DOI:
10.1039/C5RA25613E
(Paper)
RSC Adv., 2016,
6, 15854-15860
Polypyrrole-coated flower-like Pd nanoparticles (Pd NPs@PPy) with enhanced stability and heat conversion efficiency for cancer photothermal therapy†
Received
2nd December 2015
, Accepted 28th January 2016
First published on 29th January 2016
Abstract
A novel kind of flower-like Pd NPs with strong absorption in the near-infrared (NIR) region was successfully synthesized by using a seed-mediated method in aqueous solutions. The biocompatibilty of the prepared flower-like Pd NPs was improved by coating them with polypyrrole (PPy). In comparison to the pure NPs, the PPy-coated flower-like Pd NPs (Pd NPs@PPy) exhibited stronger NIR absorption, higher structural stability and lower cytotoxicity. Moreover, the photothermal conversion efficiency (η) of the Pd NPs@PPy was effectively tuned via controllably changing the thickness of the PPy shell. A η of 96.0% at 808 nm was reached when the Pd NPs@PPy with a shell of 7.0 nm was prepared. This η was higher than that of the bare flower-like Pd NPs due to the absorption of PPy in the NIR regions. In vitro photothermal heating of Pd NPs@PPy in the presence of HeLa cells led to cell destruction after 10 min of laser irradiation at a low power density of 3 W cm−2. The Pd NPs@PPy shows a potential application as a promising photothermal nanoplatform for cancer therapy.
1. Introduction
During the past decade, plasmonic nanomaterials have attracted increasing attention due to their promising applications in optical sensing of biomolecules,1 photoacoustic tomography,2 and photo-thermal therapy (PTT),3 etc.4–6 Among these biomedical applications, PTT on the basis of near-infrared (NIR) irradiation to produce significant local hyperthermia for the destruction of cancer cells has developed quickly because it is a non-invasive and harmless approach for cancer therapy. Normally, for an ideal photothermal platform, a strong NIR absorption and high photothermal conversion efficiency (η) are the keys to get a superior photothermal therapy efficacy. However, the poor bio-compatibility of the current plasmonic nanomaterials becomes the main problem of the practical photothermal nanoplatforms, which limits their applications.7–9
Up to now, types of photo-thermal agents mainly include organic compounds,10 carbon materials,11 polymers,12,13 inorganic materials,14–16 and their composites.17,18 Among these materials, anisotropic and hollow Au nanostructures are the focuses of current research due to their high optical extinction coefficients.19–21 Unfortunately, the lack of structure stability upon NIR irradiation limits their practical application as desired photothermal agents. In comparison, Pd-based nanostructures such as Pd nanosheets, which were successfully prepared and exhibited high photothermal cell-killing efficacy, possess better structure stability. Moreover, Pd-based nanostructures have strong absorption and high thermal transformation efficiency in the NIR region. Although the advantages mentioned above, the 2D ultrathin nature of the nanosheets prevents them from efficiently entering the cancer cells.22–24 Recently, it has been found that porous Pd nanoparticles (NPs) have enhanced NIR absorption compared with the solid Pd NPs and exhibit good photothermal conversion behavior.25,26 However, cetyltrimethylammonium chloride (CTAC), a surfactant with positive charges which strongly influence the cytomembrane and induce high toxicity, will inevitably be employed in the process of the porous Pd NPs synthesis, resulting in a poor biocompatibility.27 Therefore, it remains a challenge to develop Pd NPs with strong NIR-absorption, high photothermal efficiency, good structure stability, and simultaneously suitable shape as well as low toxicity for photothermal therapy.
In order to improve the biocompatibility and minimize the cytotoxicity, it is reasonable to coat the NPs with silica and/or polymer shells. For example, Zheng and co-workers enveloped Pd nanosheets with a silica shell, which simultaneously improved the biocompatibility and the entrance of the Pd nanosheets into cancer cells.24 However, the introduction of shells should be restrainedly implemented in photothermal therapy because of the possible hindrance to the heat transfer and the penetration of the electric field. Therefore, a suitable shell material with controllable thickness is the key to solve this problem. As a result, biocompatible polypyrrole (PPy) has been considered as a desirable package material due to its low cytotoxicity, high photothermal stability, low cost, easy synthesis and strong absorption in the NIR region.28–30
Herein, the well-defined flower-like Pd NPs with small crevices were successfully synthesized, which were coated with biocompatible PPy to decrease their cytotoxicity. The influence of the PPy shell thickness on the photothermal transduction was systematically investigated. As a result of contributions from the dual absorbance of Pd NPs and the PPy shell, the obtained composite possessed an enhanced photo-thermal performance, represented by the η up to 96.0%. For in vitro photothermal therapy, the composite with a 7.0 nm-PPy shell exhibited a quite high death rate of HeLa cells under 808 nm laser irradiation with relatively low power density.
