DOI:
10.1039/C5RA25220B
(Paper)
RSC Adv., 2016,
6, 20014-20020
Diketopyrrolopyrrole-based ratiometric fluorescent probe for the sensitive and selective detection of cysteine over homocysteine and glutathione in living cells†
Received
27th November 2015
, Accepted 29th January 2016
First published on 1st February 2016
Abstract
A new fluorescent probe (DPP-AC) based on diketopyrrolopyrrole with an acrylate group was designed and synthesized for the sensitive and selective detection of biological thiols. The acrylate group of DPP-AC as the receptor moiety displayed obvious ratiometric changes from red to yellow upon the addition of cysteine (Cys), and the change in fluorescence ratio (I552/I664) was over 20-fold. The limit of detection towards Cys was calculated as 84 nM; much lower than the normal concentration in living cells. More importantly, DPP-AC had outstanding selectivity towards cysteine over homocysteine (Hcy), glutathione (GSH) and other amino acids. DPP-AC was successfully employed to detect endogenous Cys in Hela cells and discriminate Cys from Hcy and GSH in living cells.
Introduction
Biological thiols, such as cysteine (Cys), homocysteine (Hcy) and glutathione (GSH), mainly refer to the small intracellular biomolecules containing the sulfhydryl group and have strong nucleophilicity and redox reactivity.1–3 These biothiols are widespread in biological environments, and they play significant roles in maintaining the redox homeostasis of proteins, cells, and organisms, and maintaining normal human physiological function.4–6 Abnormal thiol levels are closely associated with various neurodegenerative and cardiovascular diseases, such as Alzheimer’s disease, folate deficiency, various tumors, liver and skin damage, neurotoxicity, Parkinson’s disease, and so on.7–11 For these reasons, it is of critical importance to take some scientific measures to detect these biological thiols selectively and sensitively in research and clinical treatment, which has also attracted extensive attention in recent years.12,13
There are many classical ways to detect these small biological thiols, such as electrochemical detection and high performance liquid chromatography. However, the development of small molecule probes for the detection of thiols under physiological conditions has attracted increasing interest because of their high sensitivity, selectivity, rapid response, and low cost.14–17 In particular, the ratiometric fluorescent probes show better performance than traditional turn-on and turn-off fluorescent probes. Because the ratio of fluorescent signals with a shift in emission maxima can be applied to make an internal calibration of the reacted and unreacted probe, they can minimize the interference of systematic errors, such as the light intensity, sample thickness and heterogeneity, dye distribution, and so on.18–23 Therefore, the ratiometric probes are comparatively ideal fluorescent probes in biological detection and imaging.
Recently, plenty of scientific research has been carried out to implement the detection of biological thiols, but how to discriminate between these three sulfhydryl-containing amino acids (Cys, Hcy and GSH) remains a considerable problem.24,25 The sulfhydryl groups on the side chain of these amino acids have strongly nucleophilic reactivity and redox susceptibility, which are widely applied as recognition mechanisms, including the Michael addition,26–29 cleavage of sulfonic ester linkers,11,30–32 cleavage of ether linkers,33 cleavage of the disulfide group,6,34,35 metal complex-displacement coordination,36 and others.12,13 As a consequence, it is fairly difficult to discriminate the thiols by these reaction mechanisms. Some impressive work has succeeded in differentiating GSH from these three thiols,7,8,12,24,37,38 but mechanisms of distinguishing Cys from Hcy are much rarer, because there are great similarities between them in terms of structure and reaction activity, and the only difference is that Hcy has an additional methylene unit.39 However, the reaction of conjugate addition/cyclization sequence of acrylates is one of the effective mechanisms to solve this problem. In this reaction, both the sulfhydryl and amino groups are involved, and the intramolecular cyclization process differs in reaction rate between Cys and Hcy, meanwhile GSH cannot set off such a cyclization reaction due to its overlong side chain. The acrylate group is an ideal recognition moiety towards thiols using the addition/cyclization reaction mechanism.40–42 Besides, the acrylate group is also a strong electron-withdrawing moiety and could induce a sharp bathochromic shift in fluorescence, so its introduction may cause the ratiometric fluorescence change before and after the reaction with biothiols. A number of works have achieved great success in this field.43,44
1,4-Diketo-3,6-diphenylpyrrole[3,4-c]pyrrole (DPP) and its derivatives have been extensively applied in many fields due to their exceptional thermal and photochemical stability, large extinction coefficients, and high fluorescence quantum yields in both solution and membranes.45–47 In particular, DPP is a typical electron-deficient fluorophore and has many modification sites. When functionalized with electron-donor groups, DPP derivatives can exhibit red to near-infrared (NIR) emission and a large Stokes shift, which are of great benefit to their applications in bioimaging.48,49 However, only a few applications of DPP were reported in the field of reaction-based fluorescent probes.31,46,50–53
Recently, our group has reported a red turn-on fluorescent chemodosimeter (DPP-DNBS) based on a DPP derivative for the detection of Cys,31 but its sensitivity and selectivity towards the biothiols were not satisfactory for their discrimination in living cells. Its poor water-solubility also caused much inconvenience to the biological applications. In order to further boost the optical performance, a new fluorescent probe based on DPP derivatives (DPP-AC, Scheme 1) was developed for the detection of thiols in this study. The methoxytriphenylamine group, with its strong electron-donating ability, could extend the fluorescent emission enormously. The acrylate group was introduced as a selective reaction site and an electron-withdrawing group in the hope of realizing higher selective detection and ratiometric fluorescence change. Compared with 2,4-dinitrobenzenesulfonyl groups, the electron-withdrawing ability of the acrylate group was much weaker and might not quench the fluorescence. Morpholine groups were connected to the side chains of the DPP in order to improve the water solubility, which could give the bioprobe better cytocompatibility.54,55 As expected, the probe DPP-AC displayed high selectivity for the detection of Cys over Hcy and GSH, and the ratiometric properties gave it a much lower limit of detection and a much better anti-interference capability. Moreover, the DPP-AC probe has been successfully used to detect endogenous Cys and discriminate Cys, Hcy and GSH in living Hela cells.
