DOI:
10.1039/C5RA24983J
(Review Article)
RSC Adv., 2016,
6, 14964-14992
A perspective on bioethanol production from biomass as alternative fuel for spark ignition engine
Received
25th November 2015
, Accepted 21st January 2016
First published on 25th January 2016
Abstract
The increasing consumption of fossil fuels has led to the development of alternative fuels for the future. Domestic biofuel production and the utilization of alternative fuels can decrease dependency on petroleum oil, reduce trade deficits, reduce air pollution and reduce carbon dioxide emission. Bioethanol is a renewable fuel produced by the fermentation of sugar which is derived from plants such as sugarcane or beet, maize, or cassava etc. However, bioethanol consumption in an engine is approximately 51% higher than gasoline since the energy per unit volume of ethanol is 34% lower than for gasoline. Bioethanol is an oxygenated fuel that contains 35% oxygen, which can reduce particulate matter and NOx emissions caused by combustion of the fuel. Therefore, bioethanol–gasoline blends can significantly reduce petroleum use and GHG emission. In addition, utilization of lignocellulosic materials in bioethanol production is the most viable pathway from an environmental point of view. This paper reviews the current status and technologies involved in bioethanol production and the properties and engine performance from various biomass feedstocks which are the recommended sustainable alternative fuel in the future.
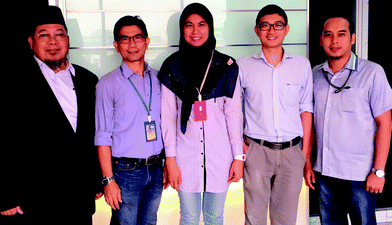 A. H. Sebayang, H. H. Masjuki, Hwai Chyuan Ong, S. Dharma, A. S. Silitonga, T. M. I. Mahlia and H. B. Aditiya | The research on biofuels has been established and developed by group members from Centre for Energy Sciences, Faculty of Engineering, University of Malaya, Kuala Lumpur, Malaysia. Professor Dr. Masjuki Haji Hassan is the leader of Biofuel research in University of Malaya. He is secretary of Council of National Professors Engineering and Technology cluster. He is also the founding President of Malaysian Tribology Society (MyTRIBOS) and the Director of the Centre for Energy Sciences. Dr. Ong Hwai Chyuan, Mr Abdi Hanra Sebayang and Mr Surya Dharma are the academic staff and Researcher in Faculty of Engineering, University of Malaya and are also involved in biofuel research. The group collaborated with Professor Dr. T. M. I. Mahlia from Universiti Tenaga Nasional (UNITEN), Dr. Arridina Susan Silitonga from Medan State Polytechnic, North Sumatera, Indonesia and Mr Aditiya Harjon Bahar from University of Melbourne, Australia. |
1. Introduction
The energy sector plays a vital role in the world economy. Increasing fossil fuel prices, energy security issues and climate change have driven and forced energy development towards alternative and renewable energy sources. Due to the soaring interest in developing renewable and cleaner energy sources, biofuel production caught our attention in the early 2000s.1 Biofuels are seen as part of the drive to move beyond the dominant fossil-fuel paradigm and the production has been increasing remarkably. This is due to many countries’ attempts to reduce oil imports, boost rural economies and improve air quality. Brazil and USA are the largest world’s ethanol producers and possess 70% of the world’s ethanol production.2 The countries were able to export 17 and 20 billion liters from Brazil and USA respectively in 2006.3 Europe stands out with its sugarcane bioethanol program and outstanding results have been gained along the entire production chain from the development, increase in sugarcane variety, and engine manufacturing that can run any gasoline and bioethanol blend.4 North America and Sweden introduced vehicles that operate on a fuel blend of 85% ethanol with 15% gasoline.5 The world’s major bioethanol producers are shown in Fig. 1.3 The USA leads the world’s bioethanol production by almost 20 billion liters of bioethanol. Second is Brazil which produced almost 17 billion liters of bioethanol, with cheaper production cost of $0.18–0.20 per liter compared with USA ($0.33–0.47 per liter). The EU draws high production cost for every liter of bioethanol produced, which ranges between $0.50–0.97 per liter, although in comparison to China, EU produced less bioethanol by 3.4 billion liters, while China produced 3.85 billion liters. Expanding bioethanol production will increase the use of renewable energy resources, thus enabling independence from fossil fuels. Bioethanol has been receiving widespread interest at the international, national and regional levels. The research on ethanol production has been established with the potential of ethanol as a valuable replacement of gasoline in the transportation fuel market.
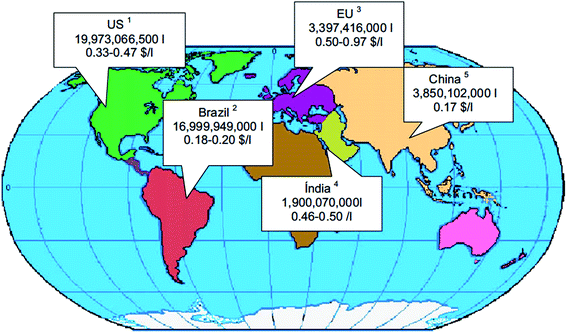 |
| Fig. 1 The main producers and production costs of bioethanol.3 | |
Ethanol can be obtained from the microbial conversion of lignocellulosic biomass through fermentation.6 The quality of the bioethanol depends on the feedstock. Therefore, many researchers have reviewed the designs and implementation technologies, from the simple conversion of various biomass, such as sugarcane, corn, cassava, rice straw and other agricultural wastes by fermentation, to the multi-stage conversion system of lignocellulosic biomass into bioethanol.7,8 The fermentation method generally comprises of three steps: (1) the formation of a fermentable solution from the selected biomass, (2) the fermentation of this solution and (3) the separation and purification of the ethanol, usually by distillation.9 Favorability of bioethanol can be seen from its properties, including density, vaporization heat, flame temperature, fuel octane, and hydrogen and carbon content which affects the compression ratio.10 Using 10% of bioethanol in a gasoline blend has the benefits of: reducing CO emissions by 8–30%, reducing toxic emissions by 5–15%, slowing the depletion of the ozone layer and lowering pollution.11 Currently, many countries have introduced 5–10% anhydrous ethanol blended with 95–90% gasoline (E10), and this mixture contains 3.5% oxygen compared to only 2.7% in pure gasoline.10,12 The higher oxygen content in the blend can contribute to a better combustion and an increase thermal efficiency.5,13 Furthermore, blending of bioethanol with gasoline affects its fuel properties, such as viscosity, lubricity, octane number, energy content, volatility and stability.14 However, a major drawback of bioethanol–gasoline fuel is that high blending of ethanol (40–60%) causes wear and corrosion of fuel pumps.5 The aim of this paper is to give a thorough overview of the current status of production, properties and engine performance of bioethanol as the substitute for gasoline fuel.
2. Feedstock for bioethanol production
The feedstock availability for bioethanol depends on the region, climate conditions as well as the soil properties.15 According to Balat et al.,16 the feedstock for bioethanol production can be categorized into three biomass types: (i) lignocellulosic biomass (e.g. straw, grasses and wood), (ii) starchy materials (e.g. corn, barley, corn, etc.) and (iii) sucrose-containing feedstocks (e.g. sugarcane, sugar beet, sweet sorghum, etc.). Algae is the feedstock with the incredibly high ethanol yield, which change the game on potential feedstock availability.4,15,17 The potential for bioethanol production from various biomass feedstocks and their comparative production potential are given in Table 1.18,19 A diverse classification of biofuel feedstocks and processes are currently being developed to meet sustainability and fuel quality standards, as well as the need of road, aviation and marine users. Biofuel can be marketed as a renewable and sustainable alternative fuel in the future. The classification of the generation for biofuel feedstocks is described in Fig. 2.20 It is clearly seen that the main difference of the classifications is due to their feedstock type. First generation utilizes carbohydrate-source crops, which is in general obtainable in the forms of seeds, grains and sugars. Second generation uses lignocellulosic biomass, which is sourced from agricultural residues. In third generation, algae (micro and macro type) are utilized as the bioethanol production feedstock and show a higher reproducibility rate in a lower area requirement.
Table 1 Potential bioethanol production for various biomass feedstocks18,19
Bioethanol feedstock |
Bioethanol production potential (L per ton) |
Sweet sorghum |
60 |
Sugarcane |
70 |
Sugar beet |
110 |
Potato |
110 |
Sweet potato |
125 |
Cassava |
180 |
Barley |
250 |
Bagasse and other cellulose biomass |
280 |
Wheat |
340 |
Maize |
360 |
Rice |
430 |
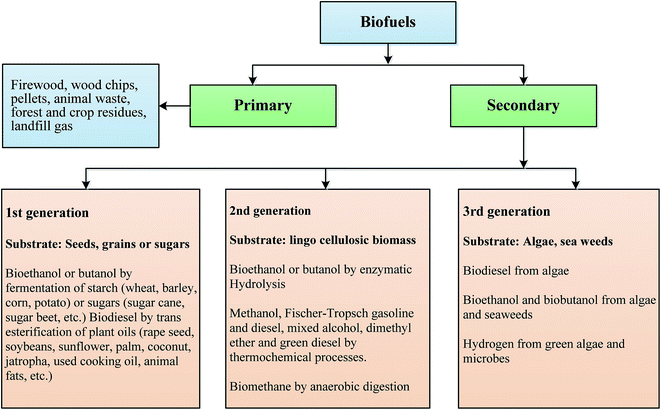 |
| Fig. 2 The classification of the generation of biofuel feedstocks.20 | |
2.1 First generation biomass
First generation bioethanol is produced from sugar-rich biomass (consisting of linkages of monosaccharides of glucose and fructose) and starch-rich (polysaccharides of glucose) crops such as grain and corn. The contained sugars can be converted to ethanol only after hydrolysis to yield fermentable sugars. The technology is well-known but the high price is the undesirable side effect of this raw material. On the other hand, first generation have raised debate about using food products for fuel production.21 The details of producing bioethanol from various first generation sources are discussed below.
2.1.1 Sugarcane and sugar beet. Sugarcane (Saccharum officinarum) is from the genus Saccharum belonging to the poaceae family; it contains a large amount of organic nutrients and minerals to make free sugars as an ideal raw material for bioethanol production.22 It is the most important feedstock grown in tropical and subtropical countries that can be used as juice or molasses (byproduct of sugar mills) for bioethanol production.16 The sugar produced from sugarcane is readily converted into glucose (the simplest sugar monomer) and can be used as fermentation substrate under anaerobic conditions. Besides, glucose is converted to ethanol and carbon dioxide by glycolysis.23 The typical content of fermentable sugar is 12–17%, in which 90% is sucrose and 10% is glucose and fructose.22,24 Therefore, sugarcane contains a high sugar content and it is the main feedstock used for ethanol production in Brazil and India.15 In addition, the sugarcane crop is favorable since its growing season is only 3–5 months.25Sugar beet (Beta vulgaris), also called beet molasses, is a suitable feedstock for bioethanol production due to the high sugar content that can be extracted by fermentation without any modifications. Sugar beet contains 15–20% of dry matter, 85–90% of fermentable sugars and 10–15% free sugars.4,22 The high free sugar content in sugar beet is usable in fermentation with an adjustment of the pH, and this feedstock gives a more profitable profile for bioethanol production.26,27 The composition profile of sugarcane and sugar beet are listed in Table 2.22,28
Table 2 The composition profile of sugarcane and sugar beet22,28
Components |
Concentration |
Sugarcane |
Sugar beet |
Solids (%) |
13.7 |
17.3 |
Sugar (%) |
12–17 |
16.5 |
Raffinose (%) |
— |
0.07 |
Monosaccharides (%) |
0.63 |
0.15 |
Polysaccharides (%) |
0.028 |
0.019 |
Lactate (%) |
0.016 |
— |
Acetate (%) |
0.033 |
— |
Sulphate (%) |
0.039 |
0.02 |
Phosphate (%) |
0.033 |
0.047 |
Nitrate (%) |
— |
0.015 |
Nitrite (%) |
— |
0.005 |
Aconitate (%) |
0.09 |
— |
K (%) |
0 |
— |
Na (%) |
0.005 |
0.015 |
Cl (%) |
— |
0.003 |
Ca (%) |
0.04 |
— |
Mg (%) |
0.028 |
— |
Total-N (%) |
— |
0.105 |
Betaine-N (%) |
— |
0.046 |
Amino acid-N (%) |
— |
0.026 |
Ammonia-N (%) |
— |
0.006 |
Amide-N (%) |
— |
0.011 |
2.1.2 Sweet sorghum. Sweet sorghum (Sorghum bicolor (L.) Moench) has been used as a potential bioethanol feedstock due to its high biomass yield and high concentration of readily fermentable sugars.25,29 Sweet sorghum has a wide degree of adaptability for the tropical region, is well adapted to sub-tropical and temperate regions of the world, and is water efficient.,25,30 Moreover, sorghum is efficient at photosynthesis based utilization of soil nutrients and its low water consumption means that it is tolerant to drought and flood, compared to sugarcane.31,32 Sweet sorghum has fast lifecycle ranging from a 3–5 month growing season.25,33 Sweet sorghum tolerates compacted subsoil at pH range of 5.0–8.5 with some degree of salinity and alkalinity and poor drainage, and its planting temperature is above 12 °C.30,34 The productivity of sweet sorghum delivers 2000–8000 liters per ha of bioethanol, making sweet sorghum a suitable feedstock for bioethanol production.8,26 The composition profile of sweet sorghum is shown in Table 3.35
Table 3 The composition profile of sweet sorghum35
Composition |
Sweet sorghum |
One kg of the stalk was crushed at 2500 psi for 1 min in a press to extract the juice. |
Juicea (% total) |
71.9 |
Sucrose (% juice) |
7.6 |
Glucose (% juice) |
2.6 |
Fructose (% juice) |
1.6 |
Total sugars (% juice) |
11.8 |
Fiber (% wt) |
13.0 |
Cellulose (% wt) |
44.6 |
Hemicellulose (% wt) |
27.1 |
Lignin (% wt) |
20.7 |
Ash (%) |
0.4 |
Brix (% juice) |
11–13 |
2.1.3 Wheat, corn, and barley grain. Wheat (Triticum vulgare) is a very good raw material for bioethanol production due to the complete gelatinization of wheat starch at 65 °C.18 Utilization of wheat or unusable wheat leads to cost effectiveness and hence it is a good option for bioethanol production.36 Moreover, 3.5 tons of wheat grain, or 3.03 tons dried wheat, can be harvested from one acre of good arable land.37Corn (Maize) is species of Zea and is an annual monoecious flowering hybrid of Zea mays.38 Corn cultivars are widely adapted and can be grown from 0–55° latitude, from sea level to 12