2. Experimental
2.1 Materials
Pyrrole (98%, Alfa Aesar), PdCl2 (98%, Sinopharm Chemical Reagent Co., Ltd), cetyltrimethylammonium chloride (CTAC, 25% in water, Fluka), NaBH4, ascorbic acid (99%, Sigma), dodecyl sodium sulfate (SDS, 99%, Alfa Aesar), ammonium peroxydisulfate (98%, Alfa Aesar, (NH4)2S2O8), and hydrochloric acid were used as received without further purification.
2.2 Synthesis of flower-like Pd NPs
Flower-like Pd NPs were prepared through a seed-mediated method according to the protocol reported by Wang et al. with modifications.25 In briefly, 89.0 mg (0.50 mmol) PdCl2 was completely dissolved in 50 mL HCl (0.04 M) at 50 °C. The resulting solution was further diluted to 50 mL with distilled water to give the 0.01 M H2PdCl4 solutions. In a typical synthesis procedure, 0.25 mL H2PdCl4 (0.01 M) was first mixed with 9.75 mL CTAC (0.1 M). After addition of 0.6 mL freshly prepared, ice-cold NaBH4 solution (0.01 M) under vigorous stirring, the mixed solution was then kept undisturbed for 2 h at room temperature to obtain Pd seeds. For the growth of the Pd NPs, 0.025 mL seed solution was added to a mixture solution made of 9.70 mL CTAC (4.5 mM) and 0.30 mL H2PdCl4 (0.01 M). After tuning the pH of the solution to weakly acidic, 0.1 mL ascorbic acid (0.1 M) was added to the above mixture solution under magnetic stirring. After mixed for 15 s, the mixture solution was left undisturbed at room temperature for 5 h.
2.3 Ligand exchange of the flower-like Pd NPs
In a type procedure, 4 mL SDS (50 mg mL−1) was added into 10 mL of flower-like Pd NPs (100 mg mL−1) solution under stirring. The mixture was incubated at room temperature for 12 h. Then the SDS modified Pd NPs were collected by centrifugation at 18
000 rpm for 10 min, and this ligand exchange procedure was repeated 3 times. After this procedure, the SDS modified Pd NPs were re-dispersed in deionized water for further use.
2.4 PPy coated flower-like Pd NPs
Typically, 2 mL SDS (40 mM), 2 mL Py (100 mM), 10 mL 2 mM acidic (NH4)2S2O8 were added into a 100 mL of SDS modified Pd NPs (30 mg mL−1) solution under vigorous stirring. The reaction mixture was incubated at room temperature for 18 h to ensure complete polymerization. The Pd NPs@PPy was collected by centrifugation at 18
000 rpm for 10 min, and the Pd NPs@PPy composites were re-dispersed in deionized water to achieve the suspension with a specific concentration (Scheme 1).
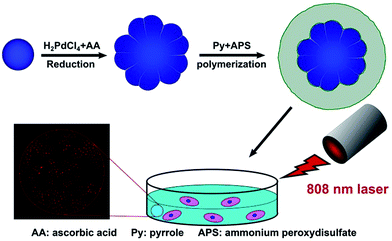 |
| Scheme 1 Schematic illustration of the synthesis of Pd NPs@PPy and photothermal therapy of cancer cells. | |
2.5 Characterization
UV-visible absorption spectra were measured using a Shimadzu UV-3600 a Shimadzu UV-2550 UV-vis-NIR spectrophotometer using quartz cuvettes with an optical path of 1 cm at room temperature. All of the solvent used in measuring the absorption spectrum is water. Transmission electron microscope (TEM) measurements were carried out on a Hitachi H-800 electron microscope at an acceleration voltage of 200 kV with a CCD camera. The high-resolution (HR) TEM (HRTEM) images were obtained by using a JEM-2100F electron microscope at 300 kV. The X-ray diffraction (XRD) data was obtained for 5 mg of Pd NPs on quartz substrates using a Siemens D5005 diffractometer. For measuring the photothermal conversion performances of the Pd NPs and PPy coated Pd NPs, 808 nm NIR diode laser (LEO photonics Co. Ltd, LE-LS-808-10000 TFC) and 660 nm NIR diode laser (LEO photonics Co. Ltd, LE-LS-660-3000 TFC) were employed with a laser spot of 1 cm2.
