DOI:
10.1039/C5RA25826J
(Paper)
RSC Adv., 2016,
6, 22845-22851
Giant enhancement of upconversion emission in NaYF4:Er3+@NaYF4:Yb3+ active-core/active-shell nanoparticles
Received
4th December 2015
, Accepted 18th February 2016
First published on 22nd February 2016
Abstract
Varying the type and the doping concentration of rare-earth ions was reported as a conventional method to tune upconversion emissions of rare-earth ions doped nanoparticles (NPs). In this work, an effective method to enhance greatly the infrared-visible upconversion emissions of Er3+ ions is demonstrated through coating an optimized active NaYF4:Yb3+ shell around NaYF4:Er3+ core nanoparticles. Phase, shape, and size of the resulting core–shell NPs are studied by X-ray diffraction and high-resolution transmission electron microscopy. Compared with the core-only NaYF4:Er3+ NPs, a maximum 97.56-fold overall enhancement in the emission intensity of Er3+ ions in the core–shell NPs is achieved under 980 nm infrared excitation, and a maximum 38.6-fold overall enhancement is achieved under 1540 nm infrared excitation. The luminescence enhancement effect and color output exhibits a strong dependence on the doping concentrations of Yb3+ in the NaYF4:Yb3+ active-shell. By analyzing the decay lifetimes of the emission bands, the giant luminescence enhancement is due to the significant increase in the near-infrared absorption and efficient energy transfer from Yb3+ primary-sensitizers to Er3+ activators via Yb3+ bridging sensitizers.
1. Introduction
Near 99% of the solar radiation energy in the ground solar spectrum is located in the 220–2500 nm wide emission band,1,2 where visible light (400–700 nm) and infrared light (700–2500 nm) account for 71% and 22% of the amount of solar radiation, respectively. Visible light is widely used in the lighting industry, three-dimensional displays, photovoltaic industry, biomedical imaging, and photosynthesis.3–12 However, there is a lack of applications for infrared light in above research fields. Infrared light in the solar radiation energy contains two main infrared emission bands centered at 980 nm and 1540 nm. Recently, lanthanide-doped nanoparticles (NPs) exhibited Stokes-shifting that can convert low-energy infrared excitation radiation into higher-energy visible emissions.13,14 The Yb3+ ion was reported to have wide absorption cross-section of 980 nm infrared emission in fluoride host.15,16 In order to enlarge photo-absorption cross section of 980 nm infrared light, the Yb3+ ion is chosen to be as a sensitizer for the Re3+ (Re = Er, Tm, and Ho) ions,17–22 due to the fact that the Yb3+ ion consists of only two levels, and has only one electronic excited state 2F5/2 that is located in the near infrared region about 980 nm. Moreover, high-power InGaAs diode lasers are available to directly pump the Yb3+ absorption band around 980 nm. The doping content of Yb3+ was usually kept at 18% or higher, because the large energy gap between the excited state 2F5/2 and ground state 2F7/2 blocks multiphoton cross-relaxations.13,17 However, among the rare-earth ions, only the Er3+ ion owns an excited state level that can absorb the 1540 nm infrared light. Unlike the Yb3+, absorption cross-section of the Er3+ ion for infrared 1540 nm emission is relative narrow, which limits the emission intensity in the Er3+ up-conversion process.23,24 Additionally, the doping contrition of Er3+ ion is usually low (about 2%), due to luminescence quenching induced by the cross-relaxation energy transfer between excited states.13 It is necessary to explore a new method to enhance the efficiency of up-conversion emissions of Er3+ ion from infrared to visible.
Recently, Liu and coworkers proposed the energy migration-mediated upconversion (EMU) in rare-earth ions doped NaGdF4 core–shell NPs to enhance the intensity of UC emissions from activators without proper intermediate energy levels.25 In the EMU process, four types of luminescent centers, such as sensitizer (Yb3+), accumulator (Tm3+), migratory (Gd3+) and activator (Tb3+, Eu3+, Dy3+ or Sm3+), are incorporated into four separate layers with precisely defined concentrations. Under 980 nm excitation, the higher excited state of Tm3+ is populated by the Yb3+, migrates its energy to the Gd3+, and then this energy is transferred to Tb3+, Eu3+, Dy3+ or Sm3+ ions through the core–shell interface within the migrator ions, emitting multicolor luminescence. Similarly, the EMU was found by Huang in NaYF4:Nd3+/Yb3+/Ln3+@NaYF4:Nd3+/Yb3+ (Ln = Ho, Er, Tm) and NaGdF4:Nd3+/Yb3+/Tm3+@NaGdF4:Eu3+/Tb3+ core/shell NPs.26–28 However, at present, giant luminescence enhancement has not been achieved under 1540 nm excitation. There have been few systematic theoretical and experimental studies focusing on it. In this work, we present an effective method to enhance infrared-visible upconversion emissions of Er3+ ions through synthesizing the colloidal cubic-phase NaYF4:Er3+@NaYF4:Yb3+ active-core/active-shell NPs. The giant overall enhancement in the emission intensity of Er3+ ions in core–shell NPs is achieved with the 980 nm and 1540 nm infrared laser as two excitation sources. The mechanism on interfacial energy transfer is discussed.
