DOI:
10.1039/C5RA26606H
(Paper)
RSC Adv., 2016,
6, 16500-16506
An electrochemiluminescence lab-on-paper device for sensitive detection of two antigens at the MCF-7 cell surface based on porous bimetallic AuPd nanoparticles†
Received
13th December 2015
, Accepted 1st February 2016
First published on 3rd February 2016
Abstract
In this work, a novel paper-based electrochemiluminescence (ECL) immunosensor for ultrasensitive and selective detection of corresponding antigens on living cells was developed employing AuPd nanoparticles as a signal amplifier and macroporous Au-paper working electrodes (Au-PWE) for efficient cell capture. The porous AuPd nanoparticles are chosen as label nanomaterials due to their controllable three-dimensional porosity and peroxidase like activity, large surface area, superior conductivity, and favorable catalytic activity to H2O2 which could enhance the ECL intensity for signal amplification. CdTe QDs and luminol groups as ECL probes were linked with the antibodies to recognize the corresponding antigens on the cell surface and the wide potential range between them compared to conventional antigens sensors could prevent interfacing with each other. Using this strategy, human carcinoembryonic (CEA) and alphafetoprotein (AFP) antigens on living MCF-7 cancer cells as models were quantified serially through a potential scanning way. Significantly, this strategy may be quite promising in cancer early diagnosis due to the excellent analytical performance.
1. Introduction
In recent years, cancer has greatly threatened people's health1,2 which originates from genetic abnormalities that usually cause the affected cells to behave differently at the molecular level.3,4 Recently, there has been a considerable interest in the design and fabrication of biosensors for cancer cell detection and their activity monitoring.5–7 As known, the key biomolecules on the cell membrane, the surface differentiation antigen clusters and receptors, may vary significantly during cancer occurrence.8–11 Moreover, compared with the multiplexed ECL determination of surface antigens with a series of electrodes, the electrode which could evaluate surface antigens of one cell population could demonstrate the correlation of antigens at the same cell surface.12 Potential-resolved ECL detection of two antigens at the cell surface has been previously reported by F. Han, et al.13 There are still remained research interests in the present work, such as combining the detection method with low-cost microfluidic paper based electrode and signal amplifying strategy by using nanostructures. Moreover, the semiconductor nanoparticles showed cathodic ECL in the presence of coreactant, such as K2S2O8 and H2O2,14,15 and luminol which with anodic ECL16,17 could be integrated into one ECL system for dual-potential ECL with no mutual interference so that the multiplex detection can be achieved.
Whitesides and co-workers18 firstly demonstrated a novel lab-on-a-paper systems using patterned paper as substrate, named microfluidic paper-based analytical devices (μ-PADs).19 μ-PADs represent new and outstanding paradigms has been widely used for fluid manipulation,20 diagnostic testing,21 and environmental monitoring22 applications. Recently, lab-on-paper techniques have been endowed with new applications for cytological or histological studies.23–26 The development of paper-based cyto-device would offer a promising potential to realize easy-to-use, inexpensive, miniaturized, and high-throughput researches about living cells in various areas. Among various analysis methods, such as chemiluminescent,27 fluorescent,28 electrochemical29 on μ-PADs, ECL detection has attracted considerable interest for its distinct advantages.30 In our former reports, potential-resolved detection of multiple cancer markers have been constructed on the paper working electrode (PWE),31,32 while there is still need to improve the sensitivity and simplicity in the detection of multiple antigens on cancer cells. In this manuscript, to improve the ECL platform and label nanomaterials, Au nanoflowers were grown on the surface of paper fiber in PWE and AuPd nanoparticles were introduced. The introduction of Au nanoflowers on the PWE could largely improve the ECL performance due to its large specific surface for immobilizing antibodies and remarkable conductivity for promoting ECL signal.
Bimetallic nanoparticles have received intensive attention due to its novel optical, electronic, magnetic, and catalytic properties, which differ from those of their respective constituent metals because of the synergistic effect and electronic effect.33,34 Due to their distinctive properties and application potentials related to thermal- and electrocatalysis, significant attention has been directed toward the innovation of Pt and/or Pd containing nanostructures.35,36 Among the various bimetallic nanoparticles, AuPd nanoparticles have been the subject of intense research because of their excellent catalytic properties.37 Although numerous reports have been published which about the synthesis and characterization of AuPd nanoparticles, the application of these particles with defined shapes and a controlled atomic distribution have not yet been studied. In this work, the AuPd nanostructures with perpendicular pore channels was prepared by a simple wet-chemical synthesis method according to the reported method with corresponding modification as we report before.38 AuPd nanostructures with large surface area, superior conductivity, and favorable catalytic activity toward H2O2 used as coreactant which could promote the ECL performance were selected as the signal label.