2.6 Photothermal tests
For measuring the photo-thermal conversion performances, a 2 mL aqueous suspension of the flower-like Pd NPs or Pd NPs@PPy with specific concentrations was added into a 1 × 1 × 4 cm quartz cuvette cell. A NIR diode laser was delivered through the quartz cuvette cell with specific power density and duration. The temperature variation was recorded one time per 30 s by a thermometer with an accuracy of ±0.1 °C (Fisher Scientific 14-648-12).
2.7 Cytotoxicity assay and photothermal effect
Human HeLa cell line was cultured in DMEM(H) supplemented 10% FBS at 37 °C in a humidified atmosphere containing 5% CO2. Standard methyl thiazolyl tetrazolium (MTT) assay was carried out to evaluate the cytotoxicity of the PPy-coated flower-like Pd NPs and pure Pd NPs. HeLa cells were seeded to 96-well plates at a density of 1 × 104 cells per well. After cultivated for 12 h, the cells were incubated with different concentrations of samples for 24 h. Subsequently, 20 μL MTT (5 mg mL−1) was added into the 96-well assay plate and incubated for another 4 h at 37 °C in a humidified atmosphere containing 5% CO2. Then, the cells were washed with PBS twice and the absorbance of each well was measured to calculate the cell viability. In the study of photothermal therapy, HeLa cells were seeded to 96-well plates at a density of 1 × 104 cells per well. After cultivated for 12 h, the cells were incubated with different concentrations of Pd NPs@PPy for 24 h. Then the as-prepared nanomaterial colloid solution with specific concentrations was added to the wells and incubated for another 24 h at 37 °C. Subsequently, the cells in each well were exposed to 808 nm laser with different power for 10 min. Then, the cell viability was measured by MTT assay as described above. After laser irradiation, the cells were stained with ethidium bromide (EB) to directly observe the cell viability and fluorescence images of live and dead HeLa cells after irradiation were obtained by fluorescence microscopy.
3. Results and discussion
3.1 Synthesis and characterization of PPy coated flower-like Pd NPs
Flower-like Pd NPs were synthesized following a seed-mediated method according to the protocol reported by Wang et al. with modifications.25 The binding of ammonium surfactants to metals is attributed to the adsorption of halide anions to the noble metal. When Cl− ions are present, they produce flower-like Pd NPs with many small crevices. The growth of flower-like Pd NPs is carried out in a faintly acidic solution to prevent oxidative etching owing to the presence of Cl− and O2 in an alkaline environment, which will give the porous structure with a bigger pore size and cause the reducing of the production yield. Fig. 1a and b give the TEM images of the prepared flower-like Pd NPs. It is observed that the Pd NPs are flower-like with many small crevices. The average size of Pd NPs is 22 ± 2 nm (Fig. 1c). As shown in Fig. 1d, although the surface plasmon resonance of the flower-like Pd NPs is not located in the vis-NIR region, the Pd NPs exhibit strong NIR absorption, which allows these Pd NPs to be a potential photo-thermal platform. In addition, compared with the solid or porous Pd NPs, the flower-like Pd NPs with enhanced extinction in NIR region are more suitable for their application in cancer treatment (Fig. S1†).