2. Experimental section
2.1. Materials
All chemical materials were used without further purification. The starting materials were reagent grade 99% pure NaCF3COO, 99.95% pure trifluoroacetic acid (CF3COOH), sodium carbonate (AR), 99.99% pure Y2O3, 99.99% pure Yb2O3, and 99.99% pure Er2O3. Analytical grade cyclohexane (99.5%), oleic acid (OA), oleylamine (OM), and ethanol were used. Deionized water was used throughout.
2.2. Synthesis of NaYF4:Er3+ NPs
Rare earth trifluoroacetates (RE(CF3COO)3, RE = Y, Yb, Er) were prepared at the beginning by dissolving rare earth oxides in the CF3COOH. According to the molar ratio of NaYF4:2%Er3+, a mixture of NaCF3COO, Y(CF3COO)3, and Er(CF3COO)3 powder were dissolved in 8 mL OM and 16 mL OA. Under vigorous stirring in a three-neck flask, the mixture was then heated to 120 °C under the protection of argon atmosphere and maintained at the same temperature for 30 min to remove the oxygen and residual water. At this end, the solution was totally clear with a slight yellow color. The mixture was then heated slowly to 275 °C in the presence of argon atmosphere and maintained at 275 °C for 30 min. After then, the mixture was cooled down rapidly to room temperature. Finally, NPs were then precipitated using ethanol and isolated via centrifugation for at least three times. The resulting nanocrystals were dried in vacuum at 70 °C for a minimum of 24 h.
2.3. Synthesis of NaYF4:Er3+@NaYF4:Yb3+ NPs
To prepare the core–shell NPs, 1 mmol core NPs of NaYF4:2%Er3+ were added to 5 mL cyclohexane, then using ultrasonic homogenizer to shatter the core NPs for 30 min. According to the molar ratio of NaYF4:2%Er3+@NaYF4:x%Yb3+ (x = 0.5, 1, 2, 3, 5), a mixture of NaCF3COO, Y(CF3COO)3, and Yb(CF3COO)3 powder were dissolved in 8 mL OM and 16 mL OA. After that, the totally shattered core NPs were added to the mixture solution. Under vigorous stirring in a three-neck flask, the mixture was then heated to 120 °C under the protection of argon atmosphere and maintained at the same temperature for 30 min to remove the oxygen and residual water. At this end, the solution was totally clear with a slight yellow color. Until the mixture was cooled down rapidly to room temperature, the mixture was then heated slowly to 275 °C in the presence of argon atmosphere and maintained at 275 °C for 30 min. Finally, NPs were then precipitated using ethanol and isolated via centrifugation for at least three times. The resulting nanocrystals were dried in vacuum at 70 °C for a minimum of 24 h.
Structures of the samples were investigated by X-ray diffraction (XRD) using X'TRA (Switzerland ARL) equipment provided with a Cu tube with Kα radiation at 1.54056 Å. The size and shape of the samples were observed by a JEM-2100 transmission electron microscope (JEOL Ltd., Tokyo, Japan). Luminescence spectra were obtained by the Acton SpectraPro Sp-2300 Spectrophotometer with a photomultiplier tube equipped with 980 nm and 1540 nm infrared laser as the excitation sources. The fluorescence decay curves in visible region were recorded on a FLSP920 fluorescence spectrophotometer and using a Shimidazu R9287 photomultiplier (200–900 nm) as the detectors. All measurements were performed at room temperature.
3. Results and discussion
Fig. 1 shows the TEM images of NaYF4:2%Er3+ core, NaYF4:2%Er3+@NaYF4 active-core/inactive-shell, and NaYF4:2%Er3+@NaYF4:2%Yb3+ active-core/active-shell NPs. In order to further study the structure of NPs, the histograms of size distributions of the corresponding NPs were shown in Fig. 1(d). A typical TEM image of the NaYF4:2%Er3+ core NPs in Fig. 1(a) shows that the core NPs are well dispersed hexagon and uniform in size with an average diameter of ∼12 nm. After surface modification by the NaYF4 inactive shell, the average size of the NaYF4:2%Er3+@NaYF4 core–shell NPs increased to ∼20 nm, and their shapes remain almost unchanged, as shown in Fig. 1(b) and (d). The same result for the NaYF4:2%Er3+@NaYF4:2%Yb3+ core–shell NPs is observed, which are shown in Fig. 1(c) and (d). The increase in the particle size indicates that the shell has been successfully grown around the core. Typical high-resolution TEM (HRTEM) images of the core–shell NPs in the insets reveal their highly crystalline nature and core–shell structure with the clear crystal fringes. The powder X-ray diffraction patterns of NaYF4:2%Er3+ core, NaYF4:2%Er3+@NaYF4 active-core/inactive-shell, and NaYF4:2%Er3+@NaYF4:2%Yb3+ active-core/active-shell NPs are shown in Fig. 2. The diffraction peaks of all the UCNPs can be indexed as a pure cubic NaYF4 phase (JCPDS, Card No. 06-0342.). The well defined peaks indicate the high crystallinity of the synthesized UCNPs. The diffraction peak shifts slightly to the lower-angle side is observed. The similar shifts of diffraction peaks were also observed in Mn3+–Yb3+–Er3+ doped and Li+–Yb3+–Tm3+ doped NaYF4 UCNPs,29,30 which is ascribed to decrease of the unit-cell volumes induced by the substitution of Y3+ ions by smaller other ions in the host lattice.