000 ft and with a growing season of 42–400 days.39 29% of the world’s bioethanol production comes from corn grain.40
Barley (Hordeum vulgare L.) is a very attractive feedstock for bioethanol production and is being used in Europe (62%), Asia (15%) and North America (14%).41 Barley grows well in many areas and it can become a financially cost effective bioethanol feedstock for many regions. Barley is typically utilized for brewing, animal feed, human food and other industrial uses.42 Barley is harvested sooner, and it still can give good maturity and high yield.43,44 Furthermore, 70% of the barley composition is carbohydrate, which indicates good potential in bioethanol production.43 The composition profile of wheat, corn and barley grain are presented in Table 4.45–48
Table 4 The composition profile of wheat, corn and barley grain biomass45–48
Composition |
Wheat |
Corn |
Barley |
Moisture (%) |
12.5 |
15 |
11.1 |
Calories (cal per 100 g) |
330 |
361 |
349 |
Protein (%) |
12.3 |
10.2 |
2.4 |
Fat (%) |
1.8 |
4.3 |
1.0 |
Ash (%) |
1.7 |
1.2 |
0.9 |
Sugar (%) |
3.6 |
5.3 |
5.3 |
2.1.4 Cassava. Cassava (Manihot esculenta Crantz), also know as: manioc, yucca, tapioca, has a diameter and length of 5–10 cm and 15–35 cm respectively.49 It is considered a tuberous root plant that is native to South America and cultivated around tropical and subtropical countries.50,51 Cassava can grow at ambient temperatures of 25–30 °C with an optimum annual rainfall requirement of 760–1015 mm.25 Cassava can tolerate prolonged drought since it has the ability to utilize nutrients from the stalk and the storage roots once moisture is restored.52 Cassava is harvested around 8–24 months after planting.49 Cassava is one of the most important carbohydrate sources in the tropics since it holds a starch content of up to 90% dry weight.50 The rich starch in cassava is related to the unique properties which make cassava suitable for food and non-food applications.53 Therefore, cassava starch is one of the best fermentable substances for the production of ethanol. Cassava also contains protein 1% (fresh) and 1.41% (dry), 65% of moisture, 0.9% of ash and 0.03% of phosphorus.54 Furthermore, cassava has a high carbohydrate content of 32–35%, which is considered productive in agro-economic applications. The composition profile of cassava biomass is presented in Table 5.49,55
Table 5 The composition profile of cassava biomass (100 g basis)49,55
Composition |
Cassava |
Calories (cal) |
135 |
Peel (%) |
10–20 |
Cork layer (%) |
0.5–2.0 |
Edible portion (%) |
80–90 |
Moisture (%) |
62–66 |
Total solids (%) |
38 |
Volatile solids (%) |
99 |
Protein (g) |
1.2 |
Total nitrogen (%) |
0.22 |
Lipid (g) |
0.20 |
Starch (g) |
18–32 |
Fiber (g) |
1.10 |
Carbohydrate (%) |
35 |
Total carbon (%) |
19 |
Ash (%) |
0.9–1 |
Fat (mg) |
0.1 |
Calcium (mg) |
26 |
Phosphorus (mg) |
32 |
Iron (mg) |
1 |
Sodium (mg) |
2 |
Potassium (mg) |
394 |
Vitamin B2 (mg) |
0.04 |
Vitamin C (mg) |
34 |
Niacin (mg) |
0.60 |
Cyanide (%) |
— |
2.2 Second generation biomass (lignocellulosic biomass)
Second generation biomass are derived from lignocellulosic components of agricultural residues, such as straws, wood and other agricultural wastes.56–59 This type of biomass is usable in second-generation biofuel (biomass to liquid) that has an improvement of CO2 balance with the use of cheap, waste sources that do not compete with human food products.15,56,60 The use of agricultural residues can provide benefit to the agricultural sector by providing additional potential income streams for farmers.61
The composition of lignocellulosic biomass is divided into three main components: lignin (10–20% dry wt), cellulose (30–50% dry wt) and hemicellulose (15–35% dry wt).17,62 Hemicellulose (C5H8O4)n, is a polymer structure of hexose (D-glucose, D-mannose and D-galactose) and pentose sugars (D-xylose and L-arabinose).63 It contains D-glucuronic, D-galacturonic and methylgalacturonic acids in sugar acids (uronic acids).64 Hemicelluloses are available as xyloglucans or xylans depending on the types of plants.65 Moreover, hemicelluloses are linked to cellulose as abundant carbonics in plants.66 The dominant source of hemicellulose biomass are woody biomass, hardwoods and softwoods.17,67,68 Cellulose (C6H10O5)n is a long-chain structural component of glucose monomers (D-glucose) linked to β-(1,4)-glycosidic bonds.69 The cellulose molecules also consist of long chains of β-glucose monomers gathered into micro-fibril bundles.70 Cellulose is insoluble in water, and a hydrolysis process is required to break down the polymer to free complex sugar molecules, also known as a saccharification process.71 The product of cellulose is a six-carbon sugar from woody and agricultural biomass.67 Lignin C9H10O3(OCH3)0.9–1.7]n is a structural compound of three types of monomers (p-coumaryl, synapyl alcohols and coniferyl) joined together by a set of linkages to create a matrix.72 The complex structures such as hydroxyl, methoxyl and carbonyl have a high polarity which responsible for the hardness of the bonds and consistency of the binding material which holds the cellulosic fibers.73 Lignin is resistant to chemical and biological degradation, which affects the quality of ethanol in bioethanol production.66 The major lignin sources are softwood barks, hardwood barks and herbaceous species such as bagasse, corncobs, peanut shells, rice hulls and straws.7,41,74 The molecule structure of hemicellulose, cellulose and lignin are shown in Fig. 3.75,76 The composition profile of the lignocellulosic biomass is presented in Table 6.41,69,77–81 Furthermore, extensive chemical ash compositions are summarized in Table 7.82–85
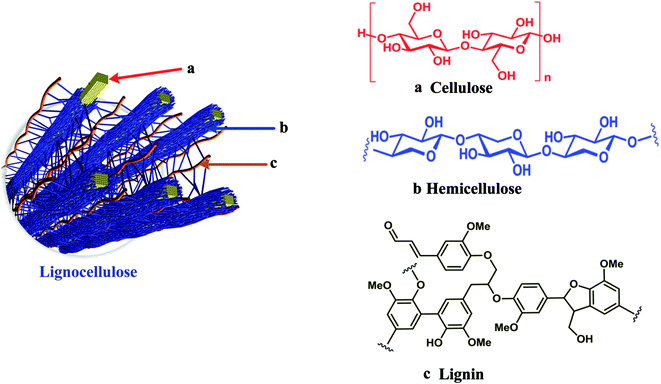 |
| Fig. 3 Lignocellulosic biomass (a) cellulose, (b) hemicellulose and (c) lignin.75,76 | |
Table 6 The composition profile of hemicellulose, cellulose and lignin from lignocellulosic biomass
Lignocellulosic biomass |
Cellulose glucan (% wt) |
Hemicellulose (% wt) |
Lignin (% wt) |
Ref. |
Xylan |
Arabinan |
Galactan |
Mannan |
Acid insoluble lignin |
Acid soluble lignin |
Barley straw |
35.65 |
16.86 |
2.11 |
— |
13.8 |
20.7 |
2.4 |
41 |
Corn stover |
38.3 |
21.0 |
2.7 |
2.1 |
— |
17.4 |
— |
78 |
Wheat straw |
30.2 |
18.7 |
2.8 |
0.8 |
— |
17 |
— |
79 |
Rice straw |
31.1 |
18.7 |
3.6 |
— |
— |
13.3 |
— |
69 |
Rye straw |
30.9 |
21.5 |
— |
— |
— |
22.1 |
3.2 |
69 |
Oat straw |
39.4 |
27.1 |
— |
— |
— |
17.5 |
— |
77 |
Sunflower stalks |
42.1 |
29.7 |
— |
— |
— |
13.4 |
— |
77 |
Sugarcane bagasse |
43.1 |
31.1 |
— |
— |
— |
11.4 |
— |
69 |
Sweet sorghum bagasse |
27.3 |
13.1 |
1.4 |
— |
— |
14.3 |
— |
69 |
Olive tree pruning |
25.0 |
11.1 |
2.4 |
1.5 |
0.8 |
16.2 |
2.2 |
81 |
Poplar |
43.8 |
14.8 |
— |
— |
— |
29.1 |
— |
80 |
Spruce |
43.8 |
6.3 |
— |
— |
14.5 |
28.3 |
0.53 |
69 |
Oak |
45.2 |
20.3 |
— |
— |
4.2 |
21.0 |
3.3 |
69 |
Table 7 The chemical composition of different biomass
Feedstock |
Chemical composition (% wt) |
Ref. |
SiO2 |
CaO |
K2O |
P2O5 |
Al2O3 |
MgO |
Fe2O3 |
SO3 |
Na2O |
TiO2 |
Wood and woody biomass |
Eucalyptus bark |
10.04 |
57.74 |
9.29 |
2.35 |
3.1 |
10.91 |
1.12 |
3.47 |
1.86 |
0.12 |
82 |
Poplar bark |
1.86 |
77.31 |
8.93 |
2.48 |
0.62 |
2.36 |
2.36 |
0.74 |
4.84 |
0.12 |
82 |
Wood residue |
53.15 |
11.66 |
4.85 |
1.37 |
12.64 |
3.06 |
3.06 |
1.99 |
4.47 |
0.57 |
84 |
![[thin space (1/6-em)]](https://www.rsc.org/images/entities/char_2009.gif) |
Agricultural residue |
Bamboo whole |
9.92 |
4.46 |
53.38 |
20.33 |
0.67 |
6.57 |
0.67 |
3.68 |
0.31 |
0.01 |
85 |
Rice husks |
94.48 |
0.97 |
2.29 |
0.54 |
0.21 |
0.19 |
0.22 |
0.92 |
0.16 |
0.02 |
84 |
Sugarcane bagasse |
46.79 |
4.91 |
6.95 |
3.87 |
14.6 |
4.56 |
11.12 |
3.57 |
1.61 |
2.02 |
84 |
Sunflower husks |
23.66 |
15.31 |
28.53 |
7.13 |
8.75 |
7.33 |
4.27 |
4.07 |
0.8 |
0.15 |
83 |
![[thin space (1/6-em)]](https://www.rsc.org/images/entities/char_2009.gif) |
Other biomass residue |
Mixed waste paper |
28.62 |
7.63 |
0.16 |
0.2 |
53.53 |
2.4 |
0.82 |
1.73 |
0.54 |
4.37 |
84 |
Sewage sludge |
33.28 |
13.04 |
1.6 |
15.88 |
12.91 |
2.49 |
15.7 |
2.05 |
2.25 |
0.8 |
83 |
Wood yard waste |
60.1 |
23.92 |
2.98 |
1.98 |
3.08 |
2.17 |
1.98 |
2.46 |
1.01 |
0.32 |
84 |
2.3 Third generation biomass
Currently, microalgae and seaweeds are gaining popularity as third generation biofuel (TGB) feedstocks as the wider expansion of bio-refineries leads to a shortage of energy crops that are intended for biofuel industries.17 The potential of TGB feedstock depends on the structure of various polysaccharides walled by cellulose-based cell walls, which can be further processed and fermented into biofuel.6,17 TGB does not require as much land as the conventional agriculture, has a high productivity area, recycles carbon dioxide, is able to grow from different type of water source (e.g., seawater, brackish water and wastewater), and is compatible with the bio-refineries’ integrated bioethanol production system.86 Besides that, TGB does not raise doubts about impact on food supply and land reservation security.87
2.3.1 Microalgae. Microalgae are an abundant and carbon neutral renewable resource which can be considered as a TGB that can be used in bioethanol production.88 There are various types of microalgae such as C. vulgaris, Chloroccum sp., N. gaditana, etc. that are potential feedstocks for biomass conversion into bioethanol.87,89 Microalgae have a fast grow rate with large yields of biomass and are able to sustain hostile environments including insufficient nutrients and high salinity.90,91 The cell walls of microalgae are cellulose, mannans, xylans, and sulfated glycans.92 Table 8 summarizes the composition profile of algae biomass sources.86,91,93,94
Table 8 Composition profile of microalgae
Microalgae |
Composition (% wt) |
Ref. |
Carbohydrates |
Xylose |
Mannose |
Glucose |
Galactose |
Lipids |
Proteins |
Starch |
Others |
G. verrucosa |
42.67 |
— |
— |
24 |
— |
— |
— |
— |
8.83 |
86 |
C. vulgaris |
44 |
— |
— |
20.52 |
— |
35 |
32 |
12–17 |
— |
94 |
C. infusionum |
32.52 |
9.54 |
4.87 |
15.22 |
2.89 |
— |
— |
11.32 |
56.16 |
93 |
T. suecica |
27.41 |
— |
— |
— |
— |
14.25 |
58.32 |
— |
— |
94 |
Chloroccum sp. |
32.52 |
9.54 |
4.87 |
15.22 |
2.89 |
— |
— |
11.32 |
56.16 |
93 |
C. sorokiniana |
18.2 |
13.8 |
— |
70.8 |
— |
— |
42.8 |
— |
0.78 |
91 |
N. gaditana |
11.2 |
28.8 |
— |
59.0 |
— |
— |
39.5 |
— |
0.17 |
91 |
S. almeriensis |
14.5 |
33.4 |
— |
52.2 |
— |
— |
36.7 |
— |
0.71 |
91 |
2.3.2 Seaweeds. Seaweeds are considered third generation biomass which are a carbon neutral renewable resource compared to first and second generation biomass.95 Seaweeds yield a high rate of biomass and show a fast growth with good productivity in comparison to the land crops.96 The high yield of seaweeds is supported by the lesser energy requirements coupled with their ability to absorb nutrients throughout their surface areas. This makes it possible for seaweeds to save energy since there is no requirement to transport nutrients internally.97 The constituents of seaweeds depend on species, habitat, maturity and environmental conditions, but generally they contain fatty acids, oxylipins, non-starch polysaccharides, dietary fiber, minerals and vitamins, terpenoids, carotenoids, phlorotannins, steroids and protein.98,99 Seaweeds can be harvested naturally or by additional technological efforts, which vary the production cost and yield.100 Seaweeds are able to use the unlimited supply of water and nutrients from the ocean and as a result yield about ±90% of the ±1.6 million tons of total dry seaweed. The growing process of seaweeds also dissolves carbon dioxide on the surface of water, and the required nutrients can also be absorbed from processing effluents.101 The composition profile of seaweeds is presented in Table 9.96,98,99,101–104
Table 9 Composition profile of seaweeds
Seaweeds |
Composition (% wt) |
Ref. |
Carbohydrates |
Fiber |
Lipids |
Protein |
Moisture |
Ash |
Laminaria spp. |
— |
— |
2 |
12 |
— |
26 |
101 |
C. lentillifera |
59.27 |
3.17 |
0.86 |
12.49 |
25.31 |
24.21 |
99 |
S. horneri |
19.93 |
— |
0.82 |
22.94 |
86.94 |
32 |
102 |
C. veravelensis |
37.23 |
— |
2.8 |
7.77 |
87.88 |
33.7 |
96 |
Sargassum spp. |
41.81 |
9.84 |
0.75 |
10.25 |
11.16 |
26.19 |
103 |
T. triquetra |
45.68 |
— |
4.83 |
10.12 |
15.83 |
40.34 |
96 |
S. naozhouense |
47.73 |
4.83 |
1.06 |
11.2 |
— |
35.18 |
98 |
H. clathratus |
82.26 |
2.7 |
2.18 |
6.39 |
59.63 |
6.47 |
104 |
U. reticulata |
55.77 |
4.84 |
0.75 |
21.06 |
22.51 |
17.58 |
99 |
3. Conversion process of biomass to bioethanol
3.1 Biomass feedstock handling
The handling of biomass feedstock is the preliminary step to prepare the feedstock, involving cleaning and preliminary cutting to reduce the physical size before the pretreatment process.105 During feedstock handling, the storage of biomass feedstock is essential to maintain the quality of the supply for bioethanol production. Large storage places are used for biomass feedstock to avoid affecting the biomass through excessive humidity, rodents and microbial growth. Moreover, drying under the sun and thermal or mechanical drying techniques are the common methods before storage.106 Fig. 4 shows the conversion process of biomass to bioethanol.107
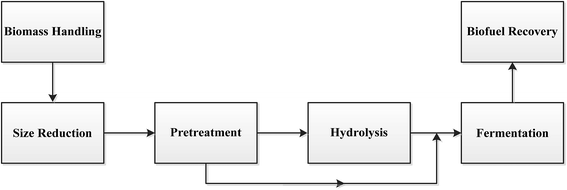 |
| Fig. 4 Conversion process of biomass to bioethanol.107 | |
3.2 Pretreatment of biomass feedstock
In bioethanol production, biomass has complex structures that are required to be broken down into oligomeric subunits by pretreatment.68 The purposes of pretreatment are: to remove hemicellulose, to remove or redistribute lignin (for lignocellulosic biomass); to increase the mean pore size and to provide better access for enzymes (during enzymatic hydrolysis).108 The pretreatment methods can be largely classified into four methods as below.