Here, we report a simple and rapid paper-based ECL method for the measurement of two antigens at cells surface based on the use of CdTe QDs and luminol groups doped AuPd nanostructures as ECL probes. AuPd are chosen as label materials in this work due to its strong absorption ability, large surface area, superior conductivity, and favorable catalytic activity to H2O2. By labeling luminol and CdTe QDs groups on AFP and CEA antibodies, the ECL method was applied on the detection of the AFP and CEA antigen labeled human normal cells respectively. Afterward, the assay was applied for the quantification of AFP and CEA antigen at MCF-7 cancer cells. Combined with the paper array, the immunosensor was able to simultaneously detect multiple analytes.
2. Experimental
2.1. Reagents and apparatus
All reagents were of analytical-reagent grade or the highest purity available and directly used for the following experiments without further purification. The aqueous solutions unless indicated were prepared with ultrapure water. CEA antibody and antigen, AFP antibody and antigen were purchased from Linc-Bio Science Co. Ltd. (Shanghai, China). Bovine serum albumin (BSA) was purchased from Sigma-Aldrich Inc. (U.S.A.). 1-Ethyl-3-(3-dimethylaminopropyl) carbodiimide (EDC), and N-hydroxysuccinimide (NHS) were from Alfa Aesar China Ltd. Sodium tetrachloropalladate (Na2PdCl4, ≥99.9%), hexadecylpyridinium chloride monohydrate (C5H5N(Cl)(CH2)15CH3·H2O, HDPC), ascorbic acid (C6H8O6, AA) were all purchased from Aladdin Industrial Corporation. Chloroauric acid (HAuCl4·3H2O), sodium carbonate, ethylene glycol (EG) and trisodium citrate were obtained from Shanghai Reagent Company (Shanghai, China). 0.1 M phosphate buffer solution (PBS, pH 7.4) was employed as the supporting electrolyte. Whatman chromatography paper #1 (58.0 cm × 68.0 cm) (pure cellulose paper) was obtained from GE Healthcare Worldwide (Pudong, Shanghai, China) and used with further adjustment of size (A4 size). Carbon ink (ED423ss) and Ag/AgCl ink (CNC-01) were purchased from Acheson. The human breast cancer cells MCF-7 and human epithelial breast cell line 7610 (HMEpic) were provided by Shandong Tumor Hospital.
The ECL measurements were carried out on a MPI-E multi-functional electrochemical and chemiluminescence analytical system (Xi'an Remax Analytical Instrument Ltd. Co.) biased at 800 V. The scanning electronic microscope (SEM) images were obtained from a HITACHI S-3500 SEM (Japan). Transmission electron microscopy (TEM) was collected with a JEM-2100 high-resolution transmission electron microscope (200 kV). Electrochemical impedance spectra (EIS) were performed on a CHI 600D Electrochemical Workstation (Shanghai CH Instruments Inc., China).
2.2. Synthesis of porous bimetallic AuPd nanoparticles
The AuPd nanostructures with perpendicular pore channels was obtained by a wet-chemical method according to our previously reported method.38 Firstly, 2.0 mL of 10 mmol L−1 Na2PdCl4 solution, 0.2 mL 1% HAuCl4 solution and 0.1 g HDPC was added into a round-bottom flask and followed by adding 25 mL H2O under vigorously stirring. Then, 2 mL of 0.1 mmol L−1 freshly prepared AA solution was added quickly into the solution mentioned above with gentle shaking. After the round-bottom flask was sealed, the resulting homogeneously solution was standing for 3 h under room temperature. The obtained black precipitate could be easily isolated from the solution by centrifugation and was purified by washing several times with ultrapure water, followed by washing thoroughly with pure ethanol, and then dried in a vacuum overnight for later use.
2.3. Preparation of CEA antibodies associated CdTe@AuPd complex
To prepare the ECL probe, the mixture solution containing ethylenediamine (2 mL) and 1 mg nanoporous AuPd bimetallic nanoparticles were added into 5 mL of ethanol under sonication for 2 h. Then the resulting amino group functionalized nanoporous AuPd bimetallic nanoparticles were washed with ultrapure water and then isolated by centrifugation. Subsequently, amino group functionalized nanoporous AuPd bimetallic nanoparticles were added into the solution of CdTe QDs containing 2 mL of EDC (20 mM) for 1 h. Finally, the prepared CdTe@AuPd nanoparticles were washed with water several times and dried at 60 °C in vacuum. The CdTe@AuPd–Ab2 bioprobe integrated both the specific recognition of corresponding CEA antigen on the captured MCF-7 cell surface by antibody and amplification of ECL signal based on the CdTe@AuPd with the H2O2 as the coreactant. And the preparation of the AFP antibody–luminol@AuPd was shown in the ESI.†
2.4. Preparation of CEA and AFP labeled HMEpic cell
As for the MCF-7 cancer cells, with a diameter of about 12 μm, may cause the largely decrease in the ECL signal due to the significant impedence. So different concentrations of CEA and AFP labeled normal HMEpic cells were selected as the standard cells respectively. Firstly, CEA standard solutions with different concentration were prepared. Then, 1 mL of HMEpic cells (1.0 × 106 cells mL−1) were incubated with 1 mL of the various CEA concentrations: 0, 0.005, 0.01, 0.05, 0.1, 1.0, 5, 50, 200 (ng mL−1) with the addition of GA as a linker for amino. The same procedure was applied for the linkage of the AFP on the cells.