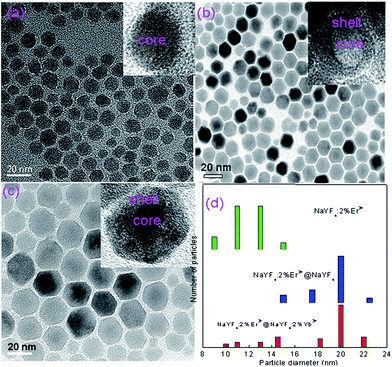 |
| Fig. 1 TEM micrographic images of (a) NaYF4:2%Er3+ core, (b) NaYF4:2%Er3+@NaYF4 active core/inactive shell, and (c) NaYF4:2%Er3+@NaYF4:2%Yb3+ active-core/active-shell. The insets are HRTEM images. (d) Histograms of size distributions of the corresponding NPs. | |
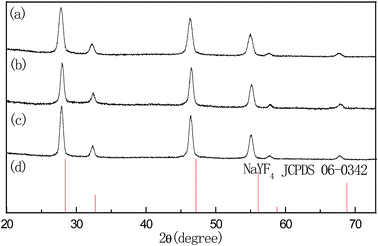 |
| Fig. 2 XRD patterns of (a) NaYF4:2%Er3+ core, (b) NaYF4:2%Er3+@NaYF4 active core/inactive shell, and (c) NaYF4:2%Er3+@NaYF4:2%Yb3+ active-core/active-shell NPs. (d) The standard data of JCPDS card No. 06-0342. | |
Fig. 3(a) shows the upconversion emission spectra of the core-only, active-core/inactive-shell and active-core/active-shell NPs under 980 nm excitation. Under 980 nm excitation, green, red, and infrared upconversion emissions centered at around 524, 543, 653 nm, and 800 nm are observed in the core-only NPs, corresponding to the 2H11/2 → 4I15/2, 4S3/2 → 4I15/2, 4F9/2 → 4I15/2, and 4I9/2 → 4I15/2 transitions of Er3+ ions, respectively. Compared with the core-only NPs, an obvious upconversion luminescence enhancement can be observed after coating the NaYF4 and NaYF4:Yb3+ shell. The enhancement of NaYF4:2%Er3+@NaYF4 active-core/inactive-shell NPs is up to 9.35 times. By changing the doping concentrations of Yb3+ in the NaYF4:Yb3+ active-shell, the largest enhancement about ∼97.56 times is observed in the NaYF4:2%Er3+@NaYF4:2%Yb3+ active-core/active-shell NPs. This giant enhancement is originated from significant increase in the near-infrared absorption and efficient energy transfer from Yb3+ primary-sensitizers to Er3+ activators via Yb3+ bridging sensitizers.13,26
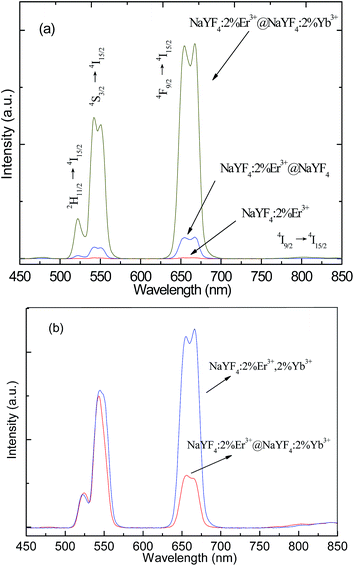 |
| Fig. 3 (a) Upconversion emission spectra of NaYF4:2%Er3+ core, NaYF4:2%Er3+@NaYF4 active-core/inactive-shell, and the NaYF4:2%Er3+@NaYF4:x%Yb3+ (x = 0.5, 1, 2, 3, 5) active-core/active-shell NPs under 980 nm excitation. (b) Upconversion emission spectra of the NaYF4:2%Er3+@NaYF4:2%Yb3+ active-core/active-shell NPs and NaYF4:2%Er3+,2%Yb3+ NPs under 980 nm excitation. | |
As reported in literature, Yb3+–Er3+ codoped NaYF4 NPs is one of the effective upconversion materials.15,31 In this paper, we study the difference between Yb3+–Er3+ codoped NaYF4 NPs and NaYF4:2%Er3+@NaYF4:2%Yb3+ active-core/active-shell NPs on their optical properties. The upconversion emission spectra of NaYF4:2%Er3+@NaYF4:2%Yb3+ and NaYF4:2%Er3+,2%Yb3+ NPs under excitation of 980 nm is shown in Fig. 3(b). From Fig. 3(b), we can see that the emission intensity of the NaYF4:2%Er3+,2%Yb3+ is much higher than that of NaYF4:2%Er3+@NaYF4:2%Yb3+ active-core/active-shell under the excitation of 980 nm. It means that energy transfer between Yb3+ and Er3+ ions in in situ substitutional Y site is more effective than energy transfer between Yb3+ and Er3+ ions in the active-core/active-shell structure.