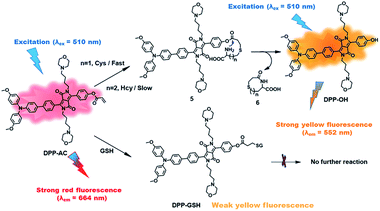 |
| Scheme 1 Chemical structures of probe DPP-AC and its reaction mechanism with Cys, Hcy and GSH. | |
Results and discussion
Molecular design and synthesis
In order to obtain sensitive and selective fluorescent probes with long emission wavelengths for the detection of biological thiols, probe DPP-AC was designed and synthesized (Scheme 2). DPP is an electron-deficient fluorophore, so the introduction of the methoxytriphenylamine group constructed a fluorescent molecule with a typical donor–acceptor structure, which greatly increased the absorption and emission wavelength of the fluorophore and made the fluorescent signal obtain deeper tissue penetration and minimum interference of the auto-fluorescence in the organisms. The acrylate group has a strong electron-withdrawing ability as well as reactivity with biological thiols, so its existence might result in a further red shift in fluorescence, causing the ratiometric fluorescence changes. After undergoing the cyclization and cleavage reaction with the targets, the fluorescence would change with the leaving of the electron-withdrawing groups. Additionally, the introduction of morpholine groups would be beneficial to improving the water solubility of the molecule to some degree.
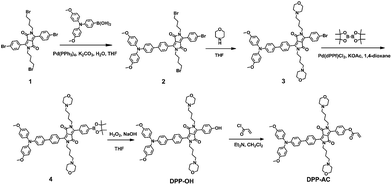 |
| Scheme 2 Synthetic route towards probe DPP-AC. | |
The synthesis route towards probe DPP-AC is shown in Scheme 2. Compound 2 was obtained through a Suzuki coupling reaction between compound 1 and 4-(bis(4-methoxyphenyl)-amino)phenyl boronic acid. After the introduction of morpholine to the alkyl chain of DPP, compound 3 could react with bis(pinacolato)diboron under the catalysis of bis(diphenylphosphino)ferrocene palladium dichloride. Compound DPP-OH was synthesized by the oxidation of compound 4 with hydrogen peroxide. Finally, in the presence of triethylamine, DPP-OH could react with acryloyl chloride to obtain the desired product, DPP-AC. All of the structures of the new compounds were confirmed by 1H NMR, 13C NMR and MS-ESI, and the relevant spectra are shown in the ESI.†
Optical response of probe DPP-AC towards Cys
The absorption and time-dependent fluorescence responses of DPP-AC towards Cys were firstly studied in DMSO-buffer solution (PBS
:
DMSO = 6
:
4, v/v, pH = 7.4) at 37 °C in order to obtain more obvious experimental phenomena and clearer photographs. From the absorption spectra of DPP-AC (10 μM) shown in Fig. 1A, the maximum absorption wavelength showed an obvious blue shift of about 20 nm when reacting with 10.0 equivalents (100.0 μM) of Cys, Hcy and GSH. The addition of biothiols reduced the absorbance significantly; especially the addition of Cys. Hence, the color of the solution paled and turned slightly yellow, which was observed with the naked eye. Besides, we were surprised to find that the changes of fluorescence were also very obvious (Fig. 1C). The solution of DPP-AC showed strong red fluorescent emission at 664 nm before the addition of Cys (Fig. S1†), but with the addition of Cys, the red fluorescence gradually declined, while the yellow fluorescence at 552 nm gradually increased. After 100 min, the solution showed yellow fluorescence and remained unchanged. This phenomenon might result from the effect of the intramolecular charge transfer (ICT) process. The acrylate had electron-withdrawing ability, which made the fluorescence show red shifts by a large margin. However, the ICT process was cut off after the reaction of acrylate with Cys, and the fluorescence turned from red to yellow simultaneously. This kind of ratiometric fluorescent change was of great benefit to the application in living cells, and it was also conducive to obtaining a lower limit of detection.
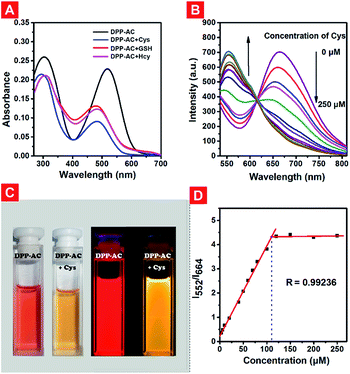 |
| Fig. 1 (A) Absorption of DPP-AC (10 μM) in the absence and presence of Cys, Hcy, and GSH (100 μM) in DMSO-buffer solution. (B) Fluorescence spectra of DPP-AC (10 μM) reacted with various concentrations (0–250 μM) of Cys in DMSO-buffer solution. (C). Photographs of DPP-AC before and after the reaction with Cys, under natural light and UV radiation, respectively. (D) Linear correction between the fluorescence ratio (I552/I664) changes and the Cys concentration addition (0–250 μM) at 37 °C in DMSO-buffer solution (PBS : DMSO = 6 : 4, v/v, pH = 7.4) at 37 °C with an excitation wavelength (λex) of 510 nm. | |
The concentration-dependent optical response towards Cys was also studied. Different concentrations from 0.0 to 250.0 μM of Cys solution were added to DPP-AC (10 μM) solution. After 100 min, the fluorescence intensity was measured. The change in fluorescence upon titration with Cys was recorded in Fig. 1B. The free DPP-AC exhibited fluorescence at 664 nm. With the addition of Cys, the fluorescence decreased gradually, and at the same time the fluorescence at 552 nm appeared, suggesting that a new fluorophore was built. The fluorescence intensity ratio (I552/I664) of DPP-AC showed good linear correlation with the concentrations of Cys (0.0–100.0 μM) (Fig. 1D). Therefore, DPP-AC could also be used for the quantitative detection of Cys within a certain range. The limit of detection was calculated as 84 nM; much lower than the normal concentration (0.25–0.38 mM) in living cells. The fluorescence quantum yields of DPP-AC and DPP-OH were estimated 0.128 and 0.149, respectively, with rhodamine B in ethanol as a standard.
Selectivity towards different amino acids
When designing a probe for biological applications, excellent selectivity is one of the most important properties we need to take into consideration. For this purpose, the three biothiols (Cys, Hcy, GSH) and eleven other kinds of amino acid were selected to conduct contrast experiments (Fig. 2), including alanine (Ala), arginine (Arg), aspartic (Asp), glutamic (Glu), glycine (Gly), leucine (Leu), methionine (Met), proline (Pro), serine (Ser), threonine (Thr), and tryptophan (Try).
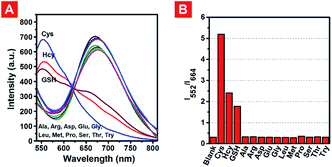 |
| Fig. 2 Fluorescent responses of DPP-AC (A and B) upon addition of various kinds of amino acids. Fluorescent spectra (A) were acquired after the addition of various amino acids (20 μM) in DMSO-buffer solution (PBS : DMSO = 6 : 4, v/v, pH = 7.4) at 37 °C. Bars (B) represent the fluorescence intensity ratio I552/I664 of DPP-AC (λex = 510 nm). | |
As can be seen in Fig. 2, all of the common amino acids without sulfhydryl groups did not cause the ratiometric change of DPP-AC in DMSO-buffer solution (PBS
:
DMSO = 6
:
4, v/v, pH = 7.4). On the contrary, the addition of Cys, Hcy and GSH would induce ratiometric changes in the fluorescence, but the fluorescence changes induced by Hcy were much less and slower than Cys. Besides, there was only a slight change in fluorescence for GSH under the same conditions. This difference might result from the different relative reaction rates of intramolecular cyclization, because the cyclization reaction to form an eight-membered ring was more kinetically unfavorable than that of the seven-membered ring, and the overlong side chain of GSH prevents it from setting off such reactions. Based on the different reaction rates and mechanism, DPP-AC showed outstanding selectivity towards Cys over Hcy, GSH and other amino acids.
Detection mechanism in sensing Cys
In order to further explain the reaction mechanism of DPP-AC with Cys, 1H NMR and MS titrations were performed. Based on the reported conjugate addition/cyclization reaction of the acrylate moiety in detecting Cys over Hcy and GSH, the carbon–carbon double bond in the acrylate moiety of DPP-AC would disappear after the intramolecular cyclization and a small cyclic molecule would form, which could definitely bring about some changes in the low field of the 1H NMR spectrum (Fig. 3). There are three groups of peaks ranging from 6.05 ppm to 6.67 ppm in Fig. 3, corresponding to the three protons of the acrylate moiety. Upon the addition of Cys, they were significantly decreased and finally disappeared after 2 hours on account of the change from a carbon–carbon double bond to a single bond with the addition of the sulfhydryl group. In the meantime, a new singlet peak gradually appeared at 8.02 ppm on account of the generation of a phenolic hydroxyl group, illustrating the generation of the new compound DPP-OH. An MS titration was also performed to confirm the reaction mechanism (Fig. S2†). After reacting with Cys (10 μM) for 30 min, the reaction products were analyzed by mass spectrometry. The peaks at m/z = 890.4487 and m/z = 944.4519 correspond to DPP-OH ([C57H61N5O8 + H]+ calculated m/z 890.4422) and the unreacted DPP-AC ([C54H59N5O7 + H]+ calculated m/z 944.4598), respectively. Besides, the addition intermediate product (compound 5, [C61H70N6O10S+H]+ calculated m/z 1079.4952) and the cyclization product (compound 6, [C6H9NO3S + H]+ calculated m/z 176.0311) were also found at m/z = 1079.4915 and 176.0301, respectively.
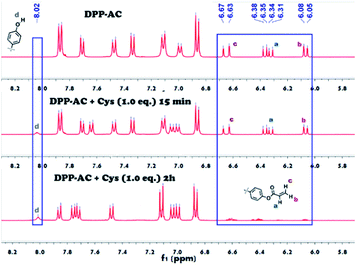 |
| Fig. 3 Selected 1H NMR spectra of DPP-AC (5 mM) reacted with 1.0 equivalent of Cys for 0 s (without Cys), 15 min and 2 h in DMSO-d6. | |
Stability
Stability is another important indicator to evaluate the properties of the fluorescent probe. For this purpose, experiments on photostability, chemostability and pH stability were performed (Fig. 4). As shown in Fig. 4A, after strong light irradiation of 1 kW m−2 (a 300 W xenon lamp as a white exited source which can simulate the solar spectrum) for over 60 min, the fluorescence intensity of fluorescein isothiocyanate declined dramatically, by more than 50%. On the contrary, the intensity of DPP-AC and its reaction product DPP-OH decreased no more than 16% under the same conditions, demonstrating pretty good photostability.
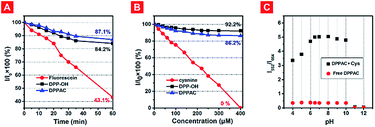 |
| Fig. 4 (A) Photostability of DPP-AC (blue triangles) and DPP-OH (black squares) compared with fluorescein isothiocyanate (red dots) under 1 kW m−2 light irradiation for 0–60 min. I0 is the initial fluorescent intensity and I is the fluorescent intensity of the sample after light irradiation at certain time intervals. (B) Chemostability of DPP-AC (blue triangles) and DPP-OH (black squares) compared with cyanine (red dots) after the addition of 40 equivalents of NaClO. I0 is the initial fluorescent intensity and I is the fluorescent intensity of the sample after the addition of certain equivalents of NaClO. (C) The fluorescent intensity ratio (I552/I664) of 10 μM DPP-AC and before (red dots) and after (black squares) the reaction with 100 μM Cys for 120 min at different pH values. λex = 510 nm. | |
NaClO was selected to test the chemostability of the probes (Fig. 4B). After titration with 40 equivalents of NaClO, the fluorescence of DPP-AC and DPP-OH both remained almost unchanged, showing good chemostability. However, as a comparison, the pentamethine cyanine dye had already become non-fluorescent because of its poor chemostability towards oxidizing agents.
The effect of pH was also studied. The information in Fig. 4C demonstrates that DPP-AC could react sensitively with Cys in the range of pH 6–10, and the fluorescence changes were extremely obvious in this pH range. In acidic conditions (pH < 6), the reaction rate and the extent of fluorescence changes became slightly lower on account of the lower reactivity at low pH values. When the pH value was too high (pH > 10), the molecular structure of DPP-AC was completely damaged and the fluorescence disappeared. Consequently, this probe showed fairly good stability and fluorescence response towards Cys in the physiological pH range and could be employed for detection in living cells.
Intracellular detection
Probe DPP-AC was applied to detect cellular biothiols in living Hela cells (Fig. 5). The bioimaging was observed with confocal laser scanning microscopy (CLSM). The Hela cells were firstly incubated with 10 μM DPP-AC for 20 min at 37 °C. There was strong fluorescence observed in the green channel (532.8 nm–572.8 nm) and weak fluorescence observed in the red channel (642.8 nm–682.8 nm) under the fluorescence microscope. We speculated that DPP-AC had already reacted with endogenous Cys in the living cells, because the limit of detection of the probe was much lower than the concentration of endogenous Cys. Thus a control experiment was then performed to confirm this explanation. The Hela cells were firstly pretreated with 5 mM N-ethylmalemide (NEM) for 60 min to remove the endogenous thiol species in the cells, followed by incubation with 10 μM DPP-AC for 20 min at 37 °C. Just as expected, little fluorescence could be observed in the green channel, while the fluorescence in the red channel became a lot stronger than Fig. 5B. Meanwhile, another control experiment was continued. The pre-incubating cells with NEM were firstly stained with DPP-AC (10 μM), then Cys (50 μM) was added and they were incubated for 20 min. We found that the fluorescence in the green channel become strong again, and the fluorescence in the red channel became much weaker simultaneously. This result showed that the fluorescence changes were indeed caused by the endogenous Cys in the living cells, and the probe DPP-AC had the ability to detect endogenous Cys in living cells due to its much lower LOD than the concentration of Cys in Hela cells. And it was more visual to analyze the changes from the intensity ratio signals of the green/red channels. Moreover, the favorable overlay of the fluorescent image and bright field image illustrated that the probe was distributed in the cytoplasm homogeneously.
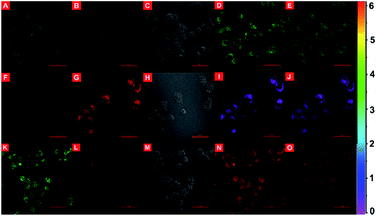 |
| Fig. 5 Confocal laser scan images of Hela cells. Cell images were obtained using an excitation wavelength of 510 nm. (A–E) Images of Hela cells incubated with DPP-AC (10 μM) at for 20 min at 37 °C. (F–J) Images of Hela pretreated by NEM (5 μM) for 60 min, and then incubated with DPP-AC (10 μM) for 20 min at 37 °C. (K–O) Images of Hela cells stained with DPP-AC (10 μM) for 20 min at 37 °C, and then further treated with Cys (50 μM) for 20 min at 37 °C. (A, F and K) Green channel (λex = 510.0 nm, λem = 532.8 nm–572.8 nm). (B, G and L) Red channel (642.8 nm–682.8 nm). (C, H and M) Bright field image (D, I and N) pseudocolored ratiometric images (Fgreen/Fred) generated from green channel to red channel (E, J and O) overlay of the ratio images and bright field images. Note: the ratiometric images were obtained by the image analysis software, Image-Pro. Scale bar = 50 μm. | |
Similar experiments were also conducted for Hcy and GSH. The pre-incubating cells with NEM were firstly stained with DPP-AC (10 μM), then Cys, Hcy, and GSH (50 μM) was added respectively and they were then incubated for 20 min. As shown in Fig. 6, compared with the other two biothiols, the change of fluorescence intensity ratio was much more obvious when the cells treated with Cys, and the ratiometric fluorescence signals successfully helped us discriminate Cys from Hcy and GSH in living Hela cells.
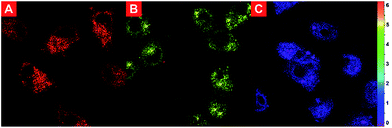 |
| Fig. 6 Pseudocolored ratiometric images (Fgreen/Fred) of Hela cells incubated with DPP-AC (10 μM) for 20 min, and then treated with 50 μM three different biothiols (Cys, Hcy and GSH) for 20 min at 37 °C. Cell images were obtained using an excitation wavelength of 510 nm. (A) Cys (B) Hcy (C) GSH. Note: the ratiometric images were obtained by the image analysis software, Image-Pro. Scale bar = 50 μm. | |
Conclusions
In this study, a new fluorescent probe (DPP-AC) based on diketopyrrolopyrrole with an acrylate group was developed with a convenient synthetic route for the detection of biothiols. Depending on the strong electron-withdrawing ability and specific cyclization and cleavage reaction of the acrylate moiety with Cys, DPP-AC displayed an impressive ratiometric fluorescence change towards Cys, which was beneficial to rectify the systematic errors and improve the signal–noise ratio. The change of the fluorescence ratio (I552/I664) was over 20-fold, and the limit of detection was calculated as 84 nM; much lower than the concentration in living cells. Both 1H NMR and MS titrations were performed to explain the cyclization and cleavage reaction mechanism of detecting Cys. Besides, DPP-AC showed perfect sensitivity and selectivity towards Cys over Hcy, GSH and various other amino acids, which solves a perplexing problem in biothiol probe design. More importantly, the ratiometric probe was successfully employed to detect endogenous Cys in living Hela cells, and the fluorescence ratio signals were conducive to the quantitative analysis of Cys. This performance means that the probe possesses the potential for monitoring and analyzing the concentration of biothiols in cells to facilitate clinical diagnosis. This research paves a significant way for designing DPP-based ratiometric fluorescent probes with effective sensing capability and high biocompatibility for biological applications.
Experimental section
Synthesis of compound 2
Compound 1 (400 mg, 0.56 mmol), Pd(PPh3)4 (24 mg, 0.04 mmol) and 4-(bis(4-methoxyphenyl)amino)phenyl boronic acid (196 mg, 0.56 mmol) were dissolved in THF (15 mL). Under the protection of an argon atmosphere, 5 mL of aqueous potassium carbonate solution (2 M) was added. The reaction mixture was then stirred at 40 °C for 3 hours before being allowed to cool to room temperature. The reaction mixture was extracted with dichloromethane (3 × 15 mL) and then the organic layer was separated and washed with water and brine. After drying over Na2SO4 and removal of the solvent under reduced pressure, the residue was purified over silica gel using dichloromethane/petroleum ether (3
:
1, v/v) as the eluent to obtain the pure compound 2 (210 mg, 0.22 mmol, 39% yield). 1H NMR (400 MHz, CDCl3) δ (ppm): 7.88 (d, J = 8.0 Hz, 2H), 7.87–4.69 (m, 6H), 7.48 (d, J = 8.0 Hz, 2H), 7.11 (dd, J = 8.0 Hz, 4H), 6.99 (d, J = 8.0 Hz, 2H), 6.87 (d, J = 8.0 Hz, 2H), 3.87–3.78 (m, 10H), 3.37–3.34 (m, 4H), 1.87–1.77 (m, 8H). 13C NMR (100 MHz, CDCl3) δ (ppm): 162.94, 160.31, 156.20, 150.81, 149.02, 146.28, 143.59, 140.48, 130.93, 129.12, 127.59, 126.98, 126.71, 125.71, 120.09, 116.55, 114.81, 109.76, 108.37, 55.52, 53.44, 32.82, 29.81, 27.92. HRMS (ESI, m/z): [M + H]+ calcd for C46H43N3Br3O4+ 938.0804, found 938.0814.
Synthesis of compound 3
Compound 2 (200 mg, 0.21 mmol) and morpholine (75 mg, 0.85 mmol) were dissolved in 15 mL of THF. The reaction mixture was then stirred under an argon atmosphere overnight at room temperature. After removing the solvent under reduce pressure, the residue was purified over silica gel using ethyl acetate/dichloromethane (1/20, v/v) as the eluent to obtain the pure compound 3 (146 mg, 0.15 mmol, 72% yield). 1H NMR (400 MHz, CDCl3) δ (ppm): 0.7.84 (d, J = 8.0 Hz, 2H), 7.74 (d, J = 8.0 Hz, 2H), 7.69 (d, J = 8.0 Hz, 2H), 7.45 (d, J = 8.0 Hz, 2H), 7.11 (dd, J = 8.0 Hz, 4H), 6.97 (d, J = 8.0 Hz, 2H), 6.86 (dd, J = 8.0 Hz, 4H), 3.89–3.81 (m, 10H), 3.71–3.66 (m, 8H), 2.40–2.31 (m, 12H), 1.68–1.67 (m, 4H), 1.50–1.49 (m, 4H). 13C NMR (100 MHz, CDCl3) δ (ppm): 162.27, 161.43, 153.30, 140.18, 139.48, 133.58, 133.17, 132.87, 131.21, 130.69, 128.41, 127.87, 127.61, 125.09, 121.20, 115.89, 91.92, 68.48, 58.73, 57.48, 54.48, 45.86, 28.51, 27.28. HRMS (ESI, m/z): [M + H]+ calcd for C54H59BrN5O6+ 952.3649, found 952.3626.
Synthesis of compound 4
A mixture of compound 3 (200 mg, 0.21 mmol), bis(pinacolato) diboron (216 mg, 0.85 mmol), potassium acetate (84 mg, 0.86 mmol) and 1,1′-bis(diphenylphosphino)ferrocene palladium dichloride (91 mg, 0.11 mmol) was dissolved in 15 mL of 1,4-dioxane which was used as the solvent. The reaction mixture was stirred and refluxed for 4 hours under the protection of an argon atmosphere. After cooling to room temperature, the reaction solution was extracted with dichloromethane (3 × 15 mL) and then the combined organic layers were dried with anhydrous Na2SO4. After removing the organic solvent under reduced pressure, the residue was purified over silica gel using ethyl acetate/dichloromethane (1/15, v/v) as the eluent. After drying in vacuo, compound 4 was obtained as a red solid (90 mg, 0.08 mmol, 41% yield). 1H NMR (400 MHz, CDCl3) δ (ppm): 7.97 (d, J = 8.0 Hz, 2H), 7.88 (d, J = 8.0 Hz, 2H), 7.77 (d, J = 8.0 Hz, 2H), 7.72 (d, J = 8.0 Hz, 2H), 7.47 (d, J = 8.0 Hz, 2H), 7.11 (dd, J = 8.0 Hz, 4H), 6.99 (d, J = 8.0 Hz, 2H), 6.86 (d, J = 8.0 Hz, 2H), 3.86–3.79 (m, 10H), 3.36–3.33 (m, 8H), 3.32–3.30 (m, 4H), 2.51–2.47 (m, 8H), 1.83–1.74 (m, 8H), 1.37–1.26 (m, 12H). 13C NMR (100 MHz, CDCl3) δ (ppm): 162.86, 162.03, 153.90, 147.61, 144.53, 140.78, 140.07, 135.91, 135.46, 134.18, 131.29, 131.25, 130.70, 129.01, 128.47, 128.21, 125.68, 121.79, 116.48, 100.33, 82.14, 59.33, 58.08, 55.07, 46.45, 29.10, 27.88, 26.62. HRMS (ESI, m/z): [M + H]+ calcd for C60H71BrN5O8+ 1000.5396, found 1000.5390.
Synthesis of DPP-OH
Compound 4 (100 mg, 0.10 mmol) was dissolved in 15 mL of THF and stirred under an argon atmosphere. Keeping the reactor in an ice bath, 2 drops of hydrogen peroxide solution (30%) and 0.1 mL of sodium hydroxide solution (1 M) were slowly added to the reaction mixture. The color of the solution darkened. After stirred for 20 min, dilute hydrochloric acid was added dropwise to the solution until the pH value was adjusted to 6.0. After removing the solvent under reduced pressure, the residue was purified over silica gel using ethanol/dichloromethane (1/20, v/v) as the eluent to obtain the pure compound DPP-OH as a deep red solid (80 mg, 0.09 mmol, 90% yield). 1H NMR (400 MHz, CDCl3) δ (ppm): 8.12 (s, 1H), 7.84 (d, J = 8.0 Hz, 2H), 7.5 (d, J = 8.0 Hz, 2H), 7.69 (dd, J = 8.0 Hz, 4H),7.46 (d, J = 8.0 Hz, 2H), 7.12 (dd, J = 8.0 Hz, 4H), 6.99 (dd, J = 8.0 Hz, 4H), 6.87 (d, J = 8.0 Hz, 2H), 3.88–3.70 (m, 10H), 3.69–3.68 (m, 8H), 2.48–2.38 (m, 12H), 1.68–1.67 (m, 4H), 1.53–1.48 (m, 4H). 13C NMR (100 MHz, CDCl3) δ (ppm): 160.86, 160.03, 159.29, 142.53, 138.78, 138.07, 133.46, 132.18, 129.54, 129.29, 127.01, 126.47, 126.21, 125.47, 123.68, 119.79, 114.96, 114.48, 89.60, 67.08, 57.33, 56.08, 53.07, 44.45, 27.10, 25.88. HRMS (ESI, m/z): [M + H]+ calcd for C54H60N5O7+ 890.4493, found 890.4445.
Synthesis of DPP-AC
A mixture of DPP-OH (100 mg, 0.11 mmol) and acrylyl chloride (31 mg, 0.33 mmol) was dissolved in 10 mL of anhydrous dichloromethane. Under an argon atmosphere, 0.1 mL of triethylamine was added to the solution at 0 °C. The reaction mixture was then warmed to room temperature and stirred overnight. The organic solvent was then removed in vacuo and the residue was purified over silica gel using petroleum ether/dichloromethane as the eluent (1/20, v/v) to obtain a red solid (51 mg, 0.05 mmol, 51% yield). 1H NMR (400 MHz, CDCl3) δ (ppm): 7.87 (dd, J = 8.0 Hz, 4H), 7.71 (d, J = 8.0 Hz, 2H), 7.47 (d, J = 8.0 Hz, 2H), 7.34 (d, J = 8.0 Hz, 2H), 7.11 (dd, J = 8.0 Hz, 2H), 6.99 (d, J = 8.0 Hz, 1H), 6.88 (m, 1H), 6.85 (d, J = 8.0 Hz, 1H), 3.86–3.81 (m, 10H), 3.68–3.63 (m, 8H), 3.66–3.63 (m, 4H), 2.53–2.49 (m, 8H), 1.82–1.78 (m, 8H). 13C NMR (100 MHz, CDCl3) δ (ppm): 126.84, 162.41, 156.25, 155.86, 154.84, 149.15, 140.41, 131.27, 130.74, 129.26, 129.00, 127.61, 127.03, 126.69, 122.75, 122.24, 120.56, 119.99, 114.82, 110.25, 109.35, 55.52, 53.46, 32.95, 32.78, 29.78, 29.68, 27.99. HRMS (ESI, m/z): [M + H]+ calcd for C57H62N5O8+ 944.4598, found 944.4524.
Acknowledgements
For financial support of this research, we thank the National Basic Research 973 Program (2013CB733700), and NSFC/China (21421004, 21172073, 21372082, 21572062 and 91233207).
Notes and references
- K. Wang, H. J. Peng and B. H. Wang, J. Cell. Biochem., 2014, 115, 1007–1022 CrossRef CAS PubMed.
- M. Zhang, M. X. Yu, F. Y. Li, M. W. Zhu, Y. H. Gao, L. Li, Z. Q. Liu, J. P. Zhang, D. Q. Zhang, T. Yi and C. H. Huang, J. Am. Chem. Soc., 2007, 129, 10332–10333 CrossRef PubMed.
- S. P. Wang, W. J. Deng, D. Sun, M. Yan, H. Zheng and J. G. Xu, Org. Biomol. Chem., 2009, 7, 4017–4020 CAS.
- H. S. Jung, X. Q. Chen, J. S. Kim and J. Y. Yoon, Chem. Soc. Rev., 2013, 42, 6019–6031 RSC.
- G. L. Wang, H. J. Jiao, X. Y. Zhu, Y. M. Dong and Z. J. Li, Analyst, 2013, 138, 2000–2006 RSC.
- M. H. Lee, J. H. Han, J. H. Lee, H. G. Choi, C. H. Kang and J. S. Kim, J. Am. Chem. Soc., 2012, 134, 17314–17319 CrossRef CAS PubMed.
- L. Y. Liu, Y. S. Guan, Y. Z. Chen, L. Z. Wu, C. H. Tung and Q. Z. Yang, J. Am. Chem. Soc., 2012, 134, 18928–18931 CrossRef PubMed.
- J. Yin, Y. Kwon, D. Kim, D. Lee, G. Kim, Y. Hu, J. H. Ryu and J. Y. Yoon, J. Am. Chem. Soc., 2014, 136, 5351–5358 CrossRef CAS PubMed.
- W. B. Zhang, P. L. Li, Q. Q. Geng, Y. H. Duan, M. C. Guo and Y. S. Cao, J. Agric. Food Chem., 2014, 62, 5845–5852 CrossRef CAS PubMed.
- F. Y. Wang, Z. Q. Guo, X. Li and C. C. Zhao, Chem.–Eur. J., 2014, 20, 11471–11478 CrossRef CAS PubMed.
- M. Li, X. M. Wu, Y. W. Y. S. L. W. H. Zhu and T. D. James, Chem. Commun., 2014, 50, 1751–1753 RSC.
- M. Isik, R. Guliyev, S. Kolemen, Y. Altay, B. Senturk, T. Tekinay and E. U. Akkaya, Org. Lett., 2014, 16, 3260–3263 CrossRef CAS PubMed.
- R. X. Peng, L. L. Lin, X. X. Wu, X. H. Liu and X. M. Feng, J. Org. Chem., 2013, 78, 11602–11605 CrossRef CAS PubMed.
- N. Jiang, J. L. Fan, F. Xu, X. J. Peng, H. Y. Mu, J. Y. Wang and X. J. Peng, Angew. Chem., Int. Ed., 2015, 54, 2510–2514 CrossRef CAS PubMed.
- L. Yang, W. S. Qu, X. Zhang, Y. D. Hang and J. L. Hua, Analyst, 2015, 140, 182–189 RSC.
- J. L. Fan, H. Y. Mu, H. Zhu, J. Y. Wang and X. J. Peng, Analyst, 2015, 140, 4594–4598 RSC.
- H. T. Zhang, C. Y. Zhang, R. C. Liu, L. Yi and H. Y. Sun, Chem. Commun., 2015, 51, 2029–2032 RSC.
- M. H. Lee, J. S. Kim and J. L. Sessler, Chem. Soc. Rev., 2015, 44, 4185–4191 RSC.
- F. Y. Wang, L. Zhou, C. C. Zhu, R. Wang, W. Fei, S. H. Luo, Z. Q. Guo, H. Tian and W. H. Zhu, Chem. Sci., 2015, 6, 2584–2589 RSC.
- K. Li, Q. Q. Su, W. Yuan, B. Tian, B. Shen, Y. H. Li, W. Feng and Y. F. Li, ACS Appl. Mater. Interfaces, 2015, 22, 12278–12286 Search PubMed.
- S. L. Shen, X. F. Zhang, S. Y. Bai, J. Y. Miao and B. X. Zhao, RSC Adv., 2015, 5, 13342–13346 Search PubMed.
- T. D. Ashton, K. A. Jolliffe and F. M. Pfeffer, Chem. Soc. Rev., 2015, 44, 4547–4595 RSC.
- C. Hu, W. Sun, J. F. Cao, P. Gao, J. Y. Wang, J. L. Fan, F. L. Song, S. G. Sun and X. J. Peng, Org. Lett., 2013, 15, 4022–4025 CrossRef CAS PubMed.
- S. Y. Lim, K. H. Hong, D. Kim, H. Kwon and H. J. Kim, J. Am. Chem. Soc., 2014, 136, 7018–7025 CrossRef CAS PubMed.
- L. Y. Liu, Y. Z. Chen, H. R. Zheng, L. Z. Wu, C. H. Tung and Q. Z. Yang, Chem. Soc. Rev., 2015, 44, 6143–6160 RSC.
- H. Kwon, K. Lee and H. J. Kim, Chem. Commun., 2011, 47, 1773–1775 RSC.
- V. Hong, A. A. Kislukhin and M. G. Finn, J. Am. Chem. Soc., 2009, 131, 9986–9994 CrossRef CAS PubMed.
- W. Y. Lin, L. Yuan, Z. M. Cao, Y. M. Feng and L. L. Long, Chem.–Eur. J., 2009, 15, 5096–5103 CrossRef CAS PubMed.
- Y. Liu, Y. Yu, J. W. Y. Lam, Y. N. Hong, M. Faisal, W. Z. Yuan and B. Z. Tang, Chem.–Eur. J., 2010, 16, 8433–8438 CrossRef CAS PubMed.
- Q. Zhang, S. S. Ding, Q. zhai and G. Q. Feng, RSC Adv., 2015, 5, 62325–62330 RSC.
- W. S. Qu, L. Yang, Y. D. Hang, X. Zhang, Y. Qu and J. L. Hua, Sens. Actuators, B, 2015, 211, 275–282 CrossRef CAS.
- L. Peng, Z. J. Zhou, R. R. Wei, K. Li, P. S. Song and A. J. Tong, Dyes Pigm., 2014, 108, 24–31 CrossRef CAS.
- M. D. Hammers and M. D. Pluth, Anal. Chem., 2014, 86, 7135–7140 CrossRef CAS PubMed.
- X. M. Wu, W. R. Sun, Z. Q. Guo, J. B. Tang, Y. Q. Shen, T. D. James, H. Tian and W. H. Zhu, J. Am. Chem. Soc., 2014, 136, 3579–3588 CrossRef CAS PubMed.
- K. G. Reddie, W. H. Humphries, C. P. Bain, C. K. Payne, M. L. Kemp and N. Murthy, Org. Lett., 2012, 14, 680–683 CrossRef CAS PubMed.
- N. Shao, J. Y. Jin, S. M. Cheung, R. H. Yang, W. H. Chan and T. Mo, Angew. Chem., Int. Ed., 2006, 45, 4944–4948 CrossRef CAS PubMed.
- J. Liu, Y. Q. Sun, Y. Y. Huo, H. X. Zhang, L. F. Wang, P. Zhang, D. Song, Y. Shi, Y. W. Shi and W. Guo, J. Am. Chem. Soc., 2014, 136, 574–577 CrossRef CAS PubMed.
- L. Y. Niu, Y. S. Guan, Y. Z. Chen, L. Z. Wu, C. H. Tung and Q. Z. Yang, Chem. Commun., 2013, 49, 1294–1296 RSC.
- L. Y. Niu, Y. S. Guan, Y. Z. Chen, L. Z. Wu, C. H. Tung and Q. Z. Yang, J. Am. Chem. Soc., 2012, 134, 18928 CrossRef CAS PubMed.
- X. F. Yang, Y. X. Guo and R. M. Strongin, Angew. Chem., Int. Ed., 2011, 50, 10690–10693 CrossRef CAS PubMed.
- D. H. Yu, Q. Zhang and S. S. Ding, RSC Adv., 2014, 4, 46561–46567 RSC.
- M. M. Zheng, H. X. Huang, M. Zhou, Y. Q. Wang, Y. Zhang, D. J. Ye and H. Y. Chen, Chem.–Eur. J., 2015, 21, 10506–10512 CrossRef CAS PubMed.
- Z. Q. Guo, S. W. Nam, S. S. Park and J. Y. Yoon, Chem. Sci., 2012, 3, 2760–2765 RSC.
- X. Dai, T. Zhang, Y. Z. Liu, T. Yan, Y. Li, J. Y. Miao and B. X. Zhao, Sens. Actuators, B, 2015, 207, 872–877 CrossRef CAS.
- M. Kaur and D. H. Choi, Chem. Soc. Rev., 2013, 44, 58–77 RSC.
- X. F. Yang, L. J. Xie, R. Ning, X. Q. Gong, Z. Liu, L. Y. Zheng, G. G. Zhang, B. Gao, G. X. Sun and G. Y. Zhang, Sens. Actuators, B, 2015, 210, 784 CrossRef CAS.
- Y. T. Gao, G. X. Feng, T. Jiang, C. C. Goh, L. G. Ng, B. Liu, B. Li, L. Yang, J. L. Hua and H. Tian, Adv. Funct. Mater., 2015, 25, 2857–2866 CrossRef CAS.
- Y. H. Li, Y. Sun, J. C. Li, Q. Q. Su, W. Yuan, Y. Dai, C. M. Han, Q. H. Wang, W. Feng and F. Y. Li, J. Am. Chem. Soc., 2015, 137, 6407–6416 CrossRef CAS PubMed.
- G. L. Zhang, H. Y. Zhang, Y. Gao, R. Tao, L. J. Xin, J. Y. Li, F. Y. Li, W. L. Liu and J. Qiao, Organometallics, 2014, 33, 61–68 CrossRef CAS.
- L. Y. Wang, J. Q. Du and D. R. Cao, Sens. Actuators, B, 2015, 205, 281–288 CrossRef.
- Y. D. Hang, X. P. He, L. Yang and J. L. Hua, Biosens. Bioelectron., 2015, 65, 420–426 CrossRef CAS PubMed.
- L. Y. Wang, L. L Yang, L. B. Huang and D. R. Cao, Sens. Actuators, B, 2015, 209, 536–544 CrossRef CAS.
- L. Y. Wang, L. H. Zhu and D. R. Cao, New J. Chem., 2015, 39, 7211–7218 RSC.
- C. L. Zhu, L. B. Liu, Q. Yang, F. T. Lv and S. Wang, Chem. Rev., 2012, 112, 4687–4735 CrossRef CAS PubMed.
- X. T. Zhang, Z. Y. Gu, L. B. liu, S. Wang and G. W. Xing, Chem. Commun., 2015, 51, 8606–8609 RSC.
Footnote |
† Electronic supplementary information (ESI) available: Detailed experimental procedures, supporting figures. See DOI: 10.1039/c5ra25220b |
|
This journal is © The Royal Society of Chemistry 2016 |