3.2.1 Physical pretreatment. Physical pretreatment reduces the particle size of the feedstock to increase surface/volume ratio, which eases the subsequent processes in the production.109,110 Physical pretreatment is divided into three techniques as follow:
• Mechanical process. Mechanical pretreatment reduces the feedstock’s size by breaking the physical structure. The typical process starts by washing then size reduction by grinding followed by extracting the essence and solid fraction separation.111,112 In lignocellulosic material, size reduction is important to increase lignocellulose exposure in the hydrolysis process.68 However, it has been reported that for particle size below ±40, mesh is not effective for the conversion to bioethanol.112 In addition, the biomass is generally ground to about 3–8 mm particles in a more compact form with a higher density such as pellets or briquettes.113 Therefore, particle size is an important property to be optimized since it affects the power required for the equipment (e.g. knife mill, hammer mill) as well as the economic feasibility of the selected method.114 Zheng et al.115 stated that mechanical process such as attrition milling, ball milling, compression milling and steam treatment can be used to destruct lignin and give better access for enzymes to attack cellulose and hemicellulose in enzymatic hydrolysis.
• Extrusion process. Extrusion process is a combination of physical and chemical processes to prepare the biomass in bioethanol production.68 This process practices heating, mixing, shearing and screw speed to shatter the lignocellulose structure and finally increase the accessibility of carbohydrates to enzymatic attack.116 The extrusion process can be improved by removing hemicelluloses and lignin by micro/nano fibrillation. This process produces five times higher yield compared to hot compressed water treatment, resulting in 77% delignification, and conversion of 69% and 38% of cellulose and hemicelluloses respectively into glucose, xylose and arabinose.115,117 Zheng et al.115 stated that a twin-screw extruder can remove 80% of xylose with soluble lignin. The process was conducted to corncobs with 100 rpm screw speed, 100 °C barrel temperature, a mass flow rate of 4 kg h−1, and produced 88% of glucose. Furthermore, the extrusion method was studied by employing sequential extrusion and clean fraction pretreatment, which resulted in a 90% glucose yield due to solvent involvement and is recognized as a promising method for lignocellulosic biomass pretreatment.118 Similarly, Karunanithy and Muthukumarappan119 reported that mixing, shearing and heating disturb the feedstock’s composition physically and chemically during the extrusion process while it passed through to the extruder barrel. The effect extrusion process for various feedstocks is presented in Table 10.119–124
Table 10 The effect of extrusion process for various feedstocks
Extrusion process |
Lignocellulose |
Extruder |
Extrusion conditions |
Sugar yield |
Ref. |
Physical pretreatment |
Corn stover |
Single screw extruder |
Screw speed: 75 rpm, temperature: 125 °C |
Glucose: 75%, xylose: 49%, combined sugar: 61% |
119 |
Big bluestern |
Single screw extruder |
Screw speed: 100 rpm, temperature: 150 °C, moisture content: 20% wb, particle size: 8 mm |
Glucose: 55.2%, xylose: 92.8%, combined sugar: 65.4% |
122 |
Acid pretreatment |
Rice straw |
Twin screw extruder |
Screw speed: 40 rpm, temperature: 120 °C H2SO4 concentration: 3% wt |
Xylose: 83.7% |
124 |
Pine sawdust |
Twin screw extruder |
Screw speed: 110 rpm, temperature: 60 °C, head pressure: 780 psi, H2SO4 concentration 70% wt |
Glucose: 44.4% |
123 |
Alkali pretreatment |
Corn stover |
Twin screw extruder |
Screw speed: 80 rpm, temperature: 140 °C, NaOH ratio: 0.04 g g−1 biomass |
Glucose: 86.6%, xylose: 50.5% |
121 |
Switchgrass |
Single screw extruder |
Screw speed: 155 rpm, temperature: 176 °C, particle size: 8 mm, NaOH concentration: 0.02 g g−1 biomass |
Glucose: 40.5%, xylose: 60%, combined sugar: 47.9% |
120 |
• Ultrasonic process. In ultrasonic pretreatment, ultrasonic wave breaks feedstock cells by mechanical shearing and formation of shock waves.125 In lignocellulosic sludge type biomass, the ultrasonic process brings benefits including an improvement in biodegradability, efficient sludge disruption, lower processing time, and no additional chemical substances required.126 Additionally, the ultrasonication process improves the feedstock digestibility by affecting the chemical, biological and physical properties of the feedstock.127 The destruction of cells sets hydrolytic enzymes free and helps to increase the hydrolysis rate of biomass, and the irradiation of cellulose by ultrasonication increases the enzymatic hydrolysis rate to around 200%.128,129 However, this process is well-known as an expensive method and requires a high energy input. In a study, Kidak et al.130 reported the material disruption by ultrasonication gives more desirable results with higher ultrasonic power. They also found that the method requires less retention time since a longer ultrasonication period gives no additional improvement.
3.2.2 Chemical pretreatment. Chemical pretreatment is identified as structure disruption of the biomass through chemical reactions. Like mechanical pretreatment, chemical pretreatment removes lignin, reduces the crystallinity of cellulose and enhances the biodegradability of the biomass.131 Chemical pretreatment is widely used with other techniques. The common methods of chemical pretreatment are as follows:
• Acid process. Acid pretreatment utilizes acidic substances (typically H2SO4 or HCl) for lignocellulosic biomass due to its powerful ability to rupture the components into simpler form.110,114 The low temperature for this process makes it a low cost pretreatment and it can loosen the cell wall matrix through hemicellulose degradation.68 The process does not affect lignin, but cellulose microfibrils are sufficient to produce high yields of monomeric sugars for fermentation.26,132 However, the utilization of acid pretreatment increases the corrosion of the equipment, hence it is important to overcome this issue especially in a large scale production with high acid reagents.110,112 Therefore, many acid processes have been modified to improve all downstream processes as well as the bioethanol yield and prices.26 Tang et al.134 performed acid pretreatment on Eulaliopsis binata using dilute sulfuric acid, resulting in 21.02% total sugars with low inhibitor levels after pretreatment by 0.5% dilute sulfuric acid at 160 °C for 30 min and at a solid-to-liquor ratio of 1
:
5. Marzialetti et al.135 performed acid hydrolysis on loblolly pine using various acids, including trifluoroacetic acid, HCl, H2SO4, HNO3, and H3PO4. The research found that trifluoroacetic acid yielded the most sugar monomers of 70% from hemicellulose at 150 °C at pH 1.65, and this acid is claimed to be the mildest acid used in the study.
• Alkali process. Alkali process is the pretreatment process of feedstock catalyzed by alkali chemicals at room temperature for up to 24 h.112,132 This method is applied in a simple operation and gives high conversion yields with only short span of time.110 It produces less sugar degradation, but the appearing inhibitors are expected to be eliminated in order to optimize the pretreatment conditions.136 Therefore, this pretreatment method is claimed to be more effective for bioethanol production than acid pretreatment.137 The suitable alkali reagents are NaOH and lime, which are employed to break intercellular bonds crosslinking hemicellulose and other components (lignin and cellulose).133 Moreover, the alkali pretreatment can cause structure swelling, increasing porosity, decreasing polymerization degree and crystallinity, separation of lignin, hemicellulose, and cellulose and also disrupts the lignin structure.114 Many studies on alkali process have focused on pretreatment to improve substrate digestibility. Sun and Cheng133 reported that alkali pretreatment is capable of lignocellulosic delignification and at the same time enhancing the reactivity of carbohydrates. It is also found that by utilizing sodium hydroxide in alkali pretreatment, it improves lignocellulose digestibility in typical lignocellulosic feedstocks, for instance wheat straw, thus it is appropriate for second generation biomass pretreatment.138,139 Chang et al.140 reported oxidative lime pretreatment with a feedstock of poplar wood. The study reported that 78% of lignin was removed with glucose improvement of 71% through enzymatic hydrolysis. Sun et al.141 conducted alkali pretreatment by different alkali solutions to release hemicellulose for wheat straw. It showed that 80% hemicellulose was released as well as 60% of lignin at 20 °C by 1.5% sodium hydroxide for 14 hours of pretreatment. Additionally, hemicellulose can produce high amounts of xylose compared to glucose and galactose at the subsequent hydrolysis process. Park et al.142 conducted bioethanol research by utilizing alkali pretreatment, resulting in 74% ethanol after 19 hours fermentation by a combination of Saccharomyces cerevisiae and Pichia stipitis as the fermentation process. Alkali reagents such as KOH, NaOH, hydrazine, anhydrous ammonia and Ca(OH)2, are typically featured in alkali pretreatment of biomass. Moreover, alkali reagents can cause biomass swelling that exposes the internal surface area as well as a decrease in crystallinity and polymerization degree.138,143
• Organosolv process. The organosolv process utilizes organic solvents to break the internal lignin–hemicellulose bonds at high temperature (100–250 °C) and provides more access to cellulose.68,144 The typical suitable organic solvents for this pretreatment method are namely tetrahydrofurfuryl alcohol, ethylene glycol, methanol, acetone and ethanol, and they are noted for their ability to solubilize lignin as well as treating cellulose so that it is digestible for enzymatic hydrolysis.68,145 The effect of organosolv pretreatment on enzymatic hydrolysis was studied by Mesa et al.146 The pretreatment of sugarcane bagasse to produce glucose under a temperature of 175 °C for 40–60 min yielded 22.1 g glucose/100 g biomass. Moreover, pretreatment by the organosolv technique can also be combined with acid (by adding HCl, H2SO4, oxalic or salicylic acid) if the process is conducted between 185–210 °C to break the hemicellulose bonds.147 However, it is suggested that the mentioned combined technique should separate lignin and hemicellulose components so that xylose yield can be focused at the most desirable yield amount.132 In addition, removal of solvents in the organosolv process is generally required due to production of inhibitors which limits the activity of enzymatic hydrolysis and fermentative microorganisms.133 The advantage of organosolv pretreatment is the ability to produce highly digestible cellulose subtracts for all biomass with high value utilization and to remove lignin which troubles the cellulolytic enzymes for lower enzymes dosage.68,112 However, this technique produces high amount of soluble phenols, furfural, 5-hydroxymethyl-2-furfural (HMF), which are obtained from lignin after pretreatment.68,110 The high cost of solvents is the disadvantage of organosolv pretreatment method, but ethanol and methanol are most commonly used solvents in this method due to their low cost.133
• Ozonolysis process. Ozonolysis pretreatment involves ozone as a strong oxidant with high delignification efficiency.148 This process removes lignin, slightly attacks hemicellulose and hardly effect on the cellulose.110 Ozonolysis is pretreatment method for feedstock which is catalyzed at atmospheric conditions (normal pressure and room temperature) and it does not produce inhibitors that impact the hydrolysis and fermentation in bioethanol production.136 Ozonolysis is environmentally sustainable as ozone is a substance that can be decomposed by high temperatures or a catalytic bed, hence less pollution is ejected into the environment.147 Lignocellulosic materials, for instance rye straw, wheat straw and cotton straw, are seen suitable to be handled with this pretreatment method.132 Nevertheless, further research on ozonolysis for bioethanol production is essential in order to further develop the beneficial points and practicality as it is well known that the method is a costly process due to the high amount of required ozone for this pretreatment.68,149García-Cubero et al.150 performed ozonolysis pretreatment to improve sugar yield from wheat and rye straws. It was found that enzymatic hydrolysis of the ozone pretreated biomass resulted in an improvement of 57 and 88.6% of the hydrolysis yield compared to the non-ozone pretreated hydrolysis yields of 16 and 29% from rye and wheat straw respectively. The study concluded that biomass type and moisture content are the substantial factors in ozonolysis. Ozonolysis has also been used in industrial wastewater treatment, and as the pretreatment method for wider various applications.151 As a result of its high delignification efficiency, ozonolysis is also utilized in pulp bleaching in the paper industry.152,153
• Ionic liquids (ILs) process. Ionic liquid pretreatment (ILs) utilizes the organic cations and inorganic anions in salts to pretreat the biomass at room temperature.110,145 In lignocellulosic biomass, ILs dissolve lignin or cellulose, and decreases cellulose crystallinity for a better hydrolysis process as the subsequent process after pretreatment.145 Besides, the IL process has good traits including non flammability, low vapor pressure, and balanced chemical and thermal stability. It also tends to sustain its liquid form in a relatively wide temperature range.68 This means that this pretreatment method supports the improvement of cellulose accessibility at room temperature with or without catalysts (acid or alkali), and it avoids the formation of inhibitors.110,116 Remsing et al.,154 found that in the IL method carbohydrate in the biomass is more attracted to the anion than the cation. This is due to the preference of chloride ions to interact the with hydroxyl protons of the carbohydrate sugar, and it occurs in a stoichiometric ratio of 1
:
1.Francisco et al.155 reported that pretreatment of lignocellulosic biomass has gained significant attention and it can dissolve carbohydrates and lignin simultaneously. The cation structure and degree of anion charge delocalization are two major factors that can affect the properties of ILs such as refractive index, density, viscosity and solubility respectively. Thus, the IL method holds a strong thermal stability as well as negligible vapor pressure and these properties are subject to change depending on the user’s desire and requirement. IL pretreatment may produce impurities, namely water, halides and other volatile substances formed from the biomass or the solvent, although they can be removed by evaporation process.156 ILs are namely green solvents as they does not contain toxic or explosive substances, and can be recycled to reduce the amount used, making them more environmentally friendly.68,157 However, other studies have reported drawbacks of this method, including the compounds harmfulness and issues in recovering ILs.110,145 Furthermore, this technology is expensive and requires further investigation of the feasibility of ILs on large-scale applications, since it holds potential in bioethanol production industry.68,158
3.2.3 Biological pretreatment. Microorganisms are employed to degrade the structure of biomass in biological pretreatment.41,112 The typical microorganisms employed for this technique are white, brown and soft-rot fungi.112 Several of white-rot fungi, namely Ceriporia lacerata, Phanerochaete chrysosporium, Pleurotus ostreatus, Cyathus stercoreus, etc., have been evaluated and resulted in a high delignification efficiency of various lignocellulosic biomasses.68,159 Brown rot fungi targets the hemicellulose and cellulose components of the biomass, white fungi degrades cellulose and lignin components and soft rot fungi are useful to release cellulose from the degradation of lignocellulose complex.8,160 Biological pretreatment is very well-known for its environmental friendliness, although it tends to work on a slow rate compared to other pretreatment methods.161,162 The advantages this method are low process costs and low energy requirements, which are due to decreased mechanical assistance, no requirements of additional chemical substances or applied pressure, it does not cause corrosion to the equipment, and there is a low production rate of inhibitors.6,68 However, the disadvantages of biological pretreatment is the extremely slow degradation rate to attain a high degree of lignin degradation (as long as few months). This causes inefficiency in the subsequent processes of hydrolysis and fermentation required to complete the entire bioethanol production.159 Therefore, there is significant focus on the development of biological pretreatment due to this method having been described as one of the most economic techniques to pretreat biomass for bioethanol industry.105,136There are many reviews reporting biological pretreatment and its future perspectives.163–166 There are few studies that have reported that Aspergillus terreus, Trichoderma spp., Cyathus stercoreus and Lentinus squarrosulus are microbes that can degrade lignocellulosic material (lignin and holocellulose) at 65–80% and 45–75% respectively, with the pretreatment condition of 25–35 °C for 3–22 days.165,166 In a similar study, Zheng et al.115 reported the effectiveness of white-rot fungi in decomposing lignocellulosic biomass, in addition to enhancing the subsequent enzymatic hydrolysis. The study determined that Irpex lacteus (the employed white-rot fungi type) succeeded in degrading lignin by 43.8%, and the subsequent enzymatic hydrolysis was found to be higher by 7-fold.