Expect for the 980 nm infrared light, the infrared band centered at 1540 nm corresponding to the 4I15/2 → 4I13/2 transition, is an effected excitation light for the Er3+.
To gain more insight, we also study the photoluminescence of the core-only, active-core/inactive-shell and active-core/active-shell NPs under 1540 nm excitation.
Fig. 4(a) shows the upconversion emission spectra of the core-only, active-core/inactive-shell and active-core/active-shell NPs under 1540 nm excitation. Green, red, and infrared upconversion emissions centered at around 523, 542, 667, and 801 nm are observed in the core-only NPs, corresponding to the 2H11/2 → 4I15/2, 4S3/2 → 4I15/2, 4F9/2 → 4I15/2, and 4I9/2 → 4I15/2 transitions of Er3+ ions, respectively. Compared with the core-only NPs, an obvious upconversion luminescence enhancement can be observed after coating the NaYF4 and NaYF4:Yb3+ shell. The largest enhancement of NaYF4:2%Er3+@NaYF4 active-core/inactive-shell NPs is up to 11.3 times, and the largest enhancement of the NaYF4:2%Er3+@NaYF4:2%Yb3+ active-core/active-shell NPs is up to about ∼38.6 times.
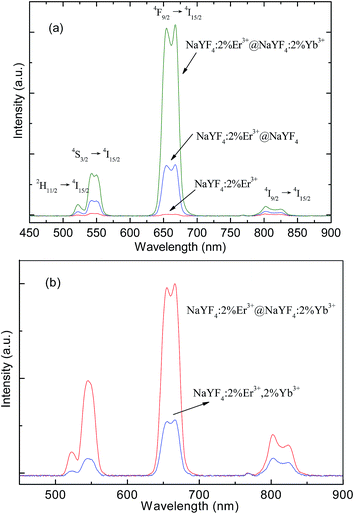 |
| Fig. 4 (a) Upconversion emission spectra of NaYF4:2%Er3+ core, NaYF4:2%Er3+@NaYF4 active-core/inactive-shell, and the NaYF4:2%Er3+@NaYF4:2%Yb3+ active-core/active-shell NPs under 1540 nm excitation. (b) Upconversion emission spectra of the NaYF4:2%Er3+@NaYF4:2%Yb3+ active-core/active-shell and NaYF4:2%Er3+,2%Yb3+ NPs under 1540 nm excitation. | |
For comparison, we also study the difference on optical properties of Yb3+–Er3+ co-doped NaYF4 NPs and NaYF4:2%Er3+@NaYF4:2%Yb3+ active-core/active-shell NPs. Upconversion emission spectra of the NaYF4:2%Er3+,2%Yb3+ NPs and NaYF4:2%Er3+@NaYF4:2%Yb3+ active-core/active-shell are given in Fig. 4(b). One can find that the emission intensity of the NaYF4:2%Er3+@NaYF4:2%Yb3+ active-core/active-shell is much stronger than that of NaYF4:2%Er3+,2%Yb3+ under 1540 nm excitation with the same excitation power. It means that the giant emission enhancement induced by the Yb3+ is more obvious in the active-core/active-shell structure than that in the substitutional doping single crystal.
In order to investigate the effect of emission enhancement induced by shell structure, the enhancement factor is calculated. The enhancement factor A is expressed as
|
A = Icore/shell/Icore,
| (1) |
where
Icore/shell and
Icore are corresponding to the emission intensity of core–shell NPs and the emission intensity of core-only NPs, respectively.