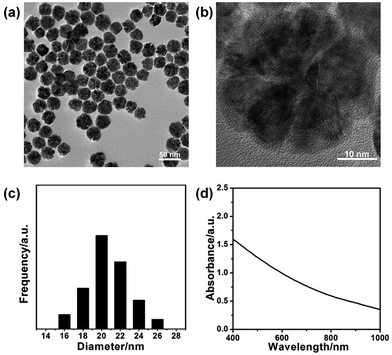 |
| Fig. 1 (a and b) TEM images of the flower-like Pd NPs at different magnifications. (c) Size distribution of the flower-like Pd NPs. (d) UV-vis-NIR absorption spectra of the flower-like Pd NPs at the concentration of 50.0 μg mL−1. | |
HRTEM and selected area electron diffraction (SAED) were used to examine the crystalline structure of the flower-like Pd NPs (Fig. 2). As illustrated in Fig. 2a, the detected lattice fringes have inter-planar distances of 0.23 nm and 0.19 nm, which correspond to (111) and (200) crystal planes of Pd NPs, respectively. The SAED pattern of flower-like Pd NPs confirms the cubic phase of Pd crystals. Fig. 2c shows XRD pattern of the flower-like Pd NPs. The peaks appeared at 40.1°, 46.6°, 68.0°, 82.1° and 86.4° are fitting well to the characteristic reflections of crystalline Pd, indicating the high crystallinity of the prepared flower-like Pd NPs.
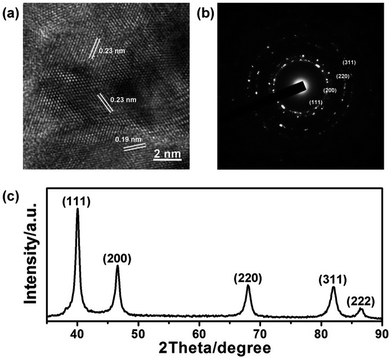 |
| Fig. 2 (a) HRTEM image, (b) SAED pattern, (c) and XRD pattern of the flower-like Pd NPs. | |
To improve the structural stability, photo-thermal conversion efficiency and biocompatibility, the flower-like Pd NPs were coated with PPy through oxidative polymerization using (NH4)2S2O8 as the initiator. To be likely responsible for the greater PPy deposition on Pd NPs, the ξ potential of the Pd NPs should turn to be negative charged by using a ligand exchange method (Fig. S2†). The thickness of the PPy shell can be controlled by changing the pyrrole content. Fig. 3a–d show the TEM images of Pd NPs@PPy in which the thickness of the PPy shell gradually increases from 2 to 12 nm with increasing the amounts of Py from 0.5 to 3 mL. Meanwhile, the crystal structure of Pd NPs has been retained well after PPy coating (Fig. 3f).
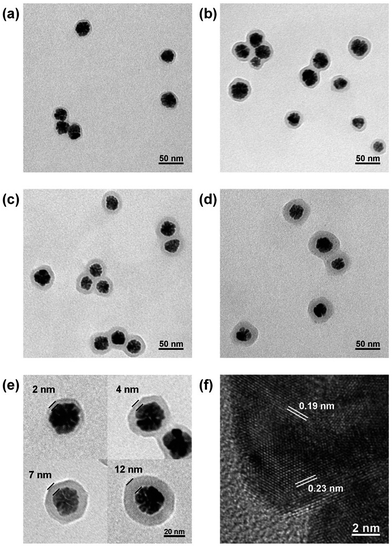 |
| Fig. 3 TEM images of Pd NPs@PPy that are prepared through addition of (a) 0.5, (b) 1, (c) 2, (d) 3 mL of 100 mM Py, (e) and the contrast image at a higher magnification of them. (f) HRTEM image of the Pd NPs after PPy modified. | |
3.2 Photothermal property of Pd NPs@PPy
In addition to the thickness of the PPy shell, the plasmon absorption of Pd NPs@PPy at 808 nm increases with increasing the pyrrole content (Fig. 4a). The stronger absorption presents advantage to the application for cancer photothermal therapy. With increasing PPy thickness, the molar extinction coefficient of Pd NPs at 808 nm improved (Table S1†) from 1.67 × 1011 to 2.55 × 1011 M−1 cm−1. Furthermore, the photo-thermal behavior of flower-like Pd NPs and Pd NPs@PPy are compared in Fig. 4b. When flower-like Pd NPs and Pd NPs@PPy with a fixed Pd NPs concentration of 50 μg mL−1 are irradiated by a 4 W cm−2 808 nm NIR laser, the temperature abruptly increases. The Pd NPs@PPy with thickness of 7 nm (7 nm-Pd NPs@PPy) exhibits the most excellent photothermal behavior with a temperature increment of 62.3 °C. Therefore, it is selected