3.2.4 Thermochemical pretreatment. The application of direct combustion is utilized in thermochemical pretreatment as one simple biomass pretreatment, and this pretreatment generally relies on the biomass properties, including volatile content, moisture content, fixed carbon content, impurities (S, N, Cl) concentration, and ash content, all of which influence the technical and economic factors of bioethanol production.167,168 The pairing of a low cost feedstock with thermochemical pretreatment for bioethanol production can bring out future securities in terms of energy, economic and infrastructure.169 Fig. 5 shows thermochemical processes and they can be used to convert lignocellulosic biomass to liquid, solid and gaseous fuels .170 In addition, several thermochemical pretreatment methods that are commonly applied in bioethanol production are discussed below.
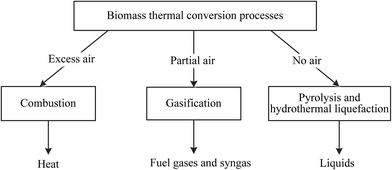 |
| Fig. 5 Lignocellulosic biomass by thermochemical technology.170 | |
• Steam explosion (STEX). Steam explosion (STEX) is widely used in bioethanol production for lignocellulosic biomass thermochemical pretreatment.68,133 This pretreatment used high temperature (160–260 °C) and pressure (0.69–4.83 MPa) and also a sudden depressurization.68 The mechanism used in STEX can be aided by the natural catalyst, acetic acid, which is released by hemicellulose acetate and organic acids such as levulinic and formic acid. The STEX method can used for an auto hydrolysis process or a natural process.171 Some factors can influence STEX efficiency such as moisture content, residence time, temperature, catalyst concentration, and time of presoaking. Optimising these can improve the sugar release, a study reported that the STEX method is able to yield xylose-sugars in a range of 45–65%.71,172 However, STEX can also generate some toxic compounds that could affect hydrolysis and fermentation steps, such as acetic acid, furfural and hydroxymethylfurfural.143 Therefore, inhibitor removal is required for every STEX pretreatment as to the inhibitors can disturb the sustainability of enzymes (in hydrolysis) and yeast (in fermentation), which leads to production additional cost.171
• Liquid hot water (LHW). The working principle of the LHW process is a thermochemical pretreatment of lignocellulosic biomass through subcritical pressure water with the assistance of CO2 to enhance the hydrolysis process.173 The LHW pretreatment is conducted with hot compressed liquid water at temperatures of 160–240 °C and pressure above 5 MPa for 15 min.174 LHW process is able to yield high xylose recovery of 88–98%, with no additional substances as catalyst.175,176 The advantages of LHW process are as a simple pretreatment process with low capital and maintenance costs (reactor materials) due to a lack of corrosion in addition to releasing high sugar yields.136,176 Additionally, lignin and hemicellulose are soluble in LHW pretreatment since it uses high amounts of water.116 Some studies have reported the suitability of LHW pretreatment in bioethanol production from various feedstocks, such as wheat straw, corn stover and sugarcane bagasse. However, the LHW process requires high water and energy consumption which makes this process not viable at the commercial scale.164,175 Nevertheless, LHW pretreatment method draws attention of researchers, especially in lignocellulosic biomass pretreatment. Pérez et al.177 performed LHW pretreatment of wheat straw, yielding sugar recovery of 43.6%, and the subsequent enzymatic hydrolysis yielded 79.8% of sugar recovery. Jiang et al.178 employed LHW pretreatment on branch, stem and boll of cotton stalk. It was found that 4.34 and 4.42% of hemicellulose was removed from the branch and stem respectively, while 58.09% hemicellulose was removed from the boll shell. This study also reported bioethanol yields of 18.3, 16.27 and 21.08 g per 100 g of stem, branch and boll shell respectively. Imman et al.179 added NaOH to the LHW pretreatment of rice straw, and claimed that sugar yield was higher with the NaOH presence (0.25–1.0%). Furthermore, hemicellulose and lignin were efficiently removed from the solid residue and a high pentose yield was obtained in the liquid phase with low formation of unwanted by-products.
• Ammonia fiber explosion (AFEX). AFEX utilizes ammonia to decrease the crystallinity of cellulose in a solid lignocellulosic biomass, as it readily disrupts carbohydrate–lignin bonds.80 AFEX pretreatment can increases lignocellulosic digestibility and enhance the yield from enzymatic hydrolysis as the subsequent process.116 Moreover, AFEX can achieve a conversion of 90% from cellulose hemicellulose into fermentable sugars and this pretreatment can be employed on numerous varieties of lignocellulosic materials.110 In fact, AFEX pretreatment can be also employed in a bioethanol production method of saccharification and cofermentation, using recombinant S. cerevisiae strains as the fermentation agent to obtain high ethanol yields.68 The AFEX method does not introduce inhibitors for subsequent processes in the production line, and cell walls extractives, for instance lignin phenolic fragments, remain on the surface of cellulose.132 The disadvantage of AFEX method is to recover the ammonia due to its high volatility which boosts up the overall production cost.68,143 AFEX pretreatment method is also useful for woody biomass and herbaceous crops.116,147 Numerous studies have been conducted to determine the optimal AFEX pretreatment for different biomass sources. Alizadeh et al.180 pretreated switchgrass with AFEX at 100 °C and an ammonia to biomass ratio of 1
:
1, yielding 0.2 g ethanol per g dry biomass. Bals et al.181 observed that enzyme formulation by AFEX pretreatment produced high sugar yields. Using switchgrass as the biomass, 520 g sugar per kg biomass was released after enzymatic hydrolysis with AFEX pretreatment, while 410 g sugar per kg biomass is normally released.
• Wet oxidation (WO). Wet oxidation pretreatment uses a combination of water, oxygen pressure, elevated temperature and alkali hydrolysis, and it is proven as an efficient pretreatment for lignocellulosic biomass to produce high yield bioethanol.68,137 Oxidation pretreatment operates at relatively low temperatures (170–200 °C), short reactor times (5–15 min) and pressures from 10–12 bar O2.132 In fact, a higher oxygen content at temperatures above 170 °C reduces the energy demand as a result of exothermic process.68 In wet oxidation process, lignin and hemicellulose, as well as cellulose, are solubilized and have their digestibility enhanced, respectively, due to the oxidative reaction and the formation of hydrolytic acid.149 Wet oxidation produces lower inhibitors (furfural and HMF) than LHW or steam explosion methods.182 In fact, the addition of alkali substances (Na2CO3) in this method maintains the process’ pH, reduces toxic compounds, and neutralizes carboxylic acid.110,137 However, this pretreatment method requires high oxygen and catalyst supply, thus driving up the production cost.183 Arvaniti et al.184 reported that the wet oxidation method can pretreat rape straw to produce bioethanol with a yield of 67% through simultaneous saccharification and fermentation (SSF).
• Microwave process (MWP). Microwave process (MWP) combines thermal and non-thermal effects in a watery environment. The microwave gives vibrations to the biomass molecules, which leads to intermolecular collisions that result in heat provision and acceleration of the process.16 In fact, microwaves give a higher heat effect compared to conduction or conventional pretreatment since there is a direct interaction between the object and the electromagnetic wave in a form of supercritical heat transfer.185 MWP is also can be performed paired with alkali catalysts, namely NaOH, Ca(OH)2, Na2CO3, and has been utilized to pretreat lignocellulosic materials including coastal berm, switchgrass, and rice straw and hulls.186,187 The major positive capability of this method is able to start and stop the process instantly, in addition to requiring less energy to run.188 However, the feasibility of the microwave process at the commercial scale is doubtful, and hence further study is strongly suggested to make the method more feasible in the future.189 Chittibabu et al.190 studied the effect of microwave pretreatment on banana pseudostem assisted with an alkali (NaOH), yielding sugar in as much as 84% after enzymatic hydrolysis with cellulase. In a similar study, Nikolić et al.191 employed MWP on cornmeal, and after fermentation by Saccharomyces cerevisiae var. ellipsoideus 13.4% ethanol was yielded.
• CO2 explosion pretreatment. CO2 explosion pretreatment is employed and conditioned as a phase of supercritical fluid, which a compressed gas above the critical point temperature and forming liquid-like substance.68,192 CO2 is injected to the biomass reactor in a very high pressure and is heated at high temperature up to 200 °C and is maintained for a desired period of time.193,194 At this high pressure, the injected CO2 then disrupts the biomass and dissolves in water, where carbonic acid is formed from this reaction and the acid supports the enhancement of the biomass hydrolysis. However, the CO2 explosion method is not compatible with dry biomass due to the lack of moisture content.193,195 CO2 explosion pretreatment also can be paired with other pretreatment methods, for instance, Cha et al.196 combined CO2 explosion with ammonia explosion to pretreat rice straw, resulting in a yield of 93.6% glucose. Gu197 reported CO2 explosion process as a nontoxic green solvent. In this method, the CO2 used to pretreat the biomass can be taken from the CO2 produced from fermentation process and it can emit the CO2 emission to the atmosphere. The advantages of employing CO2 are the cheaper cost compare to ammonia in the explosion, lower production of inhibitor compounds compared to steam explosion, and a higher yield than enzymatic hydrolysis without pretreatment.110
• Sulfite pretreatment to overcome the recalcitrance of lignocellulose (SPORL). Sulfite pretreatment to overcome the recalcitrance of lignocellulose (SPORL) is a pretreatment method that can be used to react with the strong rigidity of lignocellulosic such as woody biomass or softwood. This can overcome the recalcitrance of lignocellulose which negatively affects the sugar and ethanol recovery in bioethanol production.26,110 In this pretreatment, sulfite or bisulfite solution is reacted with the woody biomass at temperature, pH and pretreatment period of 160–190 °C, 2–5, and 10–30 min respectively.26 The SPORL method enhances the sugar yield (57–88%) compared to acid pretreatment and reduces the inhibitor compounds (up to 65%) produced.182 Therefore, SPORL is seen as a promising pretreatment method for woody biomass as it affects the downstream processes, as well as enhancing the efficiency of the bioethanol production yield and its production cost.110,147 Zhang et al.198 reported that SPORL pretreatment improves the biomass digestibility, reducing lignin hydrophobicity by lignin sulfonation, and removes hemicellulose from the biomass. The pretreatment was applied within the range of temperature (163–197 °C), time (3–37 min), sulfuric acid dosage (0.8–4.2% on switchgrass) and sodium sulfite dosage (0.6–7.4% on switchgrass). The results showed that SPORL pretreatment improved the digestibility of switchgrass through sufficiently removing hemicellulose, partially dissolving lignin, and reducing hydrophobicity of lignin by sulfonation.