Fig. 5 shows the factors of emission intensities of NaYF
4:2%Er
3+ core, NaYF
4:2%Er
3+@NaYF
4 active-core/inactive-shell, and the NaYF
4:2%Er
3+@NaYF
4:
x%Yb
3+ (
x = 0.5, 1, 2, 3, 5) active-core/active-shell NPs under 1540 nm excitation. It can be seen that the 523 nm, 542 nm, 667 nm and 801 nm emission bands are enhanced sharply in NaYF
4:2%Er
3+@NaYF
4 active-core/inactive-shell, and the NaYF
4:2%Er
3+@NaYF
4:Yb
3+ active-core/active-shell NPs. Especially, the biggest enhancement of about 118 times for the 667 nm red emission is achieved in the NaYF
4:2%Er
3+@NaYF
4:2%Yb
3+ active-core/active-shell NPs. The emission enhancement factor is about 32 times for the 667 nm emission of NaYF
4:2%Er
3+@NaYF
4 NPs, which is much weaker than the enhancement factor of NaYF
4:2%Er
3+@NaYF
4:2%Yb
3+ NPs. The observed emission enhancement is originated from the presence of strain-induced modification of the local crystal field in the shell host lattice.
13 The Yb
3+ dependent total emission intensities of NaYF
4:2%Er
3+@NaYF
4:
x%Yb
3+ (
x = 0, 0.5, 1, 2, 3, 5) active-core/active-shell NPs are given in
Fig. 5(c). One can find that the luminescence enhancement effect exhibits the inhomogeneous increase and a strong dependence on the doping concentrations of Yb
3+ in the NaYF
4:Yb
3+ active-shell. This inhomogeneous increase of total emission intensities was also observed in NaYF
4:Nd
3+/Yb
3+/Ho
3+@NaYF
4:Nd
3+/Yb
3+ core/shell NPs under 808 nm excitation,
26 which was ascribed to the trade-off between energy transfer benefit and surface quenching effect of core–shell structures. With the increase of Yb
3+ concentration, the enhancement factor reaches the maximum values for the NaYF
4:2%Er
3+@NaYF
4:2%Yb
3+ NPs. Furthermore, the effect of the shell structures on the intensity ratio of the green/red emission (
IG/
IR) is studies in
Fig. 5(b). One can find that the
IG/
IR is tuned by varying the Yb
3+ concentration in the NaYF
4:Yb
3+ shell. The intensity of green emission is much stronger than that of red emission in the NaYF
4:2%Er
3+ core NPs, while the intensity of red emission is much stronger than that green of emission in the core–shell NPs.
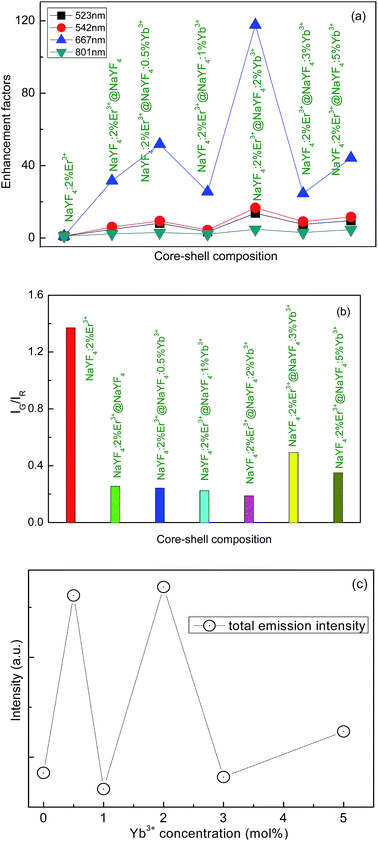 |
| Fig. 5 (a) Enhancement factors of emission intensities of core, active-core/inactive-shell, and active-core/active-shell NPs under 1540 nm excitation. (b) The effect of core–shell composition on the intensity ratio between the green and the red emissions. (c) The Yb3+ dependent total emission intensity of NaYF4:2%Er3+@NaYF4:x%Yb3+ (x = 0, 0.5, 1, 2, 3, 5) active-core/active-shell NPs. | |
To our knowledge, under 1540 nm excitation the giant enhancement of Er3+ upconversion emission induced by the active-core/active-shell construction has not been reported. Notably, the energy of the 4I11/2 level of Er3+ is almost equal to the energy of the excited state Yb3+.31 The resonant energy transfer 4I11/2 (Er3+) + 2F7/2 (Yb3+) → 4I15/2 (Er3+) + 2F5/2 (Yb3+) is possible to occur. In order to explore energy transfer from Er3+ to Yb3+, the decay behavior of 523 nm, 542 nm, 667 nm, and 801 nm emissions of NaYF4:2%Er3+ core NPs and the NaYF4:2%Er3+@NaYF4:2%Yb3+ active-core/active-shell NPs is studied in Fig. 6. These curves with non-exponential nature reveal the existence of energy transfer from Er3+ to Yb3+.32,33 The corresponding decay lifetimes can be calculated by the second order exponential curve fitting:
|
I = A1 exp(−t/τ1) + A2 exp(−t/τ2),
| (2) |
where
I is the luminescence intensity,
A1 and
A2 are constants,
t is the time,
τ1 and
τ2 are the short and long lifetimes for exponential components, respectively. The average decay lifetimes
τ are determined by the formula
|
τ = (A1τ12 + A1τ22)/(A1τ1 + A1τ2).