as photothermal agent for the later study. Fig. 4c–f show the effect of the concentration and laser power on the temperature for flower-like Pd NPs with a 7.0 nm PPy shell. With increasing the 7 nm-Pd NPs@PPy concentration, an obvious temperature increment is observed for the irradiation with an 808 nm laser (Fig. 4c). After 10 min irradiation, the temperature of the 50 μg mL−1 7 nm-Pd NPs@PPy increases by 62.0 °C, while that of the 15 μg mL−1 7 nm-Pd NPs@PPy, by 21 °C. As a result of contributions from the collective heating effect of the 7 nm-Pd NPs@PPy with high concentration, more irradiation is absorbed and converted into heat energy by the 7 nm-Pd NPs@PPy with high concentration, represented by the superior photothermal effect. Fig. 4d shows that the increased power density of laser also makes the temperature increment more obvious. This is attributed to high dose of irradiation imposed into the 7 nm-Pd NPs@PPy. Furthermore, a 660 nm laser also makes the solution temperature increase (Fig. 4e and f) because the 7 nm-Pd NPs@PPy possesses broadband absorption in the NIR region from 650 to 980 nm.
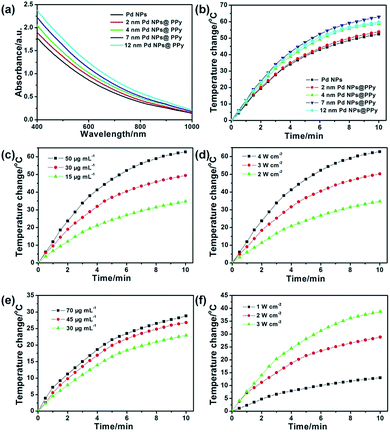 |
| Fig. 4 (a and b) UV-vis-NIR absorption spectra and photothermal heating curves of Pd NPs and Pd NPs@PPy with different PPy thicknesses. (c–f) Photothermal heating curves of the 7 nm-Pd NPs@PPy. (c) Different concentrations under a 4 W cm−2, 808 nm laser. (d) Different power densities of 808 nm laser at the concentration of 50.0 μg mL−1. (e) Different concentrations under a 2 W cm−2, 660 laser. (f) Different power densities of 660 nm laser at the concentration of 50.0 μg mL−1. | |
Following the method developed by Roper and co-workers,31 the photothermal conversion efficiency η of flower-like Pd NPs and Pd NPs@PPy was calculated according to eqn (1) (Fig. 5 and the calculation process is given in the ESI†):
|
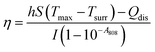 | (1) |
where
h is heat transfer coefficient,
S is the surface area of the container,
Tmax is the equilibrium temperature,
Tsurr is the temperature of the environment, and
Qdis represents the heat generated by the container itself.
I is incident laser power (4 W cm
−2), and
A808 is the absorbance of NPs at 808 nm. Thus, by using the
eqn (1), the
η values of different PPy shell thicknesses were obtained and the results were listed in Table S2.
† The values agree well with the measured temperature increment as shown in
Fig. 4b. These results indicate that a thin shell of PPy has a positive effect on improving the photo-thermal effect by combining the effects of both flower-like Pd NPs and PPy. The increase of the photo-thermal transduction is attributed to the secondary absorption of the scattered light from Pd core by PPy, which improves the efficiency in light harvesting. However, further increases cannot enhance the photothermal transduction, because thicker PPy shell may compete with the core in terms of NIR absorption.