3.3 Hydrolysis
The hydrolysis process breaks down the complex sugar structures that are built up in the biomass, for instance, polysaccharides in starch and lignocellulose, into the simplest sugar monomers, such as xylose, glucose, etc. In general, hydrolysis is designed to provide suitable fermentable sugars for fermentation microbes in the fermentation process. This effort is necessary since particular microbes are only able to convert particular sugar monomers into ethanol.66,112 Commonly, hydrolysis can be carried out in the following methods:
3.3.1 Dilute acid hydrolysis. Dilute acid is typically utilized to hydrolyze lignocellulosic biomass, since acid is capable of degrading the lignocellulosic structure into fermentable sugars. Dilute acid hydrolysis utilizes around 1% of the acid concentration to degrade the biomass and it yields around 50% of glucose as the end product.199,200 Dilute acid hydrolysis of hemicellulose and cellulose has different approaches in order to identify composition of lignocellulosic. Balat et al.16 reported that hemicellulose can degraded by dilute acid and releases xylose monomers (5-carbon sugar) under a mild hydrolysis environment. Meanwhile, cellulose components require higher temperature for the dilute acid to strike the complex structure of cellulose, and this second approach yields glucose monomers (6-carbon sugar).16 Dilute acid is a practical hydrolysis method for a continuous-production type of bioethanol plant, but prior size reduction of the feedstock is required. Dilute acid is performed in an affordable hydrolysis period and produces a fair end-product. However, it is still a challenge for this method to produce a desirably high sugar yield.167 Besides, inhibitory products are formed as by-products of the process, including acetate, furfural, hydroxybenzaldehyde (HBA), 5-hydroxymethyl furfural, etc., which are harmful for fermenting microorganisms.201
3.3.2 Concentrated acid hydrolysis. Concentrated acid is used to hydrolyze typically lignocellulosic biomass in a fair temperature condition and it employs strong acid (concentration more than 10%). Concentrated acid hydrolysis brings out better sugar yield compared to dilute acid hydrolysis, and it is aimed for a better cost efficiency of hydrolysis process. Despite the high sugar recovery and the ability of the acid to be recovered and re-concentrated, concentrated acid hydrolysis requires extreme precautions, especially during handling the acid substance since concentrated acid is extremely hazardous to human health and the environment.16,202 The drawback of the concentrated acid hydrolysis method is the tendency to corrode the production equipment, which potentially brings a large economic loss to the overall bioethanol production. This method is claimed to have a shorter processing time,203 although others have argued that it is done under a longer hydrolysis period compared to dilute acid hydrolysis.199
3.3.3 Enzymatic hydrolysis. Enzymatic hydrolysis is a common method in bioethanol production which uses a specific type of enzyme based on the type of the biomass component. For instance, cellulase is used to hydrolyze cellulose and amylase to hydrolyze amylose. This hydrolysis method is a complex degradation process, especially for lignocellulosic material, aimed to release the simplest and fermentable sugar monomers. In lignocellulosic biomass, enzymatic hydrolysis works in three ways: (i) the biomass is deformed physically and chemically, (ii) degradation of biomass’s surface, releasing less-complex sugar formations into the solution, (iii) and the last is hydrolysis of the released less-complex sugars in the solution into sugar monomers.204The advantage of enzymatic hydrolysis is that it can take place at only a mild hydrolysis environment (pH of around 4.8 and temperature of around 318–323 K). Enzymatic hydrolysis produces high yields and a better end-product compared to acid hydrolysis which does not promote any corrosion tendency to the processing equipment.205 The costly manufacturing of enzymes indeed drives the enzymatic hydrolysis cost and ultimately the overall bioethanol production cost. However, the growing advance of technologies and rapid development of enzyme manufacturing can reduce the enzyme price. This will affect the bioethanol industry as well for enzymes to become more viable and expandable.206 The production of enzymes, for instance cellulase, can be extracted from fungi and bacteria. The recorded fungi as cellulase and hemicellulose-source microbes include Trichoderma reesei, Trichoderma longibrachiatum, and Trichoderma viride.207 Meanwhile, the typical cellulase-producing bacteria include Acetivibrio, Bacteroides, Bacillus, Cellulomonas, Clostridium, Erwinia, Microbispora, Ruminococcus, Streptomyces and Thermomonospora.205
By employing a commercial cellulase, Tye et al.208 utilized kapok (Ceiba pentandra) fiber as a second generation biomass in an attempt to yield the most fermentable sugar. They observed that cellulase managed to alter the complex structure of the fiber and yielded 85.2% of reducing sugar with prior acid pretreatment. Tan and Lee95 have performed hydrolysis on seaweed solid waste enzymatically to produce bioethanol. The authors observed that enzymatic hydrolysis produced a very high glucose content of 99.8% at very mild hydrolysis conditions of 50 °C and pH 4.8. The study also subsequently performed fermentation and yielded a desirable quality of ethanol of 55.9%. However, simultaneous saccharification and fermentation (SSF) was modified in order to obtain a high ethanol yield of 90% from seaweed waste. It is show that enzymatic hydrolysis is able to be enhanced with the correct additional process and paired with the suitable biomass. Simultaneous saccharification and fermentation (SSF) of seaweed waste can yield a higher ethanol quality of 90.0%. This shows that enzymatic hydrolysis is able to be enhanced with the correct additional process and paired with the suitable biomass.95
3.4 Fermentation
Fermentation is a biomass conversion process into bioethanol by microorganisms (yeast and fungi or bacteria), which occurs by digesting fermentable sugars and producing ethyl alcohol and other byproducts.26,112 Saccharomyces and Pichia are the most common yeast type employed in bioethanol production as well as bacteria Zymomonas and Escherichia and Aspergillus.6 The nature type of fermentation microorganisms are able to convert particular sugar monomers into ethanol, namely hexoses (C6), which are usually yielded in first and second generation production, and pentoses (C5), which are usually yielded in second generation production.209 Most of the studies used Saccharomyces cerevisiae yeast type. Wyman et al.210 reported that Saccharomyces cerevisiae is able to theoretically produce 90% of ethanol from glucose sugar. Similar study by Abbi et al.211 stated that S. cerevisiae is able to ferment hexose sugar, while P. stipitis, P. tannophilus and C. shehatae yeast are typically used to ferment xylose into ethanol efficiently. Kluyveromyces marxianus yeast is able to withstand high temperatures between of 45–52 °C, and is capable of digesting a wide variety of sugar monomers, namely xylose, mannose, arabinose, and galactose.66,212 In order to upgrade the ability of the conversion process, microbe engineering is one alternative which resulted from technological advancement. The benefits include a higher ethanol yield, enabling more substrate to be digested, and more resistance against inhibitors.
In a conventional path, fermentation is occurs last after the biomass is pretreated and completely hydrolyzed. However, as the technology advances, new methods to conduct fermentation are developed as the effort to gain better ethanol quality as well as better production efficiencies. This paper gathers the varieties in fermentation methods, and each of the methods is discussed as follow.
3.4.1 Simultaneous saccharification and fermentation (SSF). Simultaneous saccharification and fermentation (SSF) technique is a single fermentation reactor in order to minimize the production of inhibitors, combining both processes (saccharification and fermentation) to run simultaneously, and to reduce additional equipment cost.114,213 In a single reactor, enzymatic hydrolysis of the biomass is run, and immediately the presented fermentation agent converts the released sugar monomers into ethanol. Moreover, the SSF technique can decrease the production period and enhance the efficiency of the production. Additionally, ethanol accumulation in the reactor does not inhibit the hydrolysis activity, making SSF a favorable method for bioethanol production.15,144 However, this fermentation technique is limited by the respective optimum conditions for both hydrolysis and fermentation, which is quite challenging to control to meet both satisfactory conditions at the same time.214 In common practice, hydrolysis is best carried out at around 50 °C, but fermentation requires warm to mild temperatures for the microbes to remain active, around 28–37 °C, hence to meet both desired conditions requires a special engineering effort. Fig. 6 shows the flowchart of simultaneous saccharification and fermentation process.215
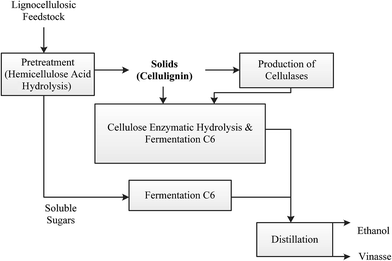 |
| Fig. 6 The flowchart of the simultaneous saccharification and fermentation (SSF) process.215 | |
3.4.2 Separate hydrolysis and fermentation (SHF). The separate hydrolysis and fermentation (SHF) technique is a fermentation process that can be perform in different vessels, where each vessel carries out a specific task.8 SHF allows each both processes, hydrolysis and fermentation at their most optimum conditions and yielding the desired products to the maximum potential.15 For instance, this technique enables hydrolysis to be performed at the optimum temperature of 45–50 °C and fermentation to be performed at about 30 °C without any hassle.8,15 SHF also allows fermentation of sugar based on its type. The hydrolysate flows into the first vessel to ferment the glucose content of the hydrolysate by glucose-fermenting microbes. The process is then continued by distilling the solution and flowing it to the second fermentation vessel to ferment the next sugar type by the specific fermentation microbes.26 SHF technique also reduces the inhibition production since the removal is practical.115 SHF technique is considerably cost effective when it focuses on the substrates, substances employed and the high quality ethanol yield, although it may not be cost effective in the equipment installment.8,216 Fig. 7 shows the flowchart of the separate hydrolysis and fermentation process.215
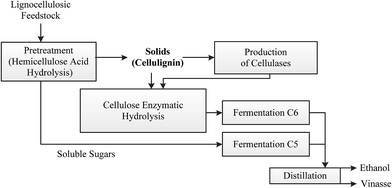 |
| Fig. 7 The flowchart of the separate hydrolysis and fermentation (SHF) process.215 | |
3.4.3 Simultaneous saccharification and co-fermentation (SSCF). This fermentation technique utilizes the integration principle in employing mixed microbes to ferment both more than one sugar type (e.g. pentoses and hexoses) resulting from the previous processes of pretreatment and hydrolysis.8,10 The use of mixed microbes is limited to the respective ability of the microbes, where hexose-fermenting microbes usually grow faster than pentose-fermenting microbes, and this leads to a higher rate of ethanol conversion from hexose.6 SSCF holds several beneficial characteristics in bioethanol production, including a lower enzyme requirement, faster production rate and lesser cost projected since the process is performed in a single reactor.15 On the other hand, SSFC requires extra conscientious controlling since the employed mixed microbes may require different optimum temperatures, as well as between the hydrolysis and fermentation process since they are run in the same reactor.217 C. shehatae, S. cerevisiae, E. coli KO11, E. coli FBR5 are the type of employed microbes to be mixed in SSCF technique to produce bioethanol from varieties of biomass, namely barley hull, wheat straw, etc.1,43,218,219 Fig. 8 describes the flowchart of SSCF process.215
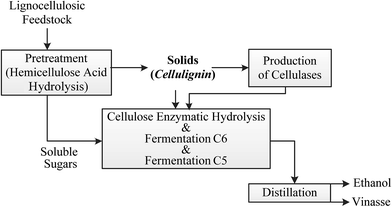 |
| Fig. 8 The flowchart of the simultaneous saccharification and co-fermentation (SSCF) process.215 | |
3.4.4 Consolidated bioprocessing (CBP). Consolidated bioprocessing is a technique in bioethanol production where enzyme production, enzymatic hydrolysis and biomass fermentation are all carried out within the same single reactor. Hence, this technique is usually called direct microbial conversion (DMC).132 In general, CBP employs a specific microorganism type that is capable of conducting such an array of tasks without additional flowing or removal processes to another reactor. In lignocellulosic biomass, for example, production of cellulase enzyme, hydrolysis of cellulose, and fermentation of the yielded sugars are performed by one type of microorganism. This method is a low cost conversion of cellulosic biomass into bioethanol with a high rate and acceptable yields.17,26 Although others have reported that CBP consumes long processing time and an undesirable ethanol yield.1,26 With CBP, external cellulose enzymes are unnecessary for the pretreatment and hydrolysis processes of bioethanol production.26,220 The common microbes employed for this method include Thermoanaerobacter ethanolicus, Clostridium thermoshydrosulfuricum, Thermoanaerobacter mathranii, Thermoanaerobium brockii, Clostridium thermosaccharolyticum. These microbes have good abilities that are utilized in many inexpensive lignocellulosic biomass-to-ethanol conversion and are able to withstand extreme temperature.8 Cardona and Sánchez221 reported the potential yeast strain of K. marxianus for the CBP method and it resulted in a good growth display of endoglucanase and β-glucosidase on the cell surface at temperature as high as 48 °C of which the ethanol was produced from the cellulosic material β-glucan with a yield of 0.47 g ethanol per gram of consumed carbohydrate. However, the CBP technique is not highly recommended since the typical microorganisms employed for CBP are intolerant of high ethanol yields, hence further microbial engineering is strongly recommended to upgrade the applicability of this technique.222,223 Fig. 9 describes the flowchart of consolidated bioprocessing process.215
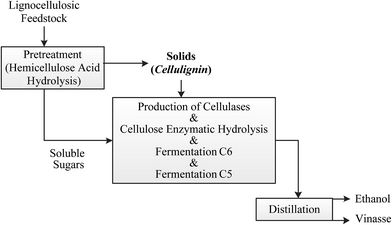 |
| Fig. 9 The flowchart of the consolidated bioprocessing (CBP) process.215 | |
3.5 Distillation and dehydration
Distillation in bioethanol production is required to separate the ethanol content out of the fermented mixture by utilizing the boiling point of ethanol (78.3 °C). In other words, in water–ethanol distillation, ethanol will be vaporized before water.16,224 In a typical bioethanol refinery, the fermentation product is flowed to the distillation column to be distilled, and the product from this process is then flowed to the rectifier and concentrated to just below the azeotropic point, as seen in Fig. 10 which shows the water–ethanol azeotropic point.109,225 Meanwhile, the remaining un-distilled fermented product is flowed to a stripping column to additionally remove the water content. Afterwards, the product of this process is combined with the ethanol produced from the previous process.226 As the result, bioethanol recovery produces quality about 99.6%.67 The solid compounds of the product are separated using centrifuge.227 Distillation or bioethanol recovery process is an energy intensive process which requires high volumes of cooling water and produce some gallons of water used for every gallon of ethanol obtained.108
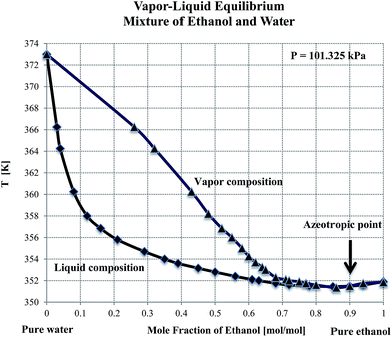 |
| Fig. 10 Water–ethanol equilibrium mixture and azeotropic point.109,225 | |
Dehydration is advantageous to remove the remaining water in the azeotropic phase.107,228 The dehydration technique is quite similar to extractive distillation but this process utilizes an additional component which lowers the heterogeneous boiling azeotrope.229 Prior to the dehydration process, the ethanol is first distilled to achieve above 96%, then filtered through molecular sieves, which absorbs the water component from the mixture.109 The result of this process is an upgraded ethanol product. The used molecular sieves can be recycled by heating the sieves to remove the absorbed water.114
Overall, the literature for bioethanol production from biomass feedstock are tabulated in Tables 11230–237 and 12.238–243
Table 11 Comparison of bioethanol production for first generation biomass feedstock
Biomass |
Fermentation process |
Results (ethanol concentration) |
Ref. |
Sugarcane (Saccharum officinarum) |
Escherichia coli KO11 and Klebsiella oxytoca P2 |
39.4–42.1 g L−1 |
230 |
Sweet sorghum (Sorghum bicolor) |
Kluyveromyces marxianus DMKU 3-1042 |
7.43% w/v at 37 °C |
234 |
Wheat (Triticum vulgare) |
Pichia kudriavzevii |
71.95 g L−1 at 40 °C |
233 |
Sugar beet (Beta vulgaris) |
S. cerevisiae Ethanol Red and S. cerevisiae safdistill C-70 |
85.0–87.0 g L−1 |
235 |
S. cerevisiae IR-2, S. cerevisiae ATCC 26603, S. cerevisiae IFO 0309 and Z. mobilis IFO 13756 |
0.44 g g−1 and the lowest productivity of 0.08 g h−1 L−1 by Z. mobilis |
232 |
Sweet sorghum (Sorghum bicolor) |
S. cerevisiae CFTR 01 and SG |
9.0% w/v by Keller variety |
231 |
S. cerevisiae ATCC 7754 and Z. mobilis ATCC 29191 |
50.26 mL L−1 |
237 |
Watermelon (Citrullus lanatus) |
S. cerevisiae Ethanol Red |
25.0% w/v at pH 3.0 and 35.0% w/v at pH 5.0 |
236 |
Table 12 Comparison of pretreatment process for second generation biomass feedstock
Biomass |
Pre-treatment |
Hydrolysis |
Results |
Ref. |
Sugarcane bagasse |
Ball milling (4 h) |
Acremonium cellulase at 5 FPU g−1 substrate of cellulase and 20 U g−1 substrate of xylanase from optimash BG at 45 °C, pH 5.0 for 72 h |
Glucose of 89.2 ± 0.7% |
238 |
Xylose of 77.2 ± 0.7% |
H2SO4 of 1% vol at 60 °C for 24 h |
In an autoclave at 121 °C for 40 min after removing the excess H2SO4 of 1% vol |
Total sugar of 68.0 g L−1 |
239 |
Wheat straw |
Knife milling with 0.7–1.0 mm and washed with water and dried |
H2SO4 of 1.85% vol at 90 °C with for 18 h which 20 : 1 of liquid to solid ration. Suspension centrifuged and the residue is washed with hot water |
Glucose of 1.70 ± 0.30 g L−1 |
240 |
Xylose of 12.80 ± 0.25 g L−1 |
Rice straw |
Chopped to 5–6 mm |
H2SO4 of 4.4% vol at 1 : 10 solid to liquid ration in boiling water bath for 1 h. The process was filtered and pH was adjusted to 5.5 |
Total sugar of 20 g L−1 |
241 |
Chopped and steam exploded at 3.5 MPa, 275 °C for 2 min |
Saccharification used cytolase, novozyme at 50 °C for 120 h |
Xylose of 5–10 g L−1 |
242 |
Maize straw |
NaOH 2% wt at 80 °C for 1 h |
Hydrolysis by cellulase of trichoderma reesei ZU-02 and cellobiose of Aspergillus niger ZU-07 |
Xylose of 23.6 g L−1 |
243 |
Glucose of 56.7 g L−1 |
Arabinose of 5.7 g L−1 |
4. Fuel properties bioethanol
The quality of combustion engine fuel can be identified through the chemical and physical properties of the fuel.244 The standardized properties for bioethanol are listed under ASTM D4806 standard, and the comparison with gasoline is given respectively in Tables 13245–247 and 14.247–249
Table 13 Bioethanol properties based on ASTM D4806 (ref. 245–247)
Property |
Specification |
ASTM test method |
Ethanol min, (% vol) |
92.1 |
D5501 |
Methanol, max, (% vol) |
0.5 |
— |
Solvent-washed gum max, (mg/100 mL) |
5.0 |
D381 |
Water content, max, (% vol) |
1.0 |
E203 |
Denaturant content, min, (% vol) |
1.96 |
— |
Denaturant content, max, (% vol), |
4.76 |
— |
Inorganic chloride content, max, (mg L−1) |
40 |
D512 |
Copper content max, (mg kg−1) |
0.1 |
D1688 |
Acidity (as acetic acid CH3COOH) max, (mg L−1) |
0.007 |
D1613 |
pHe |
6.5–9.0 |
D6423 |
Appearance-visibly free of suspended or precipitated contaminants (clear & bright) |
|
|
Table 14 Properties of bioethanol and gasoline247–249
Property |
Ethanol |
Gasoline |
Chemical formula |
C2H5OH |
C4 to C12 |
Molecular weight (g mol−1) |
46.07 |
100–105 |
Carbon (% wt) |
52.2 |
85–88 |
Hydrogen (% wt) |
13.1 |
12–15 |
Oxygen (% wt) |
34.7 |
0 |
Specific gravity |
0.794 |
0.7–0.78 |
Density @ 15 °C, (kg m−3) |
785–809.9 |
750–765 |
Boiling temperature, (°C) |
78 |
27–225 |
Reid vapor pressure @ 37.8 °C, (kPa) |
17 |
53–60 |
Research octane no. |
108 |
90–100 |
Motor octane no. |
92 |
81–90 |
(R + M)/2 |
100 |
86–94 |
Cetane no. |
— |
5–20 |
Fuel in water, (%vol) |
100 |
0 |
Water in fuel, (% vol) |
100 |
0 |
Freezing point, (°C) |
−114 |
−40 |
Kinematic viscosity (mm2 s−1) |
1.2–1.5 |
0.5–0.6 |
Flash point, closed cup, (°C) |
12 |
−42 |
Auto ignition temperature, (°C) |
423 |
257 |
Lower heating value (MJ kg−1) |
26.9 |
44 |
Higher heating value (MJ kg−1) |
29.7 |
47.3 |
Vapor Flammability limits, (% vol) |
3.5–15 |
0.6–8 |
Specific heat, liquid (kJ kg−1 K−1) |
1.7 |
2.4 |
Specific heat, vapor (kJ kg−1 K−1) |
1.93 |
2.5 |
Stoichiometric air/fuel ratio |
9 |
14.5–14.7 |
Fuel in vaporized stoichiometric mixture, (% vol) |
6.5 |
2 |
The detailed discussion of some of the properties related to bioethanol and gasoline are discussed below.
4.1 Ethanol yield
Ethanol yield is the parameter to evaluate the performance of the feedstock in bioethanol production.250 Ethanol yield can be described as theoretical and actual/experimental ethanol yield. Theoretical ethanol yield is obtained through stoichiometry calculation of the fermentable sugars, and is usually used as comparison factor. Meanwhile, actual ethanol yield is obtained from the actual production process of sugars fermentation, which is derived from the employed biomass. Actual ethanol yield depends on the conditions of fermentation, especially on the employed fermentation agents.251 Therefore, the feedstock and microorganism selection were employed in the ethanol production in order to produce high yield and good ethanol quality. The concentration of the produced ethanol can be detected by checking their corresponding refractive indexes obtained from the refractometer readings.252 The standard of ethanol yield or content for bioethanol is ASTM D4806.253
4.2 Acidity or alkalinity
The acidity or alkalinity of bioethanol is tested by reacting it with phenolphthalein to test whether the solution is alkali or acid, and the acidity is expressed as a percentage by mass of acetic acid.254 The acidity equation is given as below:254 |
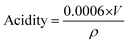 | (1) |
where: V = the volume of the NaOH solution (in mL), ρ = the density of the sample at 20 °C (in g mL−1), 0.0006 = the mass of CH3COOH corresponding to 1 mL of NaOH (in g).
The acidity or alkalinity of bioethanol describes the concentration of acid-type compounds that are present in the tested solution, and is usually regarded as total acid number.255 High acid content can cause the formation of gums and lacquers on metal surfaces, increased viscosity that impairs oil circulation, and also cause engine corrosion.256 ASTM Standard D1613 is the standard to measure the acidity or alkalinity of bioethanol. According to this standard, acidity or alkalinity of bioethanol has maximum value of 0.007 mg L−1.257
4.3 Water content
The stability of ethanol depends on the chemical composition of the bioethanol, water content and temperature.258 Water content in ethanol is generally related to the two types of purities: (i) anhydrous, where water content obtained is less than 1%; (ii) hydrous, where water content possession is ranged 5–10% in the ethanol.248 Water contamination in ethanol is possible since ethanol has a hygroscopic nature, which absorbs water from the atmosphere when stored in open container.259 The water content in the bioethanol can be measured in accordance to ASTM E203, where this standard describes the maximum specification of 1.000% vol s.246
4.4 Denatured content
Denatured ethanol contains certain additives that can make it inconsumable and toxic, which is developed to prohibit recreational consumption. The additives also give a bad taste and foul smell to the ethanol.247 Foul-tasting or toxic substances are added for about 10% of the produced ethanol after distillation to accomplish this parameter. Denatured ethanol also can be blended with gasoline to improve the octane number of gasoline.248 The denatured ethanol is set to a minimum value of 1.960% vol and maximum value of 4.760% vol according to ASTM D 4806.246
4.5 pHe
The pHe is the pH of a denatured fuel ethanol, and it is quite difficult to measure this property since high quality denatured ethanol is not an aqueous solution.260 Typically, the pHe test is conducted after the denaturing process and corrosion inhibitors addition.261 The ASTM D6423 method describes the pHe test, which covers rehydration specification of the probe between readings and repeatability for same operator, same apparatus and identical test material.247 The range of pHe of bioethanol is 6.5–9.0 with 90% confidence factor and reproducibility of 0.52.259,260
4.6 Octane number
A measurement of fuel resistivity towards denotation and self-ignition is described as octane number.262 Octane number can be rated as motor octane number (MON) or research octane number (RON), which both concludes the behavior of particular fuel combustion in an engine during high or steady load condition.105,263 As an excellent anti-detonating additive, bioethanol can improve the octane number of gasoline-base fuel.263,264 The octane number of bioethanol is specified by ASTM D2700, which are MON is 92; RON is 108; and bioethanol–gasoline blend ranges of MON is 81–90 and RON is 90–100.248,249
The comparison properties of bioethanol and bioethanol gasoline blends are summarized in Tables 15251,253,265–269 and 16.253,269–274
Table 15 The comparison properties of bioethanol from various biomass feedstock
Properties |
Unit |
Sugar molasses269 |
Sugarcane266 |
Farmstead253 |
Cassava tubers265 |
Cassava flours251 |
Palm juice268 |
Sweet sorghum syrup267 |
Boiling point |
°C |
78.5 |
78.4 |
— |
78.5 |
— |
— |
78 |
Heat of combustion |
MJ L−1 |
23.625 |
— |
— |
— |
— |
— |
— |
Heat of vaporization |
kJ mol−1 |
33.74 |
— |
— |
— |
— |
— |
— |
Lower heating value |
kJ cm−3 |
— |
— |
— |
— |
— |
— |
21.09 |
Octane rating |
— |
106–108 |
— |
— |
— |
— |
— |
— |
Stoichiometric air/fuel ratio |
— |
9/1 |
— |
— |
— |
— |
— |
— |
Concentration |
— |
99.6–99.8 |
— |
— |
— |
98 |
96 |
— |
pH |
— |
— |
— |
— |
5–6 |
6.78 |
3–4 |
5.0 |
Acidity |
mg L−1 |
≤30 |
— |
— |
— |
— |
≤36 |
— |
Water content |
% vol |
≤0.3 |
0.378 |
6.94 |
— |
— |
— |
— |
Viscosity at 40 °C |
mm2 s−1 |
— |
— |
— |
— |
2.2 |
— |
— |
Density @ 20 °C |
kg m−3 |
789.0 |
791 |
816.2 at 15 °C |
791.0 |
— |
795.0 at 25 °C |
785.0 |
Point of humidity |
— |
<6.5 |
— |
— |
— |
— |
— |
— |
Flammability limits |
°C |
— |
— |
— |
— |
— |
— |
13–42 |
Flash point |
°C |
— |
13–14 |
24.5 |
— |
23 |
— |
13 |
Vapor pressure |
kPa |
— |
— |
14.5 |
— |
— |
— |
17 |
Auto ignition temperature |
°C |
— |
— |
— |
— |
— |
— |
366 |
Distillation |
% vol |
— |
— |
— |
— |
78–100 |
— |
— |
Table 16 The properties of bioethanol gasoline blends
Property |
Unit |
Test method |
Gasoline |
Farmstead253 |
Sugar molasses269 |
Cassava270 |
E58.9 |
E60 |
E10 |
E15 |
E20 |
E25 |
E30 |
E5 |
E10 |
E15 |
Density @ 20 °C |
kg m−3 |
ASTM D1298 |
745.4 |
917.0 |
908.0 |
739.6 |
749.5 |
754.1 |
757.1 |
761.3 |
740.8 |
743.9 |
746.9 |
Flash point |
°C |
ASTM D93 |
— |
24.5 |
24.5 |
— |
— |
29.2 |
30.0 |
29.2 |
— |
— |
— |
Heating value |
MJ kg−1 |
ASTM D240 |
42.54 |
— |
— |
— |
— |
— |
— |
— |
43.45 |
42.51 |
41.58 |
Auto ignition temperature |
°C |
— |
246.0 |
— |
— |
— |
— |
— |
— |
— |
— |
— |
— |
Research octane number |
— |
ASTM D2699 |
91.3 |
— |
— |
97.1 |
98.6 |
100.4 |
99.5 |
102.5 |
— |
— |
— |
Motor octane number |
— |
ASTM D2700 |
84.0 |
— |
— |
— |
— |
— |
— |
— |
— |
— |
— |
Distillation |
|
ASTM D86 |
|
|
|
|
|
|
|
|
|
|
|
(a) Initial boiling point |
% vol |
|
38.8 |
— |
— |
— |
— |
— |
— |
— |
— |
— |
— |
(b) 10% evaporated |
% vol |
|
68.5 |
— |
— |
— |
— |
— |
— |
— |
— |
— |
— |
(c) 50% evaporated |
% vol |
|
109.6 |
— |
— |
— |
—— |
— |
— |
— |
— |
— |
— |
(d) 99% evaporated |
% vol |
|
161.5 |
— |
— |
— |
— |
— |
— |
— |
— |
— |
— |
Property |
Unit |
Test method |
Gasoline |
Cassava273 |
E10 |
E20 |
E30 |
E40 |
E50 |
E60 |
E70 |
E80 |
E90 |
E100 |
Density @ 20 °C |
kg m−3 |
ASTM D1298 |
745.4 |
750.8 |
760.5 |
778.2 |
779.2 |
780.5 |
781.2 |
782.3 |
783.4 |
784.0 |
789.0 |
Flash point |
°C |
ASTM D93 |
— |
−40 |
−20 |
−15 |
−13.5 |
−5.0 |
−1.0 |
0.0 |
5.0 |
8.5 |
12.5 |
Heating value |
MJ kg−1 |
ASTM D240 |
42.54 |
— |
— |
— |
— |
— |
— |
— |
— |
— |
— |
Auto ignition temperature |
°C |
— |
246.0 |
260 |
279 |
281 |
294 |
320 |
345 |
350 |
362 |
360 |
365 |
Research octane number |
— |
ASTM D2699 |
91.3 |
93 |
94 |
95 |
97 |
99 |
100 |
103 |
104 |
106 |
129 |
Motor octane number |
— |
ASTM D2700 |
84.0 |
— |
— |
— |
— |
— |
— |
— |
— |
— |
— |
Distillation |
|
ASTM D86 |
|
|
|
|
|
|
|
|
|
|
|
(a) Initial boiling point |
% vol |
|
38.8 |
— |
— |
— |
— |
— |
— |
— |
— |
— |
— |
(b) 10% evaporated |
% vol |
|
68.5 |
— |
— |
— |
— |
— |
— |
— |
— |
— |
— |
(c) 50% evaporated |
% vol |
|
109.6 |
— |
— |
— |
— |
— |
— |
— |
— |
— |
— |
(d) 99% evaporated |
% vol |
|
161.5 |
— |
— |
— |
— |
— |
— |
— |
— |
— |
— |
Property |
Unit |
Test method |
Gasoline |
Potato waste271 |
Cassava272 |
Sugarcane274 |
E5 |
E10 |
E15 |
E20 |
E6 |
E10 |
E15 |
E20 |
E20 |
Density @ 20 °C |
kg m−3 |
ASTM D1298 |
745.4 |
— |
— |
— |
— |
754 |
757.6 |
758.6 |
759.7 |
759.7 |
Flash point |
°C |
ASTM D93 |
— |
— |
— |
— |
— |
— |
— |
— |
— |
— |
Heating value |
MJ kg−1 |
ASTM D240 |
42.54 |
— |
— |
— |
— |
41.41 |
40.8 |
40.08 |
39.47 |
39.47 |
Auto ignition temperature |
°C |
— |
246.0 |
— |
— |
— |
— |
— |
— |
— |
— |
— |
Research octane number |
— |
ASTM D2699 |
91.3 |
89.7 |
92.3 |
94 |
99.4 |
92.8 |
93.0 |
92.4 |
93.0 |
93 |
Motor octane number |
— |
ASTM D2700 |
84.0 |
— |
— |
— |
— |
84 |
83.8 |
82.8 |
83.4 |
83.4 |
Distillation |
|
ASTM D86 |
|
|
|
|
|
|
|
|
|
|
(a) Initial boiling point |
% vol |
|
38.8 |
40.9 |
38.9 |
44 |
40.8 |
42.6 |
47.1 |
43.2 |
40.5 |
40.5 |
(b) 10% evaporated |
% vol |
|
68.5 |
54.3 |
53.1 |
57.2 |
55.4 |
61.3 |
65.1 |
62.7 |
62.4 |
62.4 |
(c) 50% evaporated |
% vol |
|
109.6 |
93.5 |
71.9 |
71.4 |
71.6 |
111.4 |
98.3 |
87.6 |
79.2 |
79.2 |
(d) 99% evaporated |
% vol |
|
161.5 |
184.1 |
175.1 |
182.4 |
176.6 |
206.1 |
191.6 |
203.6 |
201.4 |
201.4 |
5. Engine performance and emissions using bioethanol and the blends
As one of renewable energy resources obtained from biomass, bioethanol has been tested intensively in internal combustion engines. Bioethanol is a viable alternative to unleaded gasoline fuel as automotive fuel, and it can be used without modified engines.271,275,276 The application of bioethanol in spark-ignited engines has been studied and assessed through its combustion performance parameters, including brake thermal efficiency, brake specific fuel consumption, and engine power, which brings the bioethanol viability as a biofuel closer to the common practice. Additionally, gasoline bioethanol blends which substitute as fuel can enhance the blend property qualities and combustion performance in engines. Ghazikhani et al.270 analyzed the performance of two stroke SI engine using a gasoline–ethanol blend, which resulted in a CO emission reduction by 32% from an ethanol concentration of 15% in the blend. Similarly, Schifter et al.272 observed that for a 20% ethanol blend in a single cylinder engine, the CO emission was reduced by 52%. In a four-stroke SI engine, Najafi et al.271 investigated the performance of the engine fueled by gasoline–ethanol blends, and they observed that E20 enhanced the combustion efficiency by 30% and CO emitted was at 45.42%, compared to pure gasoline fuel. Using a higher ethanol ratio in the blend, Koç, et al.277 studied a single-cylinder SI engine combustion performance powered by blends E50 and E85. The authors reported that brake specific fuel consumption (BSFC) was improved to 20.3% and 45.6%, and NOx emission was decreased to 14.29% and 11.22% for E50 and E85 respectively. The benefit of blending fossil fuels with bioethanol is also eligible for diesel engines. As reported by Gomasta and Mahla,275 the engine performance efficiency was increased to 13% for ethanol–diesel blends, and hydrocarbon (HC) emissions were reduced to 35% at full load for 20% ethanol ratio in the blend compared to pure diesel fuel. The summary of engine performance and emissions productions using ethanol blends are presented in Table 17.270–272,275,277–279
Table 17 The summary of engine performance and emissions using ethanol blendsa
Engine |
Fuel type |
Test condition |
Engine performance analysis |
Emissions analysis |
Ref. |
BSFC |
BTE |
BP |
CO2 |
CO |
HC |
NOx |
BSFC = brake specific fuel consumption; BTE = brake thermal efficiency; BP = brake power; C = cylinder; ↓ = decrease; ↑ = increase; E0 = 100% vol gasoline; E5 = 5 vol% ethanol–95 vol% gasoline; gasoline; DME1 = 1 vol% dimethyl ether–99 vol% ethanol; E69.5 + 0.5 = 69.5 vol% ethanol–0.5 vol% cycloheptanol–30 gasoline; SI = spark ignition; CI = compression ignition. |
1C, two stroke, SI |
E0, E5, E10, E15 |
2500–4500 rpm |
BSFC↓ |
— |
— |
6.3%↓ |
32%↓ |
6%↓ |
38%↓ |
270 |
1C, four stroke, SI |
E0, E6, E10, E15, E20 |
2000 rpm |
BSFC↑ |
— |
— |
— |
52%↓ |
19%↓ |
60%↑ |
272 |
4C, four stroke, SI |
E0, E5, E10, E15, E20 |
1000–5000 rpm |
BSFC↓ |
30%↑ |
BP↑ |
10.41% |
45.42% |
31.69%↓ |
45.55%↑ |
271 |
1C, four stroke, SI |
E0, E50, E85 |
1500–5000 rpm |
Min. 20.3% and max. 45.6%↑ |
— |
BP↑ |
— |
1%↓ |
24%↓ |
— |
277 |
4C, four stroke, SI |
DME0, DME1, DME2 |
1400 rpm |
— |
Max 10%, ↑ |
— |
— |
— |
702 ppm↓ |
1054 ppm↑ |
278 |
1C, four stroke, CI |
E0, E5, E10, E15, E20 |
1500 rpm |
BSFC↓ |
13%↓ |
— |
— |
38%↓ |
35%↓ |
— |
275 |
4C, four stroke, SI |
E69.5 + 0.5, E64.6 + 0.4, E59.7 + 0.3, E49.8 + 0.2 |
2000–2800 rpm |
— |
31.89%↑ |
— |
8%↓ |
0.08%↓ |
— |
— |
279 |
6. Conclusion
Bioethanol is gaining interest for its ability to fit in the current energy crisis. Bioethanol is produced diversely according to the feedstock type, although in general it follows a full sequence of pretreatment, hydrolysis, fermentation and distillation. In first generation bioethanol production, sugar-rich food crops are treated, hydrolyzed and fermented to produce ethanol. Besides that, agricultural crop residues can be utilized for second generation production. Moreover, the lack of source availability of first and second generation led researchers to explore new feedstocks in bioethanol production, utilizing micro and macro algae due to their favorable rapid growth rate. This advances the technology to give more available methods and techniques, for instance pretreatment processes can be performed by tens of different techniques. The increased variety improves the ability to select the most suitable routes that match with one’s desired requirements and outcomes from the available sources. Furthermore, the suitability of gasoline bioethanol blends as an alternative fuel in spark-ignited engine is characterized by the fairness of its fuel properties. The properties of gasoline bioethanol blends such as an ethanol purity ratio of 99%, pHe of 6.5–9.0, water content of 1.0%, density of 785–809.9 were ruled according to ASTM standard. The gasoline bioethanol blends within these properties are suitable as an alternative fuel in spark-ignited engine.
This review discusses the available methods for the production of bioethanol as an alternative fuel with tremendous potential. It also suggests that bioethanol production should not only be focused on the feedstock potential and the selection of production route, but also it encourages other practical aspects when it comes to actual realization, especially the environmental impact. The feedstock exploration for bioethanol production is expandible, which means there is a lot of room for improvement and a large number of combinations of possible production paths to be sorted and arranged for suitable feedstocks. Bioethanol is indeed considered as the most influential variable for the transportation sector, since with its benefits bioethanol will bring independence from the non-renewable and more heavily polluting fossil fuels. Finally, bioethanol is one solution for the energy and environmental crisis and it is suggested to have a good synergy from both technological developments and supporting energy policies to solve energy concerns.
Acknowledgements
The authors gratefully acknowledge the financial support provided by the Ministry of Education of Malaysia and University of Malaya, Kuala Lumpur, Malaysia, under the High Impact Research Grant (Project title: Clean diesel technology for military and civilian transport vehicles, Grant no.: UM.C/HIR/MOE/ENG/07 D000007-16001), University Malaya Research Grant Scheme (Grant no.: RP022A-13AET), Fundamental Research Grant Scheme (Grant no.: FP009-2014A), SATU (Grant no.: RU021A-2015) and Postgraduate Research Grant (Grant no.: PPP-PG014-2015A).
References
- A. Gupta and J. P. Verma, Sustainable bio-ethanol production from agro residues a review, Renewable Sustainable Energy Rev., 2015, 41, 550–567 CrossRef CAS.
- M. Klein, I. N. Pulidindi, N. Perkas, E. Meltzer-Mats, A. Gruzman and A. Gedanken, Direct production of glucose from glycogen under microwave irradiation, RSC Adv., 2012, 2, 7262–7267 RSC.
- A. Szklo, R. Schaeffer and F. Delgado, Can one say ethanol is a real threat to gasoline?, Energy Policy, 2007, 35, 5411–5421 CrossRef.
- E. Gnansounou, Production and use of lignocellulosic bioethanol in Europe current situation and perspectives, Bioresour. Technol., 2010, 101, 4842–4850 CrossRef CAS PubMed.
- C. Park, Y. Choi, C. Kim, S. Oh, G. Lim and Y. Moriyoshi, Performance and exhaust emission characteristics of a spark ignition engine using ethanol and ethanol-reformed gas, Fuel, 2010, 89, 2118–2125 CrossRef CAS.
- A. M. Brownstein, Ethanol from cellulose, Renewable Motor Fuels, 2015, 23–32 Search PubMed.
- P. Binod, R. Sindhu, R. R. Singhania, S. Vikram, L. Devi, S. Nagalakshmi, N. Kurien, R. K. Sukumaran and A. Pandey, Bioethanol production from rice straw an overview, Bioresour. Technol., 2010, 101, 4767–4774 CrossRef CAS PubMed.
- N. Sarkar, S. K. Ghosh, S. Bannerjee and K. Aikat, Bioethanol production from agricultural wastes an overview, Renewable Energy, 2012, 37, 19–27 CrossRef CAS.
- L. R. Lynd, Overview and evaluation of fuel ethanol from cellulosic biomass technology, economics, the environment and policy, Annu. Rev. Energy, 1996, 21, 403–465 CrossRef.
- B. Cristian Teodor, G. Gil and V. Camelia, Bioethanol production from residual lignocellulosic materials a review part 2, Food Technol., 2013, 37, 25–38 Search PubMed.
- N. Türköz, B. Erkuş, M. İhsan Karamangil, A. Sürmen and N. Arslanoğlu, Experimental investigation of the effect of E85 on engine performance and emissions under various ignition timings, Fuel, 2014, 115, 826–832 CrossRef.
- S. A. Shahir, H. H. Masjuki, M. A. Kalam, A. Imran, I. M. R. Fattah and A. Sanjid, Feasibility of diesel biodiesel ethanol/bioethanol blend as existing CI engine fuel: an assessment of properties, material compatibility, safety and combustion, Renewable Sustainable Energy Rev., 2014, 32, 379–395 CrossRef CAS.
- E. Torres Jimenez, M. Pilar Dorado and B. Kegl, Experimental investigation on injection characteristics of bioethanol diesel fuel and bioethanol biodiesel blends, Fuel, 2011, 90, 1968–1979 CrossRef CAS.
- P. Aradhana, G. Sandip, V. Savita and G. Anurag, Ethanol diesel and biodiesel blend as substitute fuel to CI engines: a comprehensive review, Int. J. Res. Appl. Sci. Eng. Tech., 2013, 3, 2250–2459 Search PubMed.
- C. R. Soccol, L. P. D. S. Vandenberghe, A. B. P. Medeiros, S. G. Karp, M. Buckeridge, L. P. Ramos, A. P. Pitarelo, V. Ferreira-Leitão, L. M. F. Gottschalk, M. A. Ferrara, E. P. D. Silva Bon, L. M. P. D. Moraes, J. D. A. Araújo and F. A. G. Torres, Bioethanol from lignocelluloses: Status and perspectives in Brazil, Bioresour. Technol., 2010, 101, 4820–4825 CrossRef CAS PubMed.
- M. Balat, H. Balat and C. Öz, Progress in bioethanol processing, Prog. Energy Combust. Sci., 2008, 34, 551–573 CrossRef CAS.
- A. Limayem and S. C. Ricke, Lignocellulosic biomass for bioethanol production current perspectives, potential issues and future prospects, Prog. Energy Combust. Sci., 2012, 38, 449–467 CrossRef CAS.
- N. Patni, S. G. Pillai and A. H. Dwivedi, Wheat as a promising substitute of corn for bioethanol production, Procedia Eng., 2013, 51, 355–362 CrossRef CAS.
- N. Linoj Kumar, D. Prabha, G. Anandajit and M. Sameer, Liquid biofuels in South Asia resources and technologies, Asian Biotechnol. Dev. Rev., 2006, 8, 31–49 Search PubMed.
- V. R. Lebaka, Biofuel Technol, Springer, 2013, vol. 9, pp. 223–258 Search PubMed.
- P. Bajpai, Advances in bioethanol, Springer, 2013, pp. 1–90 Search PubMed.
- H. Zabed, G. Faruq, J. N. Sahu, M. S. Azirun, R. Hashim and A. Nasrulhaq Boyce, Bioethanol production from fermentable sugar juice, Sci. World J., 2014, 2014, 11 Search PubMed.
- K. Zuber and K. D. Anjani, Fermentation of biomass for production of ethanol a review, Univers. J. Environ. Res. Technol., 2013, 3, 1–13 Search PubMed.
- A. Tesfaw and F. Assefa, Current trends in bioethanol production by Saccharomyces cerevisiae: substrate, inhibitor reduction, growth variables, coculture and immobilization, Int. Scholarly Res. Not., 2014, 11 Search PubMed.
- F. J. Davila Gomez, C. Chuck Hernandez, E. Perez Carrillo, W. L. Rooney and S. O. Serna Saldivar, Evaluation of bioethanol production from five different varieties of sweet and forage sorghums (Sorghum bicolor (L.) Moench), Ind. Crops Prod., 2011, 33, 611–616 CrossRef CAS.
- M. Vohra, J. Manwar, R. Manmode, S. Padgilwar and S. Patil, Bioethanol production feedstock and current technologies, J. Environ. Chem. Ecotoxicol., 2014, 2, 573–584 CAS.
- Ó. J. Sánchez and C. A. Cardona, Trends in biotechnological production of fuel ethanol from different feedstocks, Bioresour. Technol., 2008, 99, 5270–5295 CrossRef PubMed.
- B. Janine, Production of ethanol from tropical sugar beet, Chemical and Minerals Engineering, 2010, 1–150 Search PubMed.
- A. S. Bennett and R. P. Anex, Production, transportation and milling costs of sweet sorghum as a feedstock for centralized bioethanol production in the upper Midwest, Bioresour. Technol., 2009, 100, 1595–1607 CrossRef CAS PubMed.
- A. Almodares and M. R. Hadi, Production of bioethanol from sweet sorghum a review, Afr. J. Agric. Res., 2009, 4, 772–780 Search PubMed.
- R. Cifuentes, R. Bressani and C. Rolz, The potential of sweet sorghum as a source of ethanol and protein, Energy Sustainable Dev., 2014, 21, 13–19 CrossRef CAS.
- I. A. Olukoya, D. Bellmer, J. R. Whiteley and C. P. Aichele, Evaluation of the environmental impacts of ethanol production from sweet sorghum, Energy Sustainable Dev., 2015, 24, 1–8 CrossRef CAS.
- G. Fernandes, T. G. Braga, J. Fischer, R. A. C. Parrella, M. M. de Resende and V. L. Cardoso, Evaluation of potential ethanol production and nutrients for four varieties of sweet sorghum during maturation, Renewable Energy, 2014, 71, 518–524 CrossRef CAS.
- C. V. Ratnavathi, S. K. Chakravarthy, V. V. Komala, U. D. Chavan and J. V. Patil, Sweet sorghum as feedstock for biofuel production a review, Sugar Tech, 2011, 13, 399–407 CrossRef CAS.
- M. Kim and D. Day, Composition of sugar cane, energy cane, and sweet sorghum suitable for ethanol production at Louisiana sugar mills, J. Ind. Microbiol. Biotechnol., 2011, 38, 803–807 CrossRef CAS PubMed.
- D. J. Pejin, V. M. Vučurović, S. D. Popov, J. M. Dodić and S. N. Dodić, Production of ethanol from kantata wheat variety, Acta Period. Technol., 2006, 155–161 CrossRef CAS.
- G. Punter, D. Rickeard, J. Larivé, R. Edwards, N. Mortimer, R. Horne, A. Bauen and J. Woods, Well-to-wheel evaluation for production of ethanol from wheat, Fuels Working Group, 2004, 1–40 Search PubMed.
- A. Shi, Z. Du, X. Ma, Y. Cheng, M. Min, S. Deng, P. Chen, D. Li and R. Ruan, Production and evaluation of biodiesel and bioethanol from high oil corn using three processing routes, Bioresour. Technol., 2013, 128, 100–106 CrossRef CAS PubMed.
- N. Voca, B. Varga, T. Kricka, D. Curic, V. Jurisic and A. Matin, Progress in ethanol production from corn kernel by applying cooking pre-treatment, Bioresour. Technol., 2009, 100, 2712–2718 CrossRef CAS PubMed.
- D. Pimentel and T. Patzek, Ethanol production using corn, switchgrass and wood biodiesel production using soybean and sunflower, Nat. Resour. Res., 2005, 14, 65–76 CrossRef CAS.
- M. Han, K. E. Kang, Y. Kim and G.-W. Choi, High efficiency bioethanol production from barley straw using a continuous pretreatment reactor, Process Biochem., 2013, 48, 488–495 CrossRef CAS.
- R. S. Bhatty, Glucan and flour yield of hull less barley, Cereal Chem., 1999, 76, 314–315 CrossRef CAS.
- T. H. Kim, F. Taylor and K. B. Hicks, Bioethanol production from barley hull using SAA (soaking in aqueous ammonia) pretreatment, Bioresour. Technol., 2008, 99, 5694–5702 CrossRef CAS PubMed.
- Z. Li, Y. Liu, W. Liao, S. Chen and R. S. Zemetra, Bioethanol production using genetically modified and mutant wheat and barley straws, Biomass Bioenergy, 2011, 35, 542–548 CrossRef CAS.
- P. R. Shewry, Wheat, J. Exp. Bot., 2009, 60, 1537–1553 CrossRef CAS PubMed.
- Health and Ageing Australian Government, The biology of Zea mays L. spp. mays (maize or corn), Gene Technol Regulator, 2008, 1–81 Search PubMed.
- NIIR Board of Consultants & Engineers, Wheat, rice, corn, oat, barley and sorghum processing handbook (cereal food technology), Asia Pacific Business, 2006, 1–464 Search PubMed.
- N. S. Mosier and K. E. Ileleji, How fuel ethanol is made from corn, Bioenergy: Biomass to Biofuels, 2014, 1, 337–339 Search PubMed.
- V. Okudoh, C. Trois, T. Workneh and S. Schmidt, The potential of cassava biomass and applicable technologies for sustainable biogas production in South Africa: A review, Renewable Sustainable Energy Rev., 2014, 39, 1035–1052 CrossRef.
- P. Li and M. Zhu, A consolidated bio-processing of ethanol from cassava pulp accompanied by hydrogen production, Bioresour. Technol., 2011, 102, 10471–10479 CrossRef CAS PubMed.
- M. Sanette and Y. N. Tando, Cassava as feedstock for ethanol production in South Africa, Afr. J. Biotechnol., 2013, 12, 4975–4983 CrossRef.
- J. Roberto, F. Rañola, R. B. Demafelis, E. D. Rosario and B. G. Bataller, Enhancing the viability of cassava feedstock for bioethanol in the Philippines, ISSAAS J., 2009, 15, 147–158 Search PubMed.
- C. Jansson, A. Westerbergh, J. Zhang, X. Hu and C. Sun, Cassava, a potential biofuel crop in (the) People’s Republic of China, Appl. Energy, 2009, 86, 95–98 CrossRef.
- C. R. Soccol, Biotechnology products from cassava root by solid state fermentation, J. Sci. Ind. Res., 1996, 55, 358–363 CrossRef CAS.
- A. Pandey, C. R. Soccol, P. Nigam, V. T. Soccol, L. P. S. Vandenberghe and R. Mohan, Biotechnological potential of agroindustrial residues II cassava bagasse, Bioresour. Technol., 2000, 74, 81–87 CrossRef CAS.
- A. R. Domínguez-Bocanegra, J. A. Torres-Muñoz and R. A. López, Production of bioethanol from agroindustrial wastes, Fuel, 2014, 85–89 Search PubMed.
- H. B. Aditiya, W. T. Chong, T. M. I. Mahlia, A. H. Sebayang, M. A. Berawi and H. Nur, Second generation bioethanol potential from selected Malaysia’s biodiversity biomasses: A review, Waste Manag., 2015, 47, 46–61 CrossRef PubMed.
- H. B. Aditiya, K. P. Sing, M. Hanif and T. M. I. Mahlia, Effect of acid pretreatment on enzymatic hydrolysis in bioethanol production from rice straw, Int. J. Sci. Technol., 2015, 6, 3–10 Search PubMed.
- J. K. Saini, R. Agrawal, A. Satlewal, R. Saini, R. Gupta, A. Mathur and D. Tuli, Second generation bioethanol production at high gravity of pilot-scale pretreated wheat straw employing newly isolated thermotolerant yeast Kluyveromyces marxianus DBTIOC-35, RSC Adv., 2015, 5, 37485–37494 RSC.
- S. N. Hassan, Y. M. Sani, A. R. Abdul Aziz, N. M. N. Sulaiman and W. M. A. W. Daud, Biogasoline: An out-of-the-box solution to the food-for-fuel and land-use competitions, Energy Convers. Manage., 2015, 89, 349–367 CrossRef.
- E. Iye and P. Bilsborrow, Cellulosic ethanol production from agricultural residues in Nigeria, Energy Policy, 2013, 63, 207–214 CrossRef CAS.
- J. N. Putro, A. Kurniawan, F. E. Soetaredjo, S.-Y. Lin, Y.-H. Ju and S. Ismadji, Production of gamma-Valerolactone from sugarcane bagasse over TiO2-supported platinum and acid-activated bentonite as a co-catalyst, RSC Adv., 2015, 5, 41285–41299 RSC.
- R. C. Kuhad, R. Gupta, Y. P. Khasa, A. Singh and Y. H. P. Zhang, Bioethanol production from pentose sugars current status and future prospects, Renewable Sustainable Energy Rev., 2011, 15, 4950–4962 CrossRef CAS.
- B. C. Saha, Hemicellulose bioconversion, J. Ind. Microbiol. Biotechnol., 2003, 30, 279–291 CrossRef CAS PubMed.
- J. D. McMillan, Pretreatment of Lignocellulosic Biomass, ACS Publication, 1994, vol. 15, pp. 292–324 Search PubMed.
- N. Pereira Jr, M. A. Peixoto, G. Couto and L. M. M. Santa Anna, Biomass of lignocellulosic composition for fuel ethanol production within the context of biorefinery, Brazilian National Library, 2008, vol. 2, pp. 1–47 Search PubMed.
- M. Balat, Production of bioethanol from lignocellulosic materials via the biochemical pathway: a review, Energy Convers. Manage., 2011, 52, 858–875 CrossRef CAS.
- P. Alvira, E. Tomás-Pejó, M. Ballesteros and M. J. Negro, Pretreatment technologies for an efficient bioethanol production process based on enzymatic hydrolysis: a review, Bioresour. Technol., 2010, 101, 4851–4861 CrossRef CAS PubMed.
- S. Haghighi Mood, A. Hossein Golfeshan, M. Tabatabaei, G. Salehi Jouzani, G. H. Najafi, M. Gholami and M. Ardjmand, Lignocellulosic biomass to bioethanol, a comprehensive review with a focus on pretreatment, Renewable Sustainable Energy Rev., 2013, 27, 77–93 CrossRef CAS.
- A. Ebringerova, Z. Hromadkova and T. Heinze, Hemicellulose, Adv. Polym. Sci., 2005, 186, 1–67 CrossRef CAS.
- C. N. Hamelinck, G. V. Hooijdonk and A. P. C. Faaij, Ethanol from lignocellulosic biomass techno economic performance in short, middle and long term, Biomass Bioenergy, 2005, 28, 384–410 CrossRef CAS.
- C. Sanchez, Lignocellulosic residues: Biodegradation and bioconversion by fungi, Biotechnol. Adv., 2009, 27, 185–194 CrossRef CAS PubMed.
- D. Feldman, D. Banu, A. Natansohn and J. Wang, Structure properties relations of thermally cured epoxy lignin polyblends, J. Appl. Polym. Sci., 1991, 42, 1537–1550 CrossRef CAS.
- R. R. Singhania, J. K. Saini, R. Saini, M. Adsul, A. Mathur, R. Gupta and D. K. Tuli, Bioethanol production from wheat straw via enzymatic route employing Penicillium janthinellum cellulases, Bioresour. Technol., 2014, 169, 490–495 CrossRef CAS PubMed.
- A. Brandt, J. Grasvik, J. P. Hallett and T. Welton, Deconstruction of lignocellulosic biomass with ionic liquids, Green Chem., 2013, 15, 550–583 RSC.
- H. Kobayashi and A. Fukuoka, Synthesis and utilisation