| (3) |
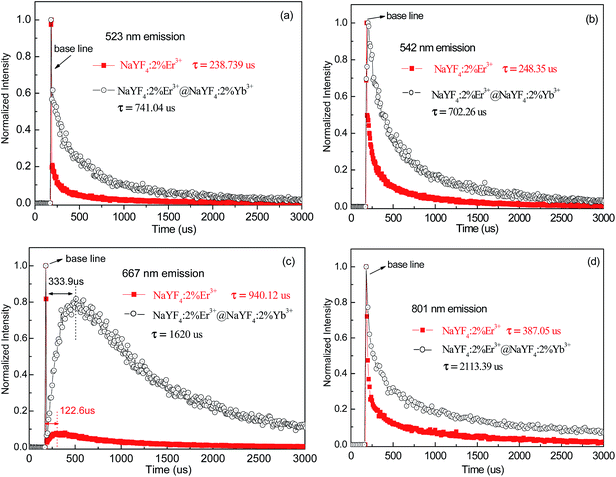 |
| Fig. 6 Decay profiles of (a) 523 nm, (b) 542 nm, (c) 667 nm, and (d) 801 nm of NaYF4:2%Er3+ core NPs and the NaYF4:2%Er3+@NaYF4:2%Yb3+ active-core/active-shell NPs under 1540 nm excitation. | |
Compared with the NaYF4:2%Er3+ core NPs, the obvious prolonged lifetimes are experimentally observed for the NaYF4:2%Er3+@NaYF4:2%Yb3+ core–shell NPs, substantiating that the excited states 4H11/2 (523 nm), 4S3/2 (542 nm), 4H11/2 (667 nm), and 4S3/2 (801 nm) are populated again through the back energy transfer from Yb3+ to Er3+.34,35 As is shown in Fig. 6, no signal rising edge is observed for the 523 nm, 542 nm, and 801 nm emissions, while the long rising edges are observed for the 667 nm emission. The excited states 4H11/2 (523 nm), 4S3/2 (542 nm), and 4S3/2 (801 nm) are populated by non-radiative relaxation from the 4F7/2 state, since no signal rising edge is observed.36 The excited state 4F9/2 (667 nm) is populated by the back energy transfer from Yb3+ to Er3+, since the long signal rising edge is observed.31 As reported in literature, the Er3+ emitted 980 nm upconversion emission under 1540 nm excitation.13 In order to analyse the energy-transfer process in NaYF4
:
Er3+@NaYF4
:
Yb3+ active-core/active-shell NPs, the 980 nm emission spectrum of NaYF4
:
Er3+@NaYF4 is given in Fig. 7. The emission intensity of 980 nm is found to decrease and then increase with increase of the Yb3+ ions, shown in the inset of Fig. 7. It means that the energy transfer from Yb3+ to Er3+occurs when the Yb3+ concentration is less than 2 mol%, while the back energy transfer from Er3+ to Yb3+ occurs when the Yb3+ concentration is more than 2 mol%.13
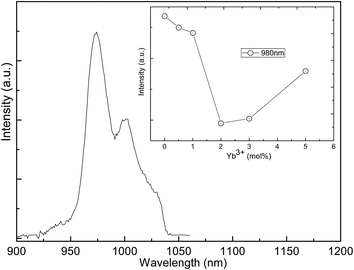 |
| Fig. 7 The 980 nm emission spectrum for NaYF4:2%Er3+@NaYF4 NPs under 1540 nm excitation. The insert shows the Yb3+ concentration dependent on the intensity of 980 nm emission in NaYF4:2%Er3+@NaYF4:x%Yb3+ (x = 0, 0.5, 1, 2, 3, 5) active-core/active-shell NPs. | |
By analyzing the decay lifetimes and spectrum of active-core/active-shell NPs, the photoluminescence mechanism is illustrated in Fig. 8. Under 1540 nm excitation, the absorption of 1540 nm photons results in direct excitation of Er3+ ions from the ground 4I15/2 state to the long-lived 4I13/2 state through a ground state absorption (GSA) process.13 Then, Er3+ ions in the 4I11/2 state are promoted to higher 4F7/2 state through two successive processes of excited state absorption. The 523 nm, 542 nm, 667 nm, 801 nm emission bands are observed through the transitions from 2H11/2, 4S3/2, 4F9/2, and 4I9/2 states to 4I15/2 state. After coating NaYF4:2%Yb3+ active-shell onto the NaYF4:2%Er3+ active-core, the back energy transfer from Er3+ (4I11/2) to Yb3+ (2F5/2) occurs, promoting the Yb3+ ion from 2F7/2 to 2F5/2. Two cross-relaxation processes between Er3+ and Yb3+, such as 2F5/2 (Yb3+) + 4I13/2 (Er3+) → 2F7/2 (Yb3+) + 4F9/2 (Er3+) and 2F5/2 (Yb3+) + 4I11/2 (Er3+) → 2F7/2 (Yb3+) + 2H11/2 (Er3+), promote the Er3+ ions in 4I13/2 and 4I11/2 states to the 4F9/2 and 2H11/2 states. An energy loop chain, Er3+ → Yb3+ → Er3+, is established to populate 2H11/2, 4S3/2, 4F9/2, and 4I9/2 states for two times through capturing non-radiative relaxation energy from the 4I13/2 and 4I11/2 states. As a result, the decay lifetimes of 2H11/2, 4S3/2, 4F9/2, and 4I9/2 states are prolonged and the corresponding emission intensities are increased sharply.