2.5 Fabrication of Au nanoflowers rooted PWE
Au nanoparticles (AuNPs) were synthesized by sodium citrate reduction of HAuCl4 in water. 160 mL H2O was heated to 90 °C, and then 1.6 mL HAuCl4 (1%) was added under vigorous stirring. 1.0 mL of 1% sodium citrate was rapidly added into above solution and then the solution changed color from pale-yellow to wine-red. After boiling for 15 min, the colloids were stirred for another 15 min and cooled down to room temperature. Then, the synthesized AuNPs was repeatedly washed with Milli-Q water for three times. Then, the as-prepared AuNPs seeds solution (20.0 μL) was dropped into the bare PWEs and then dried and repeated the sequence for three times. The origami paper device was equilibrated at room temperature for 1 h to optimize the surface immobilization of AuNPs seeds on cellulose fibers. After washed with water thoroughly to remove unbound AuNPs seeds, 15 μL of freshly prepared growth aqueous solution of phosphate buffer solution (0.01 M, pH 7.0) containing 0.50 mM HAuCl4 and 0.25 mM AA was applied for seeds growth was applied into the PWEs which has been modified with a layer of AuNPs seeds, respectively, and incubated at room temperature for 10 min. During the process of growth, the AuNPs seeds acted as catalysts for the reduction of AuCl4− by AA, resulting in the enlargement of the AuNPs seeds. Subsequently, the resulting Au-PWE was washed with water thoroughly to remove loosely bound Au nanoflowers. Thus a layer of dense and uniform Au nanoflowers on cellulose fibers with good conductivity were obtained, which were dried at room temperature for 20 min. Thus a layer of interconnected Au nanoflowers on cellulose fibers with good conductivity was obtained, which was dried at room temperature for 20 min.
2.6. Design and fabrication of this paper device
Wax printing was used as the pattern method in this work due to its rapid, inexpensive, and particularly well-suited for producing large lots of prototype μ-PADs. The shape (wax-patterns) of the hydrophobic barrier on the μ-PADs was designed using Adobe illustrator CS6 which was shown in Fig. S1.† A sandwich-type cell sensing platform was constructed on the PWE of our paper device, as shown in Fig. S1.† This 3D paper device comprised of two layers of paper with the same side length of 15 mm. The hydrophilic area without wax printing, which constituted the reservoir of the paper ECL detection cell, contains one circular paper cell zone with a diameter of 6.0 mm on paper A and one circular paper auxiliary zone on paper B with a diameter of 8.0 mm. The wax-printed papers was in an oven at 130 °C for 150 s to let the printed wax melt and penetrate through the paper to form the hydrophobic and insulating patterns. The wax-patterned paper A and paper B were then ready for screen-printing of electrode array on their hydrophilic zones after cooling to room temperature.
For paper A, the working electrodes containing the wires and contact pads was screen-printed in the defined area on paper A using carbon ink. One screen-printed carbon counter electrode and one screen-printed Ag/AgCl reference electrode were screen-printed in the defined area on paper B. The wax patterns around the electrodes constituted a paper cell zone which could fill with buffer solution.
2.7. ECL detection of antigens
Firstly, 10.0 μL of CEA antibodies (20 μg mL−1) were coated on Au-PWE and dried at room temperature. Subsequently, unspecific physically adsorbed antibodies were removed by washing with PBS buffer. Then the modified Au-PWE was soaked in 1% BSA solution at 4 °C for 20 min to block the possible remaining active sites against nonspecific adsorption. Then, the prepared biosensors were dried at room temperature for 20 min, followed by washing with PBS and the resulting biosensors were obtained and stored for later use. The as-prepared biosensors (designed as BSA/Ab1/Au-PWEs) were used for detection of AFP and CEA on cancer cell surface in a parallel method. To carry out the immunoreaction and ECL detections, the 3D origami ECL biosensor was firstly incubated with 10.0 μL of sample solution containing corresponding different concentrations of CEA labeled normal HMEpic cells in PBS for 50 min at room temperature. After washing with PBS, the origami ECL biosensor was incubated with 10.0 μL of CdTe/AuPd–Ab2 composites for 20 min at room temperature, followed by washing with PBS. Finally, the ECL signal of the immunosensor was triggered under the applied potential from −0.5 to −1.8 V in 0.05 M, pH 7.4 PBS with 2 mM H2O2 as coreactant and the data was recorded by MPI-B multiple-parameter chemiluminescence analytical testing system. The same procedure was applied for the detection of the AFP, and the ECL signal of the immunosensor was triggered under the applied potential from 0 to 0.5 V in 0.05 M, pH 7.4 PBS with 2 mM H2O2 as coreactant. The detection of CEA and AFP on the MCF-7 cell surface were shown in Fig. 1.
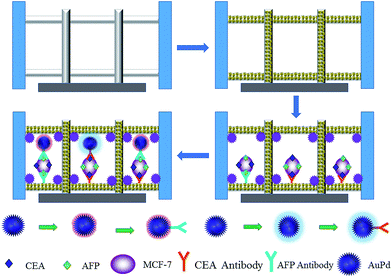 |
| Fig. 1 The fabrication process of the ECL immunosensor. | |
3. Results and discussion
3.1. Characterization of the Au-PWE and AuPd NPs
Typical SEM images of the as-prepared Au-PWE are given in Fig. 2. As shown in Fig. 2A, the porous bare paper sample with rough cellulose fibers possessed high ratio of surface area to weight, which could offer an excellent adsorption microenvironment for the AuNPs. After AuNPs were successfully seeded, a continuous and dense Au nanoflowers conducting layer on the cellulose fiber surfaces was observed (Fig. 2B–D). TEM image of the synthesized AuPd NPs was shown in Fig. 2E, which displayed that the AuPd NPs were well-defined porous nanostructures with a solid core, monodisperse and uniform in shape and size, each nanostructure surveyed was of a porous nature with highly branched subunits. The reason why these materials were selected was described in the ESI.† TEM characterization of the CdTe QDs (Fig. 2F) suggested that the as-prepared CdTe QDs had a good dispersibility and a narrow distribution of diameters in the range of 5.5–7.5 nm.
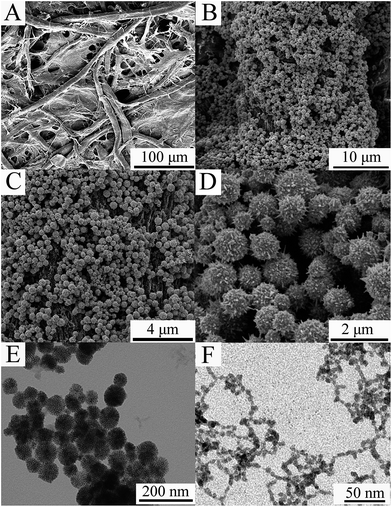 |
| Fig. 2 SEM image of (A) bare paper sample zone of PWE; SEM image of (B–D) Au nanoflower rooted PWE; TEM image of (E) AuPd nanostructure; (F) CdTe QDs. | |
3.2. Characterization of the immunosensor
As an effective tool for characterizing the interface properties of electrodes, EIS could give further information about the modification process (Fig. 3a–e). EIS were carried out in a background solution of 5 mM [Fe(CN)6]3−/4− containing 0.1 M KCl. In EIS, the diameter of the semicircle at higher frequencies corresponds to the electron-transfer resistance (Ret); a change in the value of Ret is associated with the blocking behavior of the modification processes on the electrode surface, and is reflected in the EIS as a change in the diameter of the semicircle at high frequencies.39,40 Herein, the electron-transfer of [Fe(CN)6]3−/4− onto the surface of Au-PWE was blocked by the formation of BSA/Ab1/Au-PWE, which resulted in an increase in Ret. Fig. 3A shows the EIS of PWE at different stages of treatment. Compared with the AuNPs modified PWE (curve a), the Au nanoflower layer grown on bare PWE significantly facilitated the interfacial electron transfer, exhibiting a much lower Ret in the spectrum (curve b). The Au nanoflower layer could not only enhance the electrical connectivity of the paper cell zone, but also greatly increase the surface area for Ab1 immobilization. These advantages would improve the sensitivity of antigen detection. The immobilization of the Ab1 onto Au nanoflower layer greatly increased the Ret (curve c), suggesting that Ab1 molecules were immobilized in the Au-PWE and blocked the electron exchange between the redox probe and Au nanoflower layer. After blocking with BSA, the Ret increased slightly (curve d), indicated that the residual nonspecific binding sites were blocked. Similarly, the immobilization of CEA labeled HMEpic cells independently slowed down the electron-transfer kinetics of the redox probe at the Au-PWE interface, thereby resulting in the increasing impedance of the Au-PWE (curve e), which testified to the immobilization of these substances.
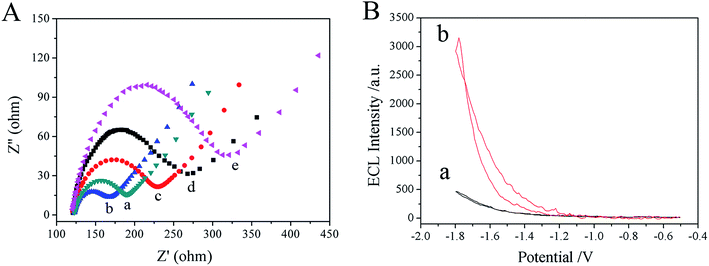 |
| Fig. 3 (A) EIS of the electrode under different condition in 5 mM [KFe(CN)6]3−/4− solution containing 0.1 M KCl: (a) AuNPs modified PWE, (b) Au-PWE, (c) Au-PWE/Ab1, (d) Au-PWE/Ab1/BSA; (e) Au-PWE/Ab1/BSA/cells; (B) ECL-potential curves of (a) no target antigens and (b) CEA (0.1 ng mL−1). | |
3.3. ECL behavior of the immunosensor
To achieve the sensitive detection of antigens at the surface of cancer cells, it is necessary to investigate the feasibility of our immunosensor. Especially, it was a concern that the CdTe@AuPd–Ab2 immobilized on the cells may be removed during multiple wash steps. Experimentally, we compared the ECL intensities obtained from immunosensors fabricated with different concentrations of target CEA-labeled HEMpic, and the results were shown in Fig. 3B. It can be observed that, when no target antigens was added, nearly no ECL signal was obtained (curve a). And when 0.1 ng mL−1 (curve b) of target CEA-labeled HEMpic were applied to fabricate immunosensors, different ECL responses were obtained. Based on the above results, we can safely draw the conclusion that our immunosensor was feasible, and the possibility that CdTe@AuPd–Ab2 may be removed during multiple wash steps can be ignored.
3.4. ECL response of the immunosensor toward CEA and AFP
To assess the analytical performance of the proposed immunosensor, the sensitivity of the prepared immunosensor was explored by evaluating different concentrations of CEA labeled HMEpic cells solution respectively including a blank one as a reference under the optimal conditions. The ECL intensity corresponding to the blank sample probably was ascribed to the physical adsorption. The ECL responses of the modified Au-PWE corresponding to CEA labeled HMEpic cell at different concentrations were shown in Fig. 4. The ECL peak intensities are proportional to the logarithmic value of the CEA concentration ranging from 0.005 to 200 ng mL−1. Calibration plots displayed a good linear relationship between the ECL intensity and the logarithm of the CEA concentration in the range of 0.005–200 ng mL−1, and the equation of linear regression was ECL = 4308.72 + 1468.67
lg
cCEA (ng mL−1), with a correlation coefficient of 0.9955 (Fig. 4A and B). The same procedure was applied for the detection of the AFP, and the equation of linear regression was ECL = 2134.87 + 559.81
lg
cAFP (ng mL−1), with a correlation coefficient of 0.9933 (Fig. 5A and B).
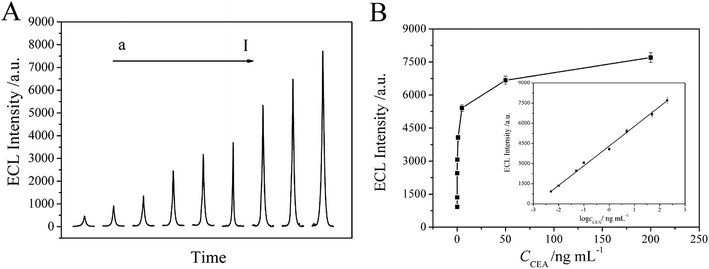 |
| Fig. 4 (A) ECL profiles of the ECL cell sensor in the presence of different concentrations of CEA used to modify normal cells in PBS containing in 0.05 M, pH 7.4 PBS with 2 mM H2O2 as coreactant. (B) Relationship between ECL intensity and CEA concentration, each point was the average of ten measurements. Insert: logarithmic calibration curve for CEA modified normal cells. | |
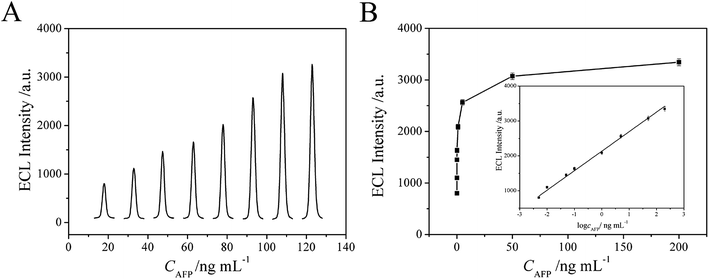 |
| Fig. 5 (A) ECL profiles of the ECL cell sensor in the presence of different concentrations of AFP used to modify normal cells in PBS containing in 0.05 M, pH 7.4 PBS with 2 mM H2O2 as coreactant (B) relationship between ECL intensity and CEA concentration, each point was the average of ten measurements. Insert: logarithmic calibration curve for AFP modified normal cells. | |
3.5. Detection of two antigens at cell surface
As shown in Fig. 5, linear relationships of ECL signal on the amount of AFP and CEA under positive and negative potentials were demonstrated. For the detection of two surface antigens at the cells surface at the same time, the cells were captured onto the Au-PWE to ensure the interaction between the antigens and antibodies. MCF-7 cancer cells with high expression of CEA and AFP antigens at the cell surface were used as a model. Using the same modification protocol, the cells (106 cells mL−1) were colabeled with AFP antibody–luminol@AuPd and CEA antibody–QDs@AuPd complexes. Fig. 6 showed the luminescence with the potential scanning from 0.5 to −1.8 V. The detection was performed in 0.1 M PBS buffer (pH 7.4), containing 2 mM H2O2. The increases in ECL under both of positive and negative potentials in Fig. 5 exhibited the existence of two antigens at the cell surface. The ECL from three groups of cells were calculated to be 0.82 fg per cell for AFP antigen and 24.69 fg per cell for CEA antigen.41,42
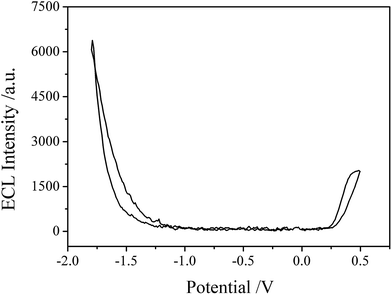 |
| Fig. 6 The luminescence curves from luminol–AFP antibody and CdTe–CEA antibody labeled MCF-7 cells (106 cells mL−1). | |
3.6. Reproducibility and stability
The reproducibility was evaluated from the ECL response of the CdTe@AuPd–Ab2/CEA/Ab1/Au-PWE. The intra-assay precision of the modified immunosensor was estimated by assaying two cell concentrations for six replicate measurements. At the CEA concentrations of 0.1 ng mL−1 and 1.0 ng mL−1, the relative standard deviations (RSDs) of intra-assay with this method were 5.0% and 4.6%, showing an acceptable precision. On the other hand, a series of six measurements from the batch resulted in a RSD of 4.3%, indicating good electrode-to-electrode reproducibility of the fabrication protocol described above. In fact, as much as 91.3% of the initial response was preserved after storage of the immunosensor at 4 °C for 30 days. The results indicated that the immunosensor exhibited satisfactory stability. This could be ascribed to the stability of the label and the immunosensor.
3.7. Possible mechanism for the ECL behavior of the system
ECL, involving a light emission process in a redox reaction of electrogenerated reactants, combines the electrochemical and luminescent techniques. It involves the production of reactive intermediates from stable precursors at the surface of an electrode. These intermediates then react under a variety of conditions to form excited state that emit light.
For such ECL system of QDs and luminol, the possible mechanism could be inferred according to previous reported literatures.43,44 In the case of the QDs–H2O2 ECL system, when the potential was cycled between −1.8 V and −0.5 V, a strong cathodic ECL signal of QD-labeled immunocomplexes was observed with an onset potential of −1.1 V.
|
H2O2 + QDs−˙ → QDs* + products
| (2) |
For the luminol–H2O2 ECL system when the potential was cycled between 0 and +0.50 V, a strong anodic ECL signal of luminol labeled immunocomplexes was observed with an onset potential at about 0.35 V. Where LH− is the deprotonated luminol and Ap2−* is 3-aminophthalate.
|
LH− − e− → LH˙ → L−˙ + H+
| (4) |
|
H2O2 + L−˙ → Ap2−* + products
| (5) |
4. Conclusions
In conclusion, based on the molecular recognition, together with the amplified property of AuPd nanostructure and the simple, rapid wax-screen-printing method, we have developed a direct, selective, and sensitive strategy for the determination of AFP and CEA antigens at the cell surface. The ECL signal from each probe was restricted in the limited potential windows so that they were used for the quantification of surface antigens at the same time. This method seems to be useful and our work will facilitate the utilization of Au-PWE in environmental chemistry, biotechnology, and medicine. Meanwhile, the sensor developed on Au-PWE in this study was very attractive for practical applications, and can be readily used for sensing other important biorecognition events.
Acknowledgements
This work was financially supported by the National Natural Science Foundation of China (21475052, 21175058, 21277058), Technology Development Plan of Shandong Province, China (Grant No. 2014GGX103012).
References
- C. F. Ding, Q. Zheng, N. N. Wang and Q. F. Yue, Anal. Chim. Acta, 2012, 756, 73–78 CrossRef CAS PubMed.
- C. Zong, J. Wu, C. Wang, H. X. Ju and F. Yan, Anal. Chem., 2012, 84, 2410–2415 CrossRef CAS PubMed.
- J. J. Zhao, L. L. Zhang, C. F. Chen, J. H. Jiang and R. Q. Yu, Anal. Chim. Acta, 2012, 745, 106–111 CrossRef CAS PubMed.
- C. Y. Hu, D. P. Yang, Z. Y. Wang, L. L. Yu, J. L. Zhang and N. Q. Jia, Anal. Chem., 2013, 85, 5200–5206 CrossRef CAS PubMed.
- C. H. Zhou, J. Y. Zhao, D. W. Pang and Z. L. Zhang, Anal. Chem., 2014, 86, 2752–2759 CrossRef CAS PubMed.
- J. J. Zhang, T. T. Zheng, F. F. Cheng, J. R. Zhang and J. J. Zhu, Anal. Chem., 2011, 83, 7902–7909 CrossRef CAS PubMed.
- M. S. Wu, D. Y. Yuan, J. J. Xu and H. Y. Chen, Anal. Chem., 2013, 85, 11960–11965 CrossRef CAS PubMed.
- H. Hatanaka, T. Yasukawa and F. Mizutani, Anal. Chem., 2011, 83, 7207–7212 CrossRef CAS PubMed.
- T. Yasukawa, H. Hatanaka and F. Mizutani, Anal. Chem., 2012, 84, 8830–8836 CrossRef CAS PubMed.
- Q. Dai, Y. Yan, C. S. Ang, K. Kempe, M. M. J. Kamphuis, S. J. Dodds and F. Caruso, ACS Nano, 2015, 9, 2876–2885 CrossRef CAS PubMed.
- E.-Q. Song, J. Hu, C.-Y. Wen, Z.-Q. Tian, X. Yu, Z.-L. Zhang, Y.-B. Shi and D.-W. Pang, ACS Nano, 2011, 5, 761–770 CrossRef CAS PubMed.
- M. S. Wu, H. W. Shi, L. J. He, J. J. Xu and H. Y. Chen, Anal. Chem., 2012, 84, 4207–4213 CrossRef CAS PubMed.
- F. Han, H. Jiang, D. Fang and D. Jiang, Anal. Chem., 2014, 86, 6896–6902 CrossRef CAS PubMed.
- H. R. Zhang, J. J. Xu and H. Y. Chen, Anal. Chem., 2013, 85, 5321–5325 CrossRef CAS PubMed.
- D. J. Lin, J. Wu, F. Yan, S. Y. Deng and H. X. Ju, Anal. Chem., 2011, 83, 5214–5221 CrossRef CAS PubMed.
- S. J. Xu, Y. Liu, T. H. Wang and J. H. Li, Anal. Chem., 2011, 83, 3817–3823 CrossRef CAS PubMed.
- J. P. Li, Q. Xu, X. P. Wei and Z. B. Hao, J. Agric. Food Chem., 2013, 61, 1435–1440 CrossRef CAS PubMed.
- A. W. Martinez, S. T. Phillips and G. M. Whitesides, Anal. Chem., 2010, 82, 3–10 CrossRef CAS PubMed.
- S. M. Wang, L. Ge, X. R. Song and J. H. Yu, Biosens. Bioelectron., 2012, 31, 212–218 CrossRef CAS PubMed.
- S. N. Tan, L. Y. Ge, H. Y. Tan, W. K. Loke, J. R. Gao and W. Wang, Anal. Chem., 2012, 84, 10071–10076 CrossRef CAS PubMed.
- S. G. Ge, L. Ge, M. Yan, X. R. Song, J. H. Yu and J. D. Huang, Chem. Commun., 2012, 48, 9397–9399 RSC.
- Z. Gu, M. X. Zhao, Y. W. Sheng, L. A. Bentolila and Y. Tang, Anal. Chem., 2011, 83, 2324–2329 CrossRef CAS PubMed.
- F. Deiss, A. Mazzeo, E. Hong, D. E. Ingber, R. Derda and G. M. Whitesides, Anal. Chem., 2013, 85, 8085–8094 CrossRef CAS PubMed.
- R. Derda, S. K. Y. Tang, A. Laromaine, B. Mosadegh, E. Hong, M. Mwangi, A. Mammoto, D. E. Ingber and G. M. Whitesides, PLoS One, 2011, 6, e18940–e18953 CAS.
- M. Funes-Huacca, A. Wu, E. Szepesvari, P. Rajendran, N. Kwan-Wong, A. Razgulin, Y. Shen, J. Kagira, R. Campbell and R. Derda, Lab Chip, 2012, 12, 4269–4278 RSC.
- A. Qi, S. P. Hoo, J. Friend, L. Yeo, Z. Yue and P. P. Y. Chan, Adv. Healthcare Mater., 2013, 3, 543–554 CrossRef PubMed.
- J. H. Yu, S. M. Wang, L. Ge and S. G. Ge, Biosens. Bioelectron., 2011, 26, 3284–3289 CrossRef CAS PubMed.
- S. G. Ge, L. Ge, M. Yan, X. R. Song and J. H. Yu, Biosens. Bioelectron., 2013, 43, 425–431 CrossRef CAS PubMed.
- M. Yan, D. J. Zang, S. G. Ge, L. Ge and J. H. Yu, Biosens. Bioelectron., 2012, 38, 355–361 CrossRef CAS PubMed.
- L. Ge, J. X. Yan, X. R. Song, M. Yan, S. G. Ge and J. H. Yu, Biomaterials, 2012, 33, 1024–1031 CrossRef CAS PubMed.
- S. W. Wang, L. Ge, Y. Zhang, X. R. Song, N. Q. Li, S. G. Ge and J. H. Yu, Lab Chip, 2012, 12, 4489–4498 RSC.
- S. Li, S. W. Wang, M. Yan, S. G. Ge, L. Ge, J. H. Yu and X. R. Song, Sens. Actuators, B, 2013, 183, 488–495 CrossRef CAS.
- M. Tsuji, K. Ikedo, M. Matsunaga and K. Uto, CrystEngComm, 2012, 14, 3411–3423 RSC.
- W. W. He, X. C. Wu, J. B. Liu, X. N. Hu, K. Zhang, S. Hou, W. Y. Zhou and S. S. Xie, Chem. Mater., 2010, 2988–2994 CrossRef CAS.
- G. R. Zhang, J. Wu and B. Q. Xu, J. Phys. Chem. C, 2012, 116, 20839–20847 CAS.
- F. H. Li, Y. Q. Guo, R. Q. Li, F. Wu, Y. Liu, X. Y. Sun, C. B. Li, W. Wang and J. P. Gao, J. Mater. Chem. A, 2013, 1, 6579–6587 CAS.
- J. Xu, A. R. Wilson, A. R. Rathmell, J. Howe, M. Chi and B. J. Wiley, ACS Nano, 2011, 5, 6119–6127 CrossRef CAS PubMed.
- S. G. Ge, F. Liu, W. Y. Liu, M. Yan, X. R. Song and J. H. Yu, Chem. Commun., 2014, 50, 475–477 RSC.
- A. Venkatanarayanan, T. E. Keyes and R. J. Forster, Anal. Chem., 2013, 85, 2216–2222 CrossRef CAS PubMed.
- C. Y. Hu, D. P. Yang, Z. Y. Wang, L. L. Yu, J. L. Zhang and N. Q. Jia, Anal. Chem., 2013, 85, 5200–5206 CrossRef CAS PubMed.
- B. J. Zeskind, C. D. Jordan, W. Timp, L. Trapani, G. Waller, V. Horodincu, D. J. Ehrlich and P. Matsudaira, Nat. Methods, 2007, 4, 567–569 CrossRef CAS PubMed.
- R. J. Grieve, K. L. Woods, P. R. Mann, S. C. H. Smith, G. D. Wilson and A. Howell, Br. J. Cancer, 1980, 42, 616–619 CrossRef CAS PubMed.
- X. F. Wang, Y. Zhou, J. J. Xu and H. Y. Chen, Adv. Funct. Mater., 2009, 19, 1444–1450 CrossRef CAS.
- W. P. Li, L. Li, S. G. Ge, X. R. Song, L. Ge, M. Yan and J. H. Yu, Chem. Commun., 2013, 49, 7687–7689 RSC.
Footnote |
† Electronic supplementary information (ESI) available. See DOI: 10.1039/c5ra26606h |
|
This journal is © The Royal Society of Chemistry 2016 |