DOI:
10.1039/C5RA25424H
(Paper)
RSC Adv., 2016,
6, 17239-17245
Efficient pyrene-imidazole derivatives for organic light-emitting diodes†
Received
30th November 2015
, Accepted 1st February 2016
First published on 2nd February 2016
Abstract
Two light emitting materials, 9-phenyl-10-(4-(1,2,2-triphenylvinyl)phenyl)-9H-pyreno[4,5-d]imidazole (PyTPEI) and 9-phenyl-10-(4′-(1,2,2-triphenylvinyl)-[1,1′-biphenyl]-4-yl)-9H-pyreno[4,5-d]imidazolepyreneimidazole (PyPTPEI), containing pyrene, imidazole and tetraphenylethene units are synthesized with high yields. Both of them exhibit good thermal stability with decomposition temperatures of 458 °C and 474 °C, respectively. The fluorescent quantum efficiency in amorphous films are as high as 70% for PyTPEI and 63% for PyPTPEI, respectively. In particular, PyPTPEI shows blue-shifted emission due to the more twisted conformation and reduced intermolecular interactions as compared with PyTPEI. The maximum current efficiency and maximum brightness of a non-doped OLED device using PyTPEI as an active layer reaches 8.73 cd A−1 and 27
419 cd m−2, which is better than that of PyPTPEI (7.68 cd A−1 and 19
419 cd m−2).
Introduction
Over the past few decades, organic light-emitting diodes (OLEDs) have been one of the most developed fields due to their potential applications in large-area, high-resolution flat panel displays and full-color lighting sources.1 Creation of luminogens with high solid-state efficiency is very important to facilitate applications in OLEDs.2 Pyrene represents one of the most investigated small organic molecules which is best known for its high photoluminescence efficiency, good thermal stability, and promising carrier mobility.3 However, pyrene π-system suffers heavily from the aggregation-caused-quenching (ACQ) effect originating from the strong π–π interaction of pyrene, which would limit its solid-state emission to some extent.4 So far, some chemical modifications have been introduced with the aim of inhibiting molecular aggregation including the attachment of branched alkyl,5 blending with transparent polymers, etc.6,7 These processes, however, are often accompanied by severe side effects. For example, the steric effects of bulky alicyclics can twist the conformations of the chromophoric units and jeopardize the electronic conjugation in the luminophores.5 And non-conjugated polymers can dilute the luminophore density and subsequently obstruct the charge transport in electroluminescence (EL) devices.7 Alternative strategy is of great interest.
In recent year, the attachment of aggregation-induced emission (AIE) group, such as tetraphenylethylene (TPE), into the ACQ units has proved to be a promising way to provide the newly resulted luminogens with high solid-state efficiencies.8 The development of pyrene chemistry is highly position-dependent. The electrophilic substitution of pyrene is known to take place preferentially at the 1, 3, 6, and 8-positions.9–11 Thus, 1-substituted pyrenes and 1,3,6,8-tetrasubstituted pyrenes can be easily accessible by direct electrophilic substitution of pyrene which has already generated abundant luminogens.12 The 4, 5, 9, and 10-positions of the pyrene ring are very favorable to extend the aromatic system. Fortunately, these positions can be reached through the oxidation of pyrene using a mixture of ruthenium(III) chloride (RuCl3) and sodium periodate (NaIO4) in heterogeneous system, which selectively provides pyrene-4,5-dione and pyrene-4,5,9,10-tetraone, depending on the reaction temperature and the amount of oxidant used.13 On the other hand, 1,3-imidazole is a commonly used five-numbered-ring functional group for efficient light emitting materials in virtue of its amphoteric characteristics originated from the two different kinds of sp2 hybrid nitrogen atoms.14 Based on these consideration, in this work, a facile and one-pot straightforward methodology is used to prepare a novel polycyclic skeleton using pyrene and imidazole as the key units.14a,15 Tetraphenylethylene (TPE) is introduced by Pd-catalyzed Suzuki cross-coupling reaction. It is hoped that TPE will work with the pyrene-imidazole unit synergistically and cooperatively, and their melts at the molecular level will afford adducts with the combined advantages of the two components, that is, both AIE-activity and good carrier injection and transport property. Through the judicious structural design, we have succeeded in creating new pyrene-based luminogens as well as displaying good performance in OLEDs. Especially, device based on PyTPEI exhibited green emission with external quantum efficiency and current efficiency up to 3.46% and 8.73 cd A−1, respectively, which indicates great potential application in optoelectronic field.
Results and discussion
Synthesis and characterization
The synthesis process for PyTPEI and PyPTPEI were shown in Scheme 1. The synthetic formulation strategy is based on the heterocyclization of imidazole and pyrene,16 whereby pyrene-4,5-tetraone and 4-bromobenzaldehyde were applied as the starting materials to afford compound 1.15a Compounds 2a and 2b could be obtained through the boronation of (2-bromoethene-1,1,2-triyl)tribenzene and (2-(4-bromophenyl)ethene-1,1,2-triyl)tribenzene, respectively.17 And then, the targeted products were achieved by the Pd-catalyzed Suzuki cross-coupling reaction with high yields. The compounds were thoroughly characterized by NMR (1H and 13C) spectroscopy, mass spectrometry and elemental analysis. The analytical data of PyTPEI and PyPTPEI were consistent well with their proposed structure.