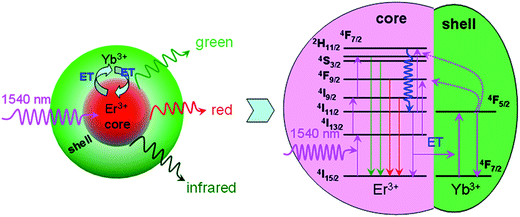 |
| Fig. 8 Schematic design of the active-core/active-shell NPs for greatly enhanced upconversion luminescence of Er3+ through the core ↔ shell two-way energy transfer under 1540 nm excitation. | |
4. Conclusion
In summary, colloidal cubic NaYF4:Er3+ core, NaYF4:Er3+@NaYF4 active-core/inactive-shell, and NaYF4:Er3+@NaYF4:Yb3+ active-core/active-shell nanoparticles were controlled by thermal decomposition of lanthanide trifluoroacetate precursors. It is found that the upconversion emission of Er3+ ions can be greatly enhanced by a factor up to 97.56-fold and 38.6-fold under 980 nm and 1540 nm excitations. Under 980 nm excitation, the luminescence enhancement effect induced by core–shell structure is lower than that induced by in situ substitutional doping of the Yb3+. Under 1540 nm excitation, the effect of core–shell structure on luminescence enhancement is prior to that induced by the substitutional Yb3+-doping. The giant enhancement of upconversion luminescence under 1540 nm excitation can be attributed to capturing non-radiative relaxation energy from the 4I13/2 and 4I11/2 states by the Yb3+ in active shell. The dependence of upconversion emission enhancement on the doping concentrations of Yb3+ ions in the active-shell layer were investigated, and the optimal doping concentrations of Yb3+ ions was found to be 2 mol%. This work provides a new paradigm for tuning luminescence intensity and color output, having implications for a range of biophotonic and infrared laser display applications.
Acknowledgements
This work was supported by National Natural Science Foundation of China (11404171), Natural Science Youth Foundation of Jiangsu Province (BK20130865), the Six Categories of Summit Talents of Jiangsu Province of China (2014-XCL-021), and the Natural Science Foundation of the Jiangsu Higher Education Institutions of China (14KJB430020).
References
- D. Q. Chen, Z. Y. Wan, Y. Zhou, W. D. Xiang, J. S. Zhong, M. Y. Ding, H. Yu and Z. G. Ji, J. Mater. Chem. C, 2015, 3, 3141–3149 RSC.
- H. F. Xiang, J. H. Cheng, X. Ma, X. Zhou and J. Joseph Chruma, Chem. Soc. Rev., 2013, 42, 6128 RSC.
- G. S. Maciel, A. Biswas, R. Kapoor and P. N. Prasad, Appl. Phys. Lett., 1978, 76, 15 CrossRef , 2000.
- G. Chen, T. Y. Ohulchanskyy, S. Liu, W. C. Law, F. Wu, M. T. Swihart, H. Agren and P. N. Prasad, ACS Nano, 2012, 6, 2969–2977 CrossRef CAS PubMed.
- C. Yuan, G. Chen, L. Li, J. A. Damasco, Z. Ning, H. Xing, T. Zhang, L. Sun, H. Zeng, A. N. Cartwright, P. Prasad and H. Agren, ACS Appl. Mater. Interfaces, 2014, 6, 18018–18025 Search PubMed.
- G. D. Farquhar and T. D. Sharkey, Annu. Rev. Plant Physiol., 1982, 33, 317–345 CrossRef CAS.
- M. M. Shang, C. X. Li and J. Lin, Chem. Soc. Rev., 2014, 43, 1372 RSC.
- X. Y. Huang, S. Y. Han, W. Huang and X. G. Liu, Chem. Soc. Rev., 2013, 42, 173 RSC.
- M. Nyk, R. Kumar, T. Y. Ohulchanskyy, E. J. Bergey and P. N. Prasad, Nano Lett., 2008, 8, 3834 CrossRef CAS PubMed.
- M. Pollnau, W. Lüthy, H. P. Weber, K. Krämer, H. U. Güdel and R. A. McFarlane, Appl. Phys. B, 1996, 62, 339 CrossRef.
- X. H. Huang and X. S. Ma, Nat. Photonics, 2014, 8, 748 CrossRef CAS.
- X. H. Huang, Opt. Lett., 2015, 40, 5231 CrossRef CAS PubMed.
- H. Dong, L. D. Sun and C. H. Yan, Chem. Soc. Rev., 2014, 44, 1608–1634 RSC.
- X. F. Wang, Q. Liu, Y. Y Bu, C. S. Liu, T. Liu and X. H Yan, RSC Adv., 2015, 5, 86219 RSC.
- J. F. Philipps, T. Töpfer, H. Ebendorff-Heidepriem, D. Ehrt and R. Sauerbrey, Appl. Phys. B, 2001, 72, 399–405 CrossRef CAS.
- V. Petit, J. L. Doualan, P. Camy, V. Ménard and R. Moncorgé, Appl. Phys. B, 2004, 78(6), 681–684 CrossRef CAS.
- J. F. Suyver, J. Grimm, M. K. Veen, D. Biner, K. W. Kramer and H. U. Gudel, J. Lumin., 2006, 117(1), 1–12 CrossRef CAS.
- R. B. Anderson, S. J. Smith, P. S. May and M. T. Berry, J. Phys. Chem. Lett., 2014, 5(1), 36–42 CrossRef CAS PubMed.
- S. Schietinger, T. Aichele, H. Q. Wang, T. Nann and O. Benson, Nano Lett., 2010, 10(1), 134–138 CrossRef CAS PubMed.
- X. M. Liu, J. W. Zhao, Y. J. Sun, K. Song, Y. Yu, C. Du, X. G. Kong and H. Zhang, Chem. Commun., 2009, 6628–6630 RSC.
- G. Y. Chen, T. Y. Ohulchanskyy, R. Kumar, H. Ågren and P. N. Prasad, ACS Nano, 2010, 4(6), 3163–3168 CrossRef CAS PubMed.
- S. Jeong, N. Won, J. Lee, J. Bang, J. Yoo, S. G. Kim, J. Ah Chang, J. Kim and S. Kim, Chem. Commun., 2011, 47, 8022–8024 RSC.
- J. N. Sandoe, P. H. Sarkies and S. Parke, J. Phys. D: Appl. Phys., 1972, 5, 1788 CrossRef CAS.
- F. Heine, E. Heumann, T. Danger, T. Schweizer, G. Huber and B. Chai, Appl. Phys. Lett., 1994, 65, 383 CrossRef CAS.
- F. Wang, R. R. Deng, J. Wang, Q. X. Wang, Y. Han, H. M. Zhu, X. Y. Chen and X. G. Liu, Nat. Mater., 2011, 10, 968–973 CrossRef CAS PubMed.
- X. Y. huang, Opt. Lett., 2015, 40, 3599 CrossRef CAS PubMed.
- X. R. Huang and J. Lin, J. Mater. Chem. C, 2015, 3, 7652 RSC.
- X. R. Huang, J. Alloys Compd., 2015, 628, 240 CrossRef CAS.
- G. Tian, et.al., Adv. Mater., 2012, 24, 1226 CrossRef CAS PubMed.
- C. Z. Zhao, et.al., Nanoscale, 2013, 5, 8084 RSC.
- J. C. Boyer, L. A. Cuccia and J. A. Capobianco, Nano Lett., 2007, 7(3), 847–852 CrossRef CAS PubMed.
- F. Pandozzi, F. Vetrone, J. Boyer, R. Naccache, J. A. Capobianco, A. Speghini and M. Bettinelli, J. Phys. Chem. B, 2005, 109, 17400–17405 CrossRef CAS PubMed.
- X. F. Wang, C. S. Liu, T. H. Yu and X. H. Yan, Phys. Chem. Chem. Phys., 2014, 16, 13440 RSC.
- M. Federighi and F. Di Pasquale, Photonics Technol. Lett., 1995, 7, 303–305 CrossRef.
- I. Etchart, A. Huignard, M. Bérard, M. N. Nordin, I. Hernández, R. J. Curry, W. P. Gillind and A. K. Cheetham, J. Mater. Chem., 2010, 20, 3989–3994 RSC.
- W. Y. Jia, K. S. Lim, H. M. Liu, Y. Y. Wang, J. J. Ju, S. I. Yun, F. E. Fernandez and W. M. Yen, J. Lumin., 1996, 190, 66–67 Search PubMed.
|
This journal is © The Royal Society of Chemistry 2016 |