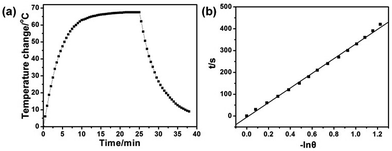 |
| Fig. 5 (a) The temporal temperature variation of 2 mL aqueous solution of flower-like Pd NPs or Pd NPs@PPy. The amount of Au is 100 μg. The solution is irradiated for 25 min using a 4 W cm−2 808 nm laser, and cooled to room temperature under ambient environment. (b) Time constant for heat transfer from the system is determined to be τs = 300 s by applying the linear time data from the cooling period (after 25 min) versus negative natural logarithm of driving force temperature. | |
In addition to the strong NIR absorption and high photothermal conversion efficiency (η), photothermal stability is also very important for photothermal agents. Fig. 6a and b indicate the photothermal stability of Pd NPs@PPy. After five cycles of heating and cooling under a 4 W cm−2 808 nm laser irradiation, UV-vis spectra exhibit only slight decrease in the NIR region and the final temperature elevation, indicating good photothermal stability of Pd NPs@PPy.
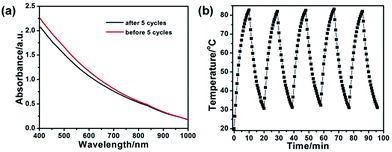 |
| Fig. 6 (a) UV-vis-NIR absorption spectra before and after irradiation, and (b) real-time temperature record of 50.0 μg mL−1 nanocomposite solution that are irradiated by a 4 W cm−2, 808 nm laser from room temperature to top temperature for 5 cycles. | |
3.3 In vitro photothermal therapy
Before applying these NPs for photothermal therapy of cancer cells, we first performed standard MTT assay to examine the cytotoxicity of 7 nm-Pd NPs@PPy. HeLa cells were seeded to 96-well plates at a density of 1 × 104 cells per well. After cultivated for 12 h, different amounts of 7 nm-Pd NPs@PPy were added to wells containing HeLa cells. As displayed in Fig. 7, upon incubation with 7 nm-Pd NPs@PPy in the range of 0–50 μg mL−1 for 24 h, the cellular viability of HeLa cells still maintained above 80%, indicating low cell-cytotoxicity and good biocompatibility of 7 nm-Pd NPs@PPy.
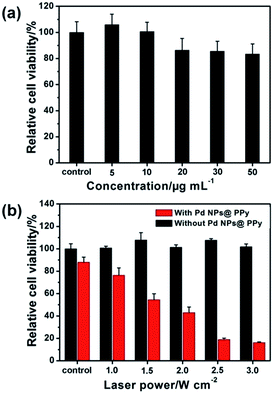 |
| Fig. 7 (a) Cytotoxicity evaluation of 7 nm-Pd NPs@PPy, which is tested by incubating HeLa cells with different concentrations of 7 nm-Pd NPs@PPy for 24 h. (b) HeLa cells viabilities under 808 nm laser irradiation with different power densities for 10 min in 100 μL of culture medium with and without 50 μg mL−1 7 nm-Pd NPs@PPy. | |
The in vitro photothermal effect of 7 nm-Pd NPs@PPy upon HeLa cells were studied by comparing the viabilities of HeLa cells under NIR laser irradiation in the presence of 7 nm-Pd NPs@PPy. After 12 h of incubation of HeLa with 50 μg mL−1 7 nm-Pd NPs@PPy solution, the cells were exposed to 808 nm laser with different power for 10 min. The cell viability after laser irradiation is exhibited by MTT assay. An obvious viability decrease with increasing irradiation intensity is found. When the intensity exceeds 2 W cm−2, the viability of the HeLa cells in the presence of 7 nm-Pd NPs@PPy is less than 40% (Fig. 7b). The cell viability further decreases to 15%, when the power density increases to 3 W cm−2 (η of 95.7%). In contrast, the 808 nm irradiation doesn't obviously suppress the viability of HeLa cells in the absence of 7 nm-Pd NPs@PPy. It is well known that heating cells between 41 and 47 °C results in cell destruction. The temperature of 7 nm-Pd NPs@PPy with NIR irradiating quickly increases higher than 41 °C (Fig. 4d), thus promoting the destruction of HeLa cells.
After NIR laser irradiating, the cells are also stained with EB, which penetrates dead cells only. Fig. 8 exhibits the staining of HeLa cells, which allows to directly observe the photothermal effect. The results clearly indicate that the HeLa cells exposed to NIR laser in the presence of composite NPs emit strong fluorescence, whereas slight fluorescence is observed for the HeLa cells in the absence of composite NPs. This indicates that the photothermal effect of 7 nm-Pd NPs@PPy leads to the of HeLa cells destruction.
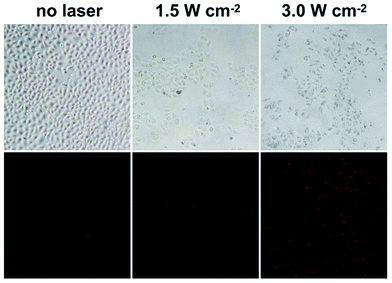 |
| Fig. 8 Bright field and fluorescent images of HeLa cells after 808 nm laser irradiation with different power densities for 10 min in 100 μL of culture medium with 50 μg mL−1 7 nm-Pd NPs@PPy. | |
4. Conclusions
In summary, the photothermal materials composed of PPy and flower-like Pd NPs have been successfully fabricated for NIR photothermal therapy of cancer. The as-prepared 7 nm-Pd NPs@PPy exhibited an ultra-high photothermal transduction efficiency (96%) and good structural stability. The nanocomposites also had broadband and strong optical absorption in NIR region. Meanwhile, in vitro cytotoxicity and photothermal effect examinations demonstrated the 7 nm-Pd NPs@PPy possessed low cytotoxicity and were effective for photothermal killing cancer cells. Owing to their good biocompatibility, photostability, and excellent photothermal properties, this nanocomposite can be used as an excellent nanoplatform for photothermal cancer therapy, which encourages further explorations of organic/inorganic nanocomposites for applications in cancer therapy.
Acknowledgements
This work was supported by the NSFC (21574018 and 51433003), the Science Technology Program of Jilin Province (20130204025GX), Jilin Provincial Education Department (543) and Jilin Provincial Key Laboratory of Micro-Nano Functional Materials (Northeast Normal University).
Notes and references
- K. Z. Kamali, A. Pandikumar, G. Sivaraman, H. N. Lim, S. P. Wren, T. Sun and N. M. Huang, RSC Adv., 2015, 5, 17809 RSC.
- X. Yang, S. E. Skrabalak, Z.-Y. Li, Y. Xia and L. V. Wang, Nano Lett., 2007, 7, 3798 CrossRef CAS PubMed.
- C. Loo, A. Lin, L. Hirsch, M.-H. Lee, J. Barton, N. Halas, J. West and R. Drezek, Technol. Cancer Res. Treat., 2004, 3, 33 CrossRef CAS PubMed.
- T. K. Sau, A. L. Rogach, F. Jäckel, T. A. Klar and J. Feldmann, Adv. Mater., 2010, 22, 1805 CrossRef CAS PubMed.
- E. Petryayeva and U. J. Krull, Anal. Chim. Acta, 2011, 706, 8 CrossRef CAS PubMed.
- R. Jiang, B. Li, C. Fang and J. Wang, Adv. Mater., 2014, 26, 5274 CrossRef CAS PubMed.
- P. K. Jain, X. Huang, I. H. El-Sayed and M. A. El-Sayed, Acc. Chem. Res., 2008, 41, 1578 CrossRef CAS PubMed.
- M. P. Melancon, M. Zhou and C. Li, Acc. Chem. Res., 2011, 44, 947 CrossRef CAS PubMed.
- V. Ntziachristos, J. Ripoll, L. V. Wang and R. Weissleder, Nat. Biotechnol., 2005, 23, 313 CrossRef CAS PubMed.
- R. Bardhan, W. Chen, C. Perez-Torres, M. Bartels, R. M. Huschka, L. L. Zhao, E. Morosan, R. G. Pautler, A. Joshi and N. J. Halas, Adv. Funct. Mater., 2009, 19, 3901 CrossRef CAS.
- F. F. Zhou, S. N. Wu, B. Y. Wu, W. R. Chen and D. Xing, Small, 2011, 7, 2727 CrossRef CAS PubMed.
- J. Yang, J. Choi, D. Bang, E. Kim, E. K. Lim, H. Park, J. S. Suh, K. Lee, K. H. Yoo, E. K. Kim, Y. M. Huh and S. Haam, Angew. Chem., Int. Ed., 2011, 50, 441 CrossRef CAS PubMed.
- K. Yang, H. Xu, L. Cheng, Y. C. Sun, J. Wang and Z. Liu, Adv. Mater., 2012, 24, 5586 CrossRef CAS PubMed.
- X. Huang, I. El-Sayed, W. Qian and M. El-Sayed, J. Am. Chem. Soc., 2006, 128, 2115 CrossRef CAS PubMed.
- J. Chen, D. Wang, J. Xi, L. Au, A. Siekkinen, A. Warsen, Z. Li, H. Zhang, Y. Xia and X. Li, Nano Lett., 2007, 7, 1318 CrossRef CAS PubMed.
- Q. Tian, F. Jiang, R. Zou, Q. Liu, Z. Chen, M. Zhu, S. Yang, J. Wang, J. Wang and J. Hu, ACS Nano, 2011, 5, 9761 CrossRef CAS PubMed.
- D. K. Lim, A. Barhoumi, R. G. Wylie, G. Reznor, R. S. Langer and D. S. Kohane, Nano Lett., 2013, 13, 4075 CrossRef CAS PubMed.
- C. Wang, H. Xu, C. Liang, Y. M. Liu, Z. W. Li, G. B. Yang, H. Cheng, Y. G. Li and Z. Liu, ACS Nano, 2013, 7, 6782 CrossRef CAS PubMed.
- C. Loo, A. Lin, L. Hirsch, M.-H. Lee, J. Barton, N. Halas, J. West and R. Drezek, Technol. Cancer Res. Treat., 2004, 3, 33 CrossRef CAS PubMed.
- H. Yuan, A. M. Fales and T. Vo-Dinh, J. Am. Chem. Soc., 2012, 134, 11358 CrossRef CAS PubMed.
- G. von Maltzahn, J. H. Park, A. Agrawal, N. K. Bandaru, S. K. Das, M. J. Sailor and S. N. Bhatia, Cancer Res., 2009, 69, 3892 CrossRef CAS PubMed.
- X. Q. Huang, S. H. Tang, X. L. Mu, Y. Dai, G. X. Chen, Z. Y. Zhou, F. X. Ruan, Z. L. Yang and N. F. Zheng, Nat. Nanotechnol., 2011, 6, 28 CrossRef CAS PubMed.
- X. Q. Huang, S. H. Tang, B. J. Liu, B. Ren and N. F. Zheng, Adv. Mater., 2011, 23, 3420 CrossRef CAS PubMed.
- S. H. Tang, X. Q. Huang and N. F. Zheng, Chem. Commun., 2011, 47, 3948 RSC.
- F. Wang, C. Li, L.-D. Sun, C.-H. Xu, J. Wang, J. C. Yu and C.-H. Yan, Angew. Chem., Int. Ed., 2012, 51, 4872 CrossRef CAS PubMed.
- J. W. Xiao, S. X. Fan, F. Wang, L. D. Sun, X. Y. Zheng and C. H. Yan, Nanoscale, 2014, 6, 4345 RSC.
- V. Shanmugam, S. Selvakumar and C. S. Yeh, Chem. Soc. Rev., 2014, 43, 6254 RSC.
- Z. J. Zhang, J. Wang and C. Y. Chen, Adv. Mater., 2013, 25, 3869 CrossRef CAS PubMed.
- L. Au, D. Zheng, F. Zhou, Z. Li, X. Li and Y. Xia, ACS Nano, 2008, 2, 1645 CrossRef CAS PubMed.
- J. Li, J. S. Han, T. S. Xu, C. R. Guo, X. Y. Bu, H. Zhang, L. P. Wang, H. C. Sun and B. Yang, Langmuir, 2013, 29, 7102 CrossRef CAS PubMed.
- D. K. Roper, W. Ahn and M. Hoepfner, J. Phys. Chem. C, 2007, 111, 3636 CAS.
Footnotes |
† Electronic supplementary information (ESI) available: The absorption spectra of solid, flower-like and porous Pd NPs in NIR region, ξ potential distribution of the Pd NPs before and after ligand exchange, the molar extinction coefficient of flower-like Pd NPs and Pd NPs@PPy with different PPy thickness, calculation of the photothermal transduction efficiency, and the photothermal conversion efficiency of the Pd NPs@PPy with different PPy thicknesses. See DOI: 10.1039/c5ra25613e |
‡ These authors contributed equally to this work. |
|
This journal is © The Royal Society of Chemistry 2016 |