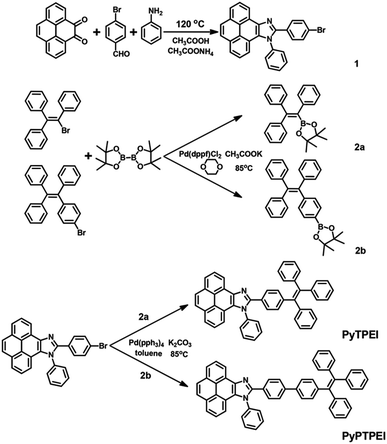 |
| Scheme 1 Synthetic routes to PyTPEI and PyPTPEI. | |
Thermal properties
The thermal stability of PyTPEI and PyPTPEI was characterized by thermal gravimetric analysis (TGA) and differential scanning calorimetry (DSC) under a nitrogen atmosphere. As shown in Fig. 1, PyTPEI and PyPTPEI exhibited excellent thermal stability with decomposition temperature (5% weight loss) at 458 °C and 474 °C, respectively. In DSC heating cycles, no Tg was detected for PyTPEI due to its rigid polycyclic skeleton, and Tm appeared at 315 °C. While Tg and Tm for PyPTPEI were at 162 °C and 283 °C respectively, which was much lower than PyTPEI demonstrating a more flexible molecular feature in PyPTPEI resulting from the additional phenyl ring between pyrene-imidazole and TPE. The rigid pyrene-imidazole moiety was responsible for their high thermostability. In addition, the exothermic peak was at 243 °C for PyTPEI and 235 °C for PyPTPEI during the thermal scanning indicating the crystalline state (Fig. 1 and S9, ESI†). The good thermal stability is very important for their applications in OLEDs.
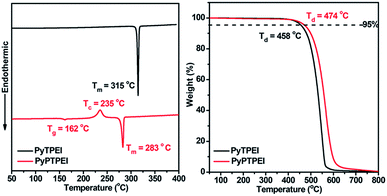 |
| Fig. 1 DSC and TGA thermograms of PyTPEI and PyPTPEI under nitrogen at a heating rate of 10 °C min−1. | |
Photophysical properties
The absorption spectra and PL emission spectra of TPE and intermediate 1 were measured in Fig. 2A and B. As can be seen from Fig. 2A, TPE show absorption at 315 nm corresponding to its π–π* transitions. However, intermediate 1 exhibits complex absorption profiles, owing to multiple localized π–π* transitions, which originated from the combination of pyrene and imidazole segment. Thus the absorption profiles of PyTPEI and PyPTPEI are similar to that of intermediate 1 which are dominated by multiple localized π–π* transitions originating from pyrene, imidazole and the TPE chromophores. The observed peak in the longer-wavelength region of 386 nm with vibrational fine structure is attributed to pyrene-imidazole-localized π–π* electronic excitation (Fig. 2C). The absorption spectra for each compound in amorphous film were very similar to that of solution, except that the absorption edges were both red-shifted about 5 nm (Fig. 2D). The optical energy gaps for PyTPEI and PyPTPEI were thus calculated to be 2.90 and 2.88 eV (Table 1), respectively. Both of them showed AIE-activity, that is, they were non-emissive in THF but exhibited intense emission in their aggregate state (Fig. S10 and S11, ESI†). Interestingly, PyTPEI showed a green emission peaking at 488 nm, whereas PyPTPEI displayed blue-shifted emission peaking at 473 nm in film although it possessed one more phenyl ring. This might be derived from the reduced intermolecular interactions in PyPTPEI because the newly formed biphenyl unit between pyrene-imidazole and TPE could rotate freely and increase the steric hindrance in solid state. The quantum efficiencies in films measured by integral sphere were as high as 70% for PyTPEI and 63% for PyPTPEI, respectively. However PLQY of intermediate 1 in film was only 9.6% with considerable bathochromic-shift (69 nm) of emission compared to that of intermediate 1 in dilute solution (Fig. 2B). In addition, the emission spectra of intermediate 1 became broad and structureless, which is typical for excimer emission.4,14a Obviously, aggregation in intermediate 1 red-shifts emission and decreases emission efficiency dramatically with notorious ACQ effect. In consequence, attaching TPE units to a pyrene-imidazole as ornaments endows the resultant products with typical AIE activity and efficient solid-state photoluminescence.
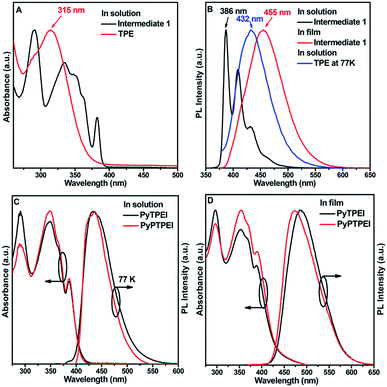 |
| Fig. 2 Normalized absorption and emission spectra of intermediate 1, TPE, PyTPEI and PyPTPEI in 2-methyltetrahydrofuran (A–C 10−5 M) and in film (B and D thickness: 30 nm). | |
Table 1 Optical and electrochemical properties of PyTPEI and PyPTPEI
Compound |
λabsa,b (nm) solu/film |
λPLa,b (nm) solu/film |
Φfc |
Egd [eV] |
Ege [eV] |
Measured in 2-methyltetrahydrofuran. Measured in solid thin film on quartz plates. Determined in evaporated film by the measurement in integrating sphere system. Optical energy bandgap (Eoptg) estimated from the absorption edge in solution. Electrochemical bandgap determined from cyclic voltammetry. |
PyTPEI |
290, 350, 386/295, 353, 388 |
433/488 |
0.70 |
2.90 |
2.99 |
PyPTPEI |
290, 348, 386/296, 354, 390 |
436/473 |
0.63 |
2.88 |
2.92 |
X-ray molecular structure and crystal packing
The single crystal of PyTPEI was obtained by the solvent evaporation method in dichloromethane. As depicted in Fig. 3, the crystal belonged to triclinic space group P
. The whole molecule formed an anti-parallel arrangement, pyrene-imidazole and TPE units alternately stacked together based on head-to-tail intermolecular interactions.18 There was no overlap between the adjacent pyrene unit which suggested that the generally observed excimer formation via π–π stackings in pyrene π-system could be avoided and accordingly eliminated the ACQ effect. It is found that there were four C–H⋯π (I, II, III, IV) interactions between hydrogen atom on phenyl rings of TPE and the π clouds of pyrene in adjacent molecules with distances of 2.73–2.99 Å, and one C–H⋯N (V) interaction between N-3 atom of imidazole and hydrogen atom on phenyl ring attaching to the N-1 atom of imidazole in adjacent molecule along b axis with a distance of 2.97 Å (Fig. S12, ESI†). The abundant intermolecular interactions helped to lock the molecular rotations of TPE in the solid state and suppress the non-radiative pathway of consuming the exciton energy to further increase the photoluminescence efficiency.
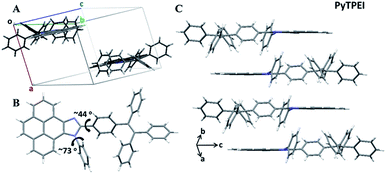 |
| Fig. 3 (A) Molecular structure of PyTPEI in one unit cell. (B) Top view. (C) Molecular arrangement along a axis, side view. | |
Theoretical calculations
To evaluate their frontier molecular orbitals and energy bandgaps, density functional theory (DFT) calculations were performed with a B3LYP/6-31G(d,p) basis set using the Gaussian 03W program (Fig. 4). The distribution of the lowest unoccupied molecular orbitals (LUMOs) and the highest occupied molecular orbitals (HOMOs) were very similar for PyTPEI and PyPTPEI. The HOMOs of the two objective compounds were mainly delocalized over the pyrene-imidazole segment, while their LUMOs were mainly located on imidazole and TPE groups. In addition, the calculated bandgaps of the compounds were nearly same which is consistent with their comparison of experimental bandgap based on optical energy bandgap and electrochemical bandgap.
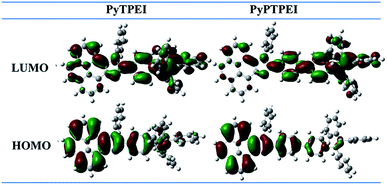 |
| Fig. 4 The HOMOs and LUMOs for PyTPEI and PyPTPEI obtained at the B3LYP/6-31G level. | |
Electrochemical properties
The electrochemical properties of these compounds were examined by cyclic voltammetry (CV). Both of them underwent irreversible oxidation and reduction process in dry dichloromethane and DMF using (Bu4N)PF6 as electrolytes under N2 atmosphere. According to the onset potentials, the HOMO levels of PyTPEI and PyPTPEI were calculated to be −5.30 and −5.36 eV, while the LUMO levels of PyTPEI and PyPTPEI were calculated to be −2.31 eV and −2.44 eV, respectively, by comparison to ferrocene (Fig. 5). The HOMO–LUMO energy gaps obtained from CV measurement coincided well with the optical energy gaps got from UV-vis measurements (Table 1).
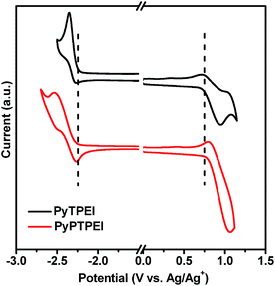 |
| Fig. 5 Cyclic voltammogram of PyTPEI and PyPTPEI in CH2Cl2 (oxidation) and DMF (reduction), measured with 0.1 M (Bu4N)PF6 as supporting electrolyte at a scan rate of 100 mV s−1. | |
Electroluminescence properties
Owing to their high solid-state efficiencies and good thermal stability, non-doped OLEDs based on PyTPEI and PyPTPEI were fabricated with a typical configuration of ITO/PEDOT:PSS (40 nm)/NPB (50 nm)/EML (20 nm)/TPBi (40 nm)/LiF (0.5 nm)/Al (120 nm), where TPBi was used as electron-transport layer and NPB was used as the hole-transport layer. The device performance was summarized in Table 2. Both devices gave green electroluminescence (EL) peaking at 500 nm with Commission Internationale de L'E' clairage (CIE) chromaticity coordinate of (0.209, 0.401) for PyTPEI and (0.202, 0.336) for PyPTPEI, respectively, where the initial band of EL spectra in PyPTPEI was blue-shifted about 20 nm compared with PyTPEI (Fig. 6A). The electroluminescent spectra were in consistent with the emission profiles of their amorphous films.
Table 2 Electroluminescence properties of the devices
Device |
Vona (V) |
CEb (cd A−1) |
EQEb (%) |
Brightnessb (cd m−2) |
ELc (nm) |
CIE (x, y)d |
Driving voltage at 1 cd m−2. Maximum value of current efficiency (CE), external quantum efficiency (EQE) and brightness. Peak wavelength of electroluminescence (EL) spectra. CIE coordinates measured at 1000 cd m−2. |
PyTPEI |
2.8 |
8.73 |
3.46 |
27 419 |
500 |
(0.209, 0.401) |
PyPTPEI |
2.8 |
7.68 |
3.35 |
19 419 |
500 |
(0.202, 0.336) |
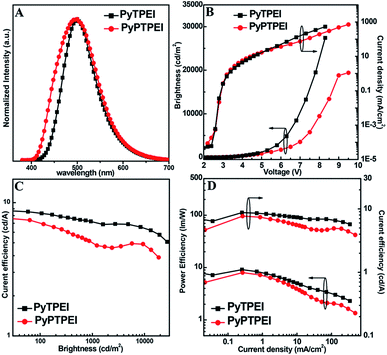 |
| Fig. 6 (A) Electroluminescence (EL) spectra for PyTPEI and PyPTPEI measured at 9 V. (B) Current density–voltage–brightness (J–V–L) characteristics. (C) Current efficiency versus brightness curves. (D) Current efficiency and power efficiency versus current density curves. | |
Fig. 6B plots the current density–luminescence–voltage (J–L–V) curves of both devices. The turn-on voltages (Von) of devices were both at 2.8 V suggesting that the dissipation of energy associated with carrier injection or transportation is quite low. The LE curves (Fig. 6D) showed a relatively stable light output, even when the current density approached 300 mA cm−2, their EL spectrum did not change in operating conditions. The PyTPEI-based device had the highest LE and external quantum efficiency (EQE) of 8.73 cd A−1 and 3.46%, respectively, yielding a maximum luminance of 27
419 cd m−2 at 8.3 V. As compared, the device using PyPTPEI as the active layer showed moderate performance with a ηc.max of 7.68 cd A−1, an EQE of 3.35% and a maximum luminance of 19
419 cd m−2. To further investigate the merits towards fusion of pyrene, imidazole and TPE subunits at molecular level and better understand the effect of 1,3-imidazole on the energy levels and the carrier injection and transport property in devices, the hole-only device with the configuration of ITO/PEDOT-PSS (40 nm)/NPB (10 nm)/EML (70 nm)/NPB (20 nm)/Al (120 nm) and the electron-only device with the configuration of ITO/TPBi (20 nm)/EML (70 nm)/TPBi (10 nm)/LiF (1 nm)/Al (120 nm) for PyTPEI and PyPTPEI were fabricated. The results (Fig. 7) indicated that both PyTPEI and PyPTPEI showed high carrier injection and transport ability which confirmed that 1,3-imidazole can act as a bipolar type building block. Their hole current values of PyTPEI and PyPTPEI were very similar and both higher than their corresponding electron current values. Furthermore, electron current values of PyTPEI was much higher than PyPTPEI, indicating that more carriers could be transported to the emitting layer of PyTPEI in OLED, which is crucial to obtain higher efficiency in PyTPEI than PyPTPEI owing to the dual-injection/transportation procedure of OLEDs.
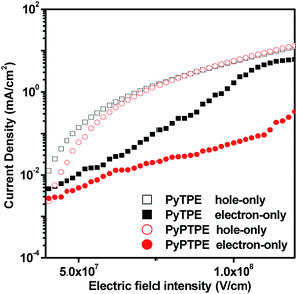 |
| Fig. 7 The electron and hole current density versus electric field intensity curves of the single carrier devices based on PyTPEI and PyPTPEI. | |
The devices based on PyTPEI and PyPTPEI will stably assemble during the device fabrication process. The amorphous films of PyTPEI and PyPTPEI were obtained through vacuum deposition method as proved by X-ray diffraction (Fig. S15, ESI†). Morphology of these films were investigated by atomic force microscopy (AFM). The images are given in Fig. 8. The PyTPEI film exhibits a quite smooth surface morphology with a roughness of 1.40 nm. After annealing at 120 °C for 1 h under ambient atmosphere, the film morphology became more smooth with a roughness of 0.74 nm. In the case of PyPTPEI, it also shows a fairly smooth surface morphology with a roughness of 0.97 nm. After annealing at 120 °C for 1 h under ambient atmosphere, the film morphology is basically unchanged. Both of them provide a homogeneous morphology of amorphous evaporated film indicating the possibility to keep uniform films throughout the device fabrication process.
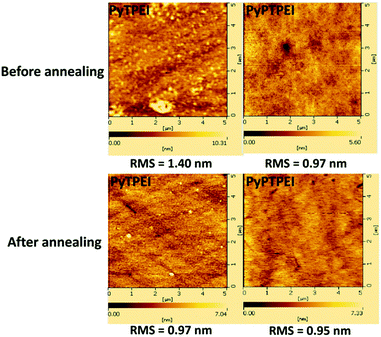 |
| Fig. 8 AFM images (5 μm × 5 μm) of evaporated films on the quartz plate before and after annealing at 120 °C for 1 h under ambient atmosphere. | |
Conclusions
In summary, we have designed and synthesized two efficient light emitting materials, PyTPEI and PyPTPEI, and determined their difference and relationship upon their molecular structure. A comparative study of these two compounds through spectroscopic, electrochemical, crystallographic, quantum calculations and OLEDs are performed. Both of them show high thermal stability. The attachment of TPE group to pyrene-imidazole endows PyTPEI and PyPTPEI with AIE-activity and high efficiencies in solid state. A blue-shifted fluorescence of PyPTPEI in the solid state is observed due to the reduced intermolecular interactions. In OLEDs, PyTPEI shows a better device performance with the maximum current efficiency of 8.73 cd A−1. Even when the brightness reached 10
000 cd m−2, the device still exhibits a very low efficiency roll-off. These molecules provides a design strategy to prevent the aggregation-induced fluorescence quenching of pyrene π system and facilitates their applications in OLEDs.
Experimental section
All of the reagents and solvents used for the syntheses were purchased from Aldrich or Acros and were used as received. All of the reactions were performed under a dry-nitrogen atmosphere. The 1H NMR spectra were recorded on an AVANCZ 500 spectrometers at 298 K by utilizing deuterated dimethyl sulfoxide (DMSO) as solvents and tetramethylsilane (TMS) as a standard. The 13C-NMR spectra were recorded on an AVANCZ 500 spectrometers at 298 K by utilizing deuterated trichloromethane as solvents and tetramethylsilane (TMS) as a standard. The final compounds were characterized by Flash EA 1112, CHNS–O elemental analysis instrument. The MALDI-TOF mass spectra were recorded using an AXIMA-CFRTM plus instrument. Diffraction data were collected on a Rigaku RAXIS-PRID diffractometer using the ω-scan mode with graphite-monochromator Mo Kα radiation. UV-vis absorption spectra were recorded on UV-3100 spectrophotometer. Fluorescence measurements were carried out with RF-5301 PC. The film PL efficiencies were measured on a Gliden Fluorescence System using an integrating sphere, with a 366.8 nm Edinburgh Instruments Ltd light emitting diode as the excitation source. Instrument model (FLS920). Thermal gravimetric analysis (TGA) was measured on a Perkin-Elmer thermal analysis system from 30 °C to 900 °C at a heating rate of 10 K min−1 under nitrogen flow rate of 80 mL min−1. Differential scanning calorimetry (DSC) was performed on a NETZSCH (DSC-204) unit from 30 °C to 400 °C at a heating rate of 10 K min−1 under nitrogen atmosphere. The electrochemical properties (oxidation and reduction potentials) were carried out via cyclic voltammetry (CV) measurements by using a standard one-compartment, three-electrode electrochemical cell given by a BAS 100B/W electrochemical analyzer. Tetrabutylammoniumhexafluorophosphate (TBAPF6) in anhydrous dimethyl formamide (DMF) or anhydrous dichloromethane (0.1 M) were used as the electrolyte for negative or positive scan. The ground-state geometries were optimized at the B3LYP/6-31G(d,p) level, which is a common method to provide molecular geometries. The HOMO/LUMO distributions are calculated on the basis of optimized ground-state.
Device fabrication: the EL devices were fabricated by vacuum deposition of the materials at 10−6 Torr onto ITO glass with a sheet resistance of 25 Ω per square. All of the organic layers were deposited at a rate of 1.0 Å s−1. The cathode was deposited with LiF (0.5 nm) at a deposition rate of 0.1 Å s−1 and then capping with Al metal (120 nm) through thermal evaporation at a rate of 4.0 Å s−1. The electroluminescent (EL) spectra and Commission Internationale De L'Eclairage (CIE) coordination of these devices were measured by a PR650 spectroscan spectrometer. The luminance–current and density–voltage characteristics were recorded simultaneously with the measurement of the EL spectra by combining the spectrometer with a Keithley model 2400 programmable voltage–current source. All measurements were carried out at room temperature under ambient conditions.
Synthesis of compound 1
A mixture of aniline (2.3 mL, 25.0 mmol), pyrene-4,5-dione (1.2 g, 5.0 mmol), 4-bromobenzaldehyde (1.2 g, 6.0 mmol), ammonium acetate (1.5 g, 20.0 mmol), and acetic acid (15 mL) was refluxed under nitrogen in an oil bath. After 2 h, the mixture was cooled and filtered. The solid product was washed with an acetic acid/water mixture (1
:
1, 50 mL). And then, it was separated by chromatography. Yield: 86.0%. MS: 472.5 (M (H+)). 1H NMR (500 MHz, DMSO-d6, 25 °C): δ 8.95 (d, J = 7.0 Hz, 1H), 8.33 (d, J = 7.4 Hz, 1H), 8.27–8.17 (m, 4H), 7.86–7.72 (m, 6H), 7.63–7.58 (m, 4H), 7.32 (d, J = 7.9 Hz, 1H).
Synthesis of PyTPEI
4,4,5,5-Tetramethyl-2-(1,2,2-triphenylvinyl)-1,3,2-dioxaborolane (2a) (0.92 g, 2.4 mmol), 10-(4-bromophenyl)-9-phenyl-9H-pyreno[4,5-d]imidazole (1) (0.95 g, 2.0 mmol), dry toluene (24 mL), and 16 mL aqueous of K2CO3 solution (2.0 mol L−1) were placed in a round-bottom flask. Pd(PPh3)4 (0.14 g, 0.12 mmol) was then added and the mixture was vigorously stirred at 90 °C for 48 h. After cooling to room temperature, the resulting mixture was extracted with dichloromethane followed by purification by column chromatography on silica gel. Yield: 80%. MS: 649.2 (M (H+)). 1H NMR (500 MHz, DMSO-d6, 25 °C): δ 8.91 (d, J = 7.6 Hz, 1H), 8.31 (d, J = 7.3 Hz, 1H), 8.25–8.17 (m, 4H), 7.78–7.71 (m, 6H), 7.41 (d, J = 8.5 Hz, 2H), 7.30 (d, J = 8.3 Hz, 1H), 7.18–7.12 (m, 9H), 7.02–6.94 (m, 8H). 13C NMR (125 MHz, CDCl3, 25 °C): δ 143.5, 143.4, 143.3, 132.2, 131.7, 131.4, 131.33, 131.29, 130.1, 129.2, 128.8, 128.0, 127.8, 127.69, 127.67, 127.5, 126.59, 126.56, 126.42, 125.3, 124.4, 124.3, 123.6, 122.9, 117.97. Elemental analysis: calculated for C49H32N2: C, 90.71; H, 4.97; N, 4.32; found: C, 90.87; H, 4.74; N, 4.39.
Synthesis of PyPTPEI
4,4,5,5-Tetramethyl-2-(4-(1,2,2-triphenylvinyl)phenyl)-1,3,2-dioxaborolane (2b) (1.10 g, 2.4 mmol), 10-(4-bromophenyl)-9-phenyl-9H-pyreno[4,5-d]imidazole (1) (0.95 g, 2.0 mmol), dry toluene (24 mL), and 16 mL aqueous of K2CO3 solution (2.0 mol L−1) were placed in a round-bottom flask. Pd(PPh3)4 (0.14 g, 0.12 mmol) was then added and the mixture was vigorously stirred at 90 °C for 48 h. After cooling to room temperature, the resulting mixture was extracted with dichloromethane followed by purification by column chromatography on silica gel. Yield: 85%. MS: 726.0 (M (H+)), 1H NMR (500 MHz, DMSO-d6, 25 °C): δ 8.96 (d, J = 7.6 Hz, 1H), 8.32 (d, J = 7.2 Hz, 1H), 8.23 (t, J = 8.6 Hz, 1H), 8.21–8.16 (m, 3H), 7.85–7.80 (m, 2H), 7.76 (dt, J = 7.9, 6.8 Hz, 4H), 7.68 (dd, J = 21.5, 8.5 Hz, 4H), 7.53 (d, J = 8.3 Hz, 2H), 7.31 (d, J = 7.8 Hz, 1H), 7.20–7.08 (m, 9H), 7.07–6.96 (m, 8H). 13C NMR (125 MHz, CDCl3, 25 °C): δ 13C NMR (126 MHz, CDCl3) δ 143.72, 143.69, 143.66, 143.3, 141.3, 140.4, 137.8, 132.2, 131.9, 131.7, 131.4, 131.34, 130.26, 130.0, 129.7, 129.2, 128.0, 127.8, 127.71, 127.65, 127.57, 126.58, 126.56, 126.52, 126.47, 126.1, 125.3, 124.6, 124.3, 123.6, 122.9, 118.0. Elemental analysis: calculated for C55H36N2: C, 91.13; H, 5.01; N, 3.86; found: C, 91.27; H, 5.14; N, 3.59.
Acknowledgements
This work was supported by the Ministry of Science and Technology of China (2013CB834801), and the National Science Foundation of China (21374038 and 91233113).
Notes and references
-
(a) C. W. Tang and S. A. VanSlyke, Appl. Phys. Lett., 1987, 51, 913 CrossRef CAS;
(b) S. R. Forrest and M. E. Thompson, Chem. Rev., 2007, 107, 923 CrossRef CAS;
(c) T. P. I. Saragi, T. Spehr, A. Siebert, T. F. Lieker and J. Salbeck, Chem. Rev., 2007, 107, 1011 CrossRef CAS PubMed;
(d) C. D. Müller, A. Falcou, N. Reckefuss, M. Rojahn, V. Wiederhirn, P. Rudati, H. Frohne, O. Nuyken, H. Becker and K. Meerholz, Nature, 2003, 421, 829 CrossRef PubMed;
(e) J. U. Park, M. Hardy, S. J. Kang, K. Barton, K. Adair, D. K. Mukhopadhyay, C. Y. Lee, M. S. Strano, A. G. Alleyne, J. G. Georgiadis, P. M. Ferreira and J. A. Rogers, Nat. Mater., 2007, 6, 782 CrossRef CAS PubMed.
-
(a) D. A. Pardo, G. E. Jabbour and N. Peyghambarian, Adv. Mater., 2000, 12, 1249 CrossRef CAS;
(b) S. R. Forrest, Nature, 2004, 428, 911 CrossRef CAS PubMed.
-
(a) T. M. Figueira-Duarte and K. Müllen, Chem. Rev., 2011, 111, 7260 CrossRef CAS PubMed;
(b) S. Bailey, D. Visontai, C. J. Lambert, M. R. Lambert, H. Frampton and D. Frampton, J. Chem. Phys., 2014, 140, 054708 CrossRef PubMed;
(c) S. Q. Zhang, X. L. Qiao, Y. Chen, Y. Y. Wang, R. M. Edkins, Z. Q. Liu, H. X. Li and Q. Fang, Org. Lett., 2014, 16, 342 CrossRef CAS PubMed.
-
(a) K.-C. Wu, P.-J. Ku, C.-S. Lin, H.-T. Shih, F.-I. Wu, M.-J. Huang, J.-J. Lin, I.-C. Chen and C.-H. Cheng, Adv. Funct. Mater., 2008, 18, 67 CrossRef CAS;
(b) J. N. Moorthy, P. Natarajan, P. Venkatakrishnan, D.-F. Huang and T. J. Chow, Org. Lett., 2007, 9, 5215 CrossRef CAS PubMed;
(c) T. Oyamada, H. Uchiuzou, S. Akiyama, Y. Oku, N. Shimoji, K. Matsushige, H. Sasabe and C. Adachi, J. Appl. Phys., 2005, 98, 074506 CrossRef;
(d) I. B. Berlman, J. Phys. Chem., 1970, 74, 3085 CrossRef CAS.
-
(a) C.-L. Chiang, S.-M. Tseng, C.-T. Chen, C.-P. Hsu and C.-F. Shu, Adv. Funct. Mater., 2008, 18, 248 CrossRef CAS;
(b) J.-S. Yang and J.-L. Yan, Chem. Commun., 2008, 1501 RSC;
(c) J. Wang, Y. F. Zhao, C. D. Dou, H. Sun, P. Xu, K. Q. Ye, J. Y. Zhang, S. M. Jiang, F. Li and Y. Wang, J. Phys. Chem. B, 2007, 111, 5082 CrossRef CAS PubMed;
(d) J. N. Moorthy, P. Natarajan, P. Venkatakrishnan, D.-F. Huang and T. J. Chow, Org. Lett., 2007, 9, 5215 CrossRef CAS PubMed.
-
(a) C. Fan, S. Wang, J. W. Hong, G. C. Bazan, K. W. Plaxco and A. J. Heeger, Proc. Natl. Acad. Sci. U. S. A., 2003, 100, 6297 CrossRef CAS PubMed;
(b) B. S. Gaylord, S. Wang, A. J. Heeger and G. C. Bazan, J. Am. Chem. Soc., 2001, 123, 6417 CrossRef CAS PubMed.
- S. Hecht and J. M. J. Fréchet, Angew. Chem., Int. Ed., 2001, 40, 74 CrossRef CAS.
-
(a) Y. N. Hong, J. W. Y. Lam and B. Z. Tang, Chem. Soc. Rev., 2011, 40, 5361 RSC;
(b) Y. N. Hong, J. W. Y. Lam and B. Z. Tang, Chem. Commun., 2009, 4332 RSC;
(c) X. F. Ji, P. Wang, H. Wang and F. H. Huang, Chin. J. Polym. Sci., 2015, 33, 890 CrossRef CAS;
(d) Y. Liu, X. Feng, J. B. Shi, J. G. Zhi, B. Tong and Y. P. Dong, Chin. J. Polym. Sci., 2012, 30, 443 CrossRef CAS.
-
(a) H. Vollmann, H. Becker, M. Corell and H. Streeck, Liebigs Ann., 1937, 531, 1 CrossRef CAS;
(b) L. Altschuler and E. Berliner, J. Am. Chem. Soc., 1966, 88, 5837 CrossRef CAS.
-
(a) M. J. S. Dewar and R. D. Dennington, J. Am. Chem. Soc., 1989, 111, 3804 CrossRef CAS;
(b) R. A. Hites, Calculated Molecular Properties of Polycyclic Aromatic Hydrocarbons, Elsevier, New York, 1987 Search PubMed.
- A. Miyazawa, T. Yamato and M. Tashiro, Chem. Express, 1990, 5, 381 CAS.
-
(a) Z. J. Zhao, S. M. Chen, J. W. Y. Lam, P. Lu, Y. C. Zhong, K. S. Wong, H. S. Kwok and B. Z. Tang, Chem. Commun., 2010, 46, 2221 RSC;
(b) Z. J. Zhao, P. Lu, J. W. Y. Lam, Z. M. Wang, C. Y. K. Chan, H. H. Y. Sung, I. D. Williams, Y. G. Ma and B. Z. Tang, Chem. Sci., 2011, 2, 672 RSC;
(c) Z. Q. Liang, Z. Z. Chu, D. C. Zou, X. M. Wang and X. T. Tao, Org. Electron., 2012, 13, 2898 CrossRef CAS.
- J. Hu, D. Zhang and F. W. Harris, J. Org. Chem., 2005, 70, 707 CrossRef CAS PubMed.
-
(a) Y. L. Liu, Z. Gao, Z. M. Wang, C. F. Feng, F. Z. Shen, P. Lu and Y. G. Ma, Eur. J. Org. Chem., 2013, 32, 7267 CrossRef;
(b) W. J. Li, L. Yao, H. C. Liu, Z. M. Wang, S. T. Zhang, R. Xiao, H. H. Zhang, P. Lu, B. Yang and Y. G. Ma, J. Mater. Chem. C, 2014, 2, 4733 RSC;
(c) Z. M. Wang, P. Lu, S. M. Chen, Z. Gao, F. Z. Shen, W. S. Zhang, Y. X. Xu, H. S. Kwok and Y. G. Ma, J. Mater. Chem., 2011, 21, 5451 RSC.
-
(a) J. Thaksen, D. Bhausaheb, M. M. Shaikh and M. Rajneesh, J. Mater. Chem. C, 2015, 3, 9981 RSC;
(b) D. Kumar, K. R. J. Thomas, C. C. Lin and J. H. Jou, Chem.–Asian J., 2013, 8, 2111 CrossRef CAS PubMed.
-
(a) J. Wang, R. Mason, D. VanDerveer, K. Feng and X. R. Bu, J. Org. Chem., 2003, 68, 5415 CrossRef CAS PubMed;
(b) J. Wang, L. Dyers, R. Mason, P. Amoyaw and X. R. Bu, J. Org. Chem., 2005, 70, 2353 CrossRef CAS PubMed.
-
(a) W. Y. Dong, Y. Y. Pan, M. Fritsch and U. Scherf, J. Polym. Sci., Part A: Polym. Chem., 2014, 53, 1753 CrossRef;
(b) R. R. Hu, J. L. Maldonado, M. Rodriguez, C. M. Deng, C. K. W. Jim, J. W. Y. Lam, M. M. F. Yuen, G. R. Ortiz and B. Z. Tang, J. Mater. Chem., 2012, 22, 232 RSC.
-
(a) Y. Liao, S. Bhattacharjee, K. A. Firestone, B. E. Eichinger, R. Paranji, C. A. Anderson, B. H. Robinson, P. J. Reid and L. R. Dalton, J. Am. Chem. Soc., 2006, 128, 6847 CrossRef CAS PubMed;
(b) L. Chen, L. X. Wang, X. B. Jing and F. S. Wang, J. Mater. Chem., 2011, 21, 10265 RSC;
(c) A. Lv, M. Stolte and F. Würthner, Angew. Chem., Int. Ed., 2015, 54, 10512 CrossRef CAS PubMed.
Footnote |
† Electronic supplementary information (ESI) available. CCDC 1435680. For ESI and crystallographic data in CIF or other electronic format see DOI: 10.1039/c5ra25424h |
|
This journal is © The Royal Society of Chemistry 2016 |