved for the solar cell based on TiO2/PbS/CdS/ZnS/N719 electrode compared with all of those based on only one sensitizer, with efficiency even larger than the summed efficiency of the separate cells. To analyze the N719 sensitizer's contribution to the photovoltaic performance, a TiO2 photoanode stained by N719 alone for 20 h was incorporated into a solar cell, which showed a Jsc of 1.43 mA cm−2, Voc of 517 mV, FF of 0.55, and an efficiency of 0.41% due to the formation of a complex between the cobalt electrolyte and the surface adsorbed dye which brings the [Co(bpy)3]3+ species into contact with the TiO2 surface.61 While the PbS/CdS/ZnS/N719 sensitized solar cell exhibited a Jsc of 7.00 mA cm−2, Voc of 513 mV, FF of 0.59, and an overall energy conversion efficiency of 2.12% (which is comparable to the highest efficiencies obtained in cells of this type). As was discussed above, this significant photovoltaic performance improvement is attributed to the type-II energy level alignment between QDs and N719 dye, enabling efficient charge transfer between the two sensitizers as confirmed by the significant PL quenching. To check the recombination inhibition by N719, we also used the EIS, which was shown and explained in Fig. S1 (in the ESI†). In addition, ZnS layer serves here as an effective blocker of charge recombination from QDs to the electrolytes rather than an undesired barrier of electron injection from N719 to TiO2 when it is thin enough, as has also been reported previously.37
The IPCE is given by eqn (1):
|
IPCE = LHE × ηinj × ηc
| (1) |
where LHE is the light harvesting efficiency at a given wavelength,
ηinj is the electron injection efficiency, and
ηc is the charge collection efficiency. As seen in
Fig. 9(b), the IPCE values were in good agreement with the trend in
Jsc values. As noted in the above discussion of
Fig. 2, the absorption of the various photoelectrodes tested here does not dramatically differ from one to another. Yet, there are very significant differences in the IPCE spectra, which therefore reflect the efficiency of passivation and charge separation processes, thus leading to significantly different
ηinj and
ηc. Notably, however, the IPCE curves do not fully reflect the absorption spectra. While the absorption peaks at about 600 nm, the IPCE curve peaks at a shorter wavelength. One possible explanation for this is the dependence of the carrier extraction efficiency on the size (and thus the band gap) of the PbS sensitizers.
4. Conclusion
In summary, we have constructed PbS-based QDSSCs which take advantage of both co-sensitization with an organic dye and a cobalt-based electrolyte. We have performed a systematic investigation of the electronic coupling between QDs and N719 dye, from which N719 molecules were known to serve as regenerating agents of the PbS QDs due to the type-II band alignment of the two. Then we have shown that a cobalt-based electrolyte system can be utilized in PbS–N719 co-sensitized solar cells, achieving photovoltaic efficiency of over 2%. This result is comparable to the highest efficiencies obtained in cells of this type, also demonstrating efficient charge transfer between the two sensitizers and thus inhibits the recombination in the photovoltaic solar cells. Yet, it appears that the photovoltaic performance could be further improved via a small increase in the band gap of the PbS sensitizers (perhaps via further cation exchange with Cd2+) so as to shift more of the PbS ensemble into the spectral region where carrier extraction in the full cell was efficient. This may contribute to the fabrication of high-voltage QDSSCs which are presently sparse.
Acknowledgements
The authors express their gratitude for the support provided by the Ministry of Science & Technology, Israel and the Ministry of Science & Technology, P.R. China: the China-Israel Cooperative Scientific Research Fund (2015DFG52690), and the National Natural Science Foundation of China (NSFC, 51272126).
References
- H. McDaniel, N. Fuke, N. S. Makarov, J. M. Pietryga and V. I. Klimov, Nat. Commun., 2013, 4, 1–10 CrossRef PubMed.
- J. H. Rhee, C. Chung and E. W. Diau, NPG Asia Mater., 2013, 5, 1–17 CrossRef.
- P. V. Kamat and J. Phys, Chem. Lett., 2013, 4, 908–918 CAS.
- I. Hod and A. Zaban, Langmuir, 2013, 30, 7264–7273 CrossRef PubMed.
- Z. Du, H. Zhang, H. Bao and X. Zhong, J. Mater. Chem. A, 2014, 2, 13033–13040 CAS.
- W. W. Yu, L. Qu, W. Guo and X. Peng, Chem. Mater., 2003, 15, 2854–2860 CrossRef CAS.
- J. Burschka, N. Pellet, S. Moon, R. Humphry-Baker, P. Gao, M. K. Nazeeruddin and M. Gratzel, Nature, 2013, 499, 316–319 CrossRef CAS PubMed.
- O. E. Semonin, J. M. Luther, S. Choi, H. Chen, J. Gao, A. J. Nozik and M. C. Beard, Science, 2011, 334, 1530–1533 CrossRef CAS PubMed.
- C. M. Cirloganu, L. A. Padilha, Q. Lin, N. S. Makarov, K. A. Velizhanin, H. Luo, I. Robel, J. M. Pietryga and V. I. Klimov, Nat. Commun., 2014, 5, 1–8 Search PubMed.
- A. J. Nozik, Chem. Phys. Lett., 2008, 457, 3–11 CrossRef CAS.
- Z. Zheng, W. Xie, Z. S. Lim, L. You and J. Wang, Sci. Rep., 2014, 4, 1–6 Search PubMed.
- K. Meng, P. K. Surolia, O. Byrne and K. R. Thampi, J. Power Sources, 2014, 248, 218–223 CrossRef CAS.
- H. Shen, H. Shen, H. Lin, H. Lin, L. Zhao, L. Zhao, Y. Liu, Y. Liu, D. Oron and D. Oron, J. Nanosci. Nanotechnol., 2013, 13, 1095–1100 CrossRef CAS PubMed.
- I. Robel, V. Subramanian, M. Kuno and P. V. Kamat, J. Am. Chem. Soc., 2006, 128, 2385–2393 CrossRef CAS PubMed.
- H. Lee, M. Wang, P. Chen, D. R. Gamelin, S. M. Zakeeruddin, M. Grätzel and M. K. Nazeeruddin, Nano Lett., 2009, 9, 4221–4227 CrossRef CAS PubMed.
- J. Lee, D. Son, T. K. Ahn, H. Shin, I. Y. Kim, S. Hwang, M. J. Ko, S. Sul, H. Han and N. Park, Sci. Rep., 2013, 3, 1–8 Search PubMed.
- S. D. Sung, I. Lim, P. Kang, C. Lee and W. I. Lee, Chem. Commun., 2013, 49, 6054–6056 RSC.
- J. Luo, H. Wei, Q. Huang, X. Hu, H. Zhao, R. Yu, D. Li, Y. Luo and Q. Meng, Chem. Commun., 2013, 49, 3881–3883 RSC.
- Y. Oda, H. Shen, L. Zhao, J. Li, M. Iwamoto and H. Lin, Sci. Technol. Adv. Mater., 2014, 15, 35006 CrossRef.
- H. Shen, X. Jiao, D. Oron, J. Li and H. Lin, J. Power
Sources, 2013, 240, 8–13 CrossRef CAS.
- K. Abe, H. Shen, X. Li, L. Zhao, X. Zhao, J. Li, M. Iwamoto and H. Lin, Polyhedron, 2014, 82, 148–153 CrossRef CAS.
- Z. Pan, H. Zhang, K. Cheng, Y. Hou, J. Hua and X. Zhong, ACS Nano, 2012, 6, 3982–3991 CrossRef CAS PubMed.
- S. Itzhakov, H. Shen, S. Buhbut, H. Lin and D. Oron, J. Phys. Chem. C, 2012, 117, 22203–22210 Search PubMed.
- V. Gonzalez-Pedro, C. Sima, G. Marzari, P. P. Boix, S. Gimenez, Q. Shen, T. Dittrich and I. Mora-Sero, Phys. Chem. Chem. Phys., 2013, 15, 13835–13843 RSC.
- G. D. Scholes and G. Rumbles, Nat. Mater., 2006, 5, 683–696 CrossRef CAS PubMed.
- C. H. M. Chuang, P. R. Brown, V. Bulovic and M. G. Bawendi, Nat. Mater., 2014, 13, 796–801 CrossRef CAS PubMed.
- B. Hyun, Y. Zhong, A. C. Bartnik, L. Sun, H. D. Abruña, F. W. Wise, J. D. Goodreau, J. R. Matthews, T. M. Leslie and N. F. Borrelli, ACS Nano, 2008, 2, 2206–2212 CrossRef CAS PubMed.
- A. Braga, S. Giménez, I. Concina, A. Vomiero and I. Mora-Seró, J. Phys. Chem. Lett., 2011, 2, 454–460 CrossRef CAS.
- L. Lai, L. Protesescu, M. V. Kovalenko and M. A. Loi, Phys. Chem. Chem. Phys., 2014, 16, 736–742 RSC.
- S. S. Rao, I. K. Durga, C. V. Tulasi-Varma, D. Punnoose, L. J. Cheol and H. Kim, New J. Chem., 2015, 39, 7379–7388 RSC.
- M. Grätzel, Acc. Chem. Res., 2009, 42, 1788–1798 CrossRef PubMed.
- I. Mora-Seró, S. Giménez, F. Fabregat-Santiago, R. Gómez, Q. Shen, T. Toyoda and J. Bisquert, Acc. Chem. Res., 2009, 42, 1848–1857 CrossRef PubMed.
- M. Sykora, M. A. Petruska, J. Alstrum-Acevedo, I. Bezel, T. J. Meyer and V. I. Klimov, J. Am. Chem. Soc., 2006, 128, 9984–9985 CrossRef CAS PubMed.
- I. Mora-Seró and J. Bisquert, J. Phys. Chem. Lett., 2010, 1, 3046–3052 CrossRef.
- S. Buhbut, S. Itzhakov, E. Tauber, M. Shalom, I. Hod, T. Geiger, Y. Garini, D. Oron and A. Zaban, ACS Nano, 2010, 4, 1293–1298 CrossRef CAS PubMed.
- H. Choi, R. Nicolaescu, S. Paek, J. Ko and P. V. Kamat, ACS Nano, 2011, 5, 9238–9245 CrossRef CAS PubMed.
- H. Shen, H. Lin, Y. Liu, J. Li and D. Oron, J. Phys. Chem. C, 2012, 116, 15185–15191 CAS.
- S. Luo, H. Shen, Y. Zhang, J. Li, D. Oron and H. Lin, Electrochim. Acta, 2016, 191, 16–22 CrossRef CAS.
- H. K. Jun, M. H. Buraidah, M. M. Noor, M. Z. Kufian, S. R. Majid, B. Sahraoui and A. K. Arof, Opt. Mater., 2013, 36, 151–158 CrossRef CAS.
- K. Meng, P. K. Surolia, O. Byrne and K. R. Thampi, J. Power Sources, 2015, 275, 681–687 CrossRef CAS.
- V. M. Blas-Ferrando, J. Ortiz, V. Gonzalez-Pedro, R. S. Sanchez, I. Mora-Sero, F. Fernandez-Lazaro and A. Sastre-Santos, Chem. Commun., 2015, 51, 1732–1735 RSC.
- V. M. Blas-Ferrando, J. Ortiz, V. González-Pedro, R. S. Sánchez, I. Mora-Seró, F. Fernández-Lázaro and Á. Sastre-Santos, Adv. Funct. Mater., 2015, 25, 3220–3226 CrossRef CAS.
- H. Lee, H. C. Leventis, S. Moon, P. Chen, S. Ito, S. A. Haque, T. Torres, F. Nüesch, T. Geiger, S. M. Zakeeruddin, M. Grätzel and M. K. Nazeeruddin, Adv. Funct. Mater., 2009, 19, 2735–2742 CrossRef CAS.
- I. Mora-Seró, T. Dittrich, A. S. Susha, A. L. Rogach and J. Bisquert, Thin Solid Films, 2008, 516, 6994–6998 CrossRef.
- Y. Justo, L. K. Sagar, S. Flamee, Q. Zhao, A. Vantomme and Z. Hens, ACS Nano, 2014, 8, 7948–7957 CrossRef CAS PubMed.
- B. O. Dabbousi, J. Rodriguez-Viejo, F. V. Mikulec, J. R. Heine, H. Mattoussi, R. Ober, K. F. Jensen and M. G. Bawendi, J. Phys. Chem. B, 1997, 101, 9463–9475 CrossRef CAS.
- Y. Xu and M. A. A. Schoonen, Am. Mineral., 2000, 85, 543–556 CrossRef CAS.
- H. Shen, J. Li, L. Zhao, S. Zhang, W. Wang, D. Oron and H. Lin, Phys. Chem. Chem. Phys., 2014, 16, 6250–6256 RSC.
- C. A. Melendres, A. Narayanasamy, V. A. Maroni and R. W. Siegel, J. Mater. Res., 1989, 4, 1246–1250 CrossRef CAS.
- O. Madelung, Semiconductors: Data Handbook, Springer, Berlin, Heidelberg, 2004 Search PubMed.
- T. P. Mernagh and A. G. Trudu, Chem. Geol., 1993, 103, 113–127 CrossRef CAS.
- J. G. Shapter, M. H. Brooker and W. M. Skinner, Int. J. Miner. Process., 2000, 60, 199–211 CrossRef CAS.
- V. Likodimos, T. Stergiopoulos, P. Falaras, R. Harikisun, J. Desilvestro and G. Tulloch, J. Phys. Chem. C, 2009, 113, 9412–9422 CAS.
- C. Pérez León, L. Kador, B. Peng and M. Thelakkat, J. Phys. Chem. B, 2006, 110, 8723–8730 CrossRef PubMed.
- C. Pérez León, L. Kador, B. Peng and M. Thelakkat, J. Phys. Chem. B, 2005, 109, 5783–5789 CrossRef PubMed.
- I. Mora-Seró, V. Likodimos, S. Giménez, E. Martínez-Ferrero, J. Albero, E. Palomares, A. G. Kontos, P. Falaras and J. Bisquert, J. Phys. Chem. C, 2010, 114, 6755–6761 Search PubMed.
- J. Kim, H. Choi, C. Nahm, C. Kim, J. Ik Kim, W. Lee, S. Kang, B. Lee, T. Hwang, H. Hejin Park and B. Park, Appl. Phys. Lett., 2013, 102, 183901 CrossRef.
- P. Reiss, M. Protière and L. Li, Small, 2009, 5, 154–168 CrossRef CAS PubMed.
- C. Chi, H. Cho, H. Teng, C. Chuang, Y. Chang, Y. Hsu and Y. Lee, Appl. Phys. Lett., 2011, 98, 012101 CrossRef.
- G. Hodes, J. Phys. Chem. C, 2008, 112, 17778–17787 CAS.
- E. Mosconi, J. Yum, F. Kessler, C. J. Gómez García, C. Zuccaccia, A. Cinti, M. K. Nazeeruddin, M. Grätzel and F. De Angelis, J. Am. Chem. Soc., 2012, 134, 19438–19453 CrossRef CAS PubMed.
Footnotes |
† Electronic supplementary information (ESI) available. See DOI: 10.1039/c5ra27514h |
‡ These authors contributed equally to this work. |
|
This journal is © The Royal Society of Chemistry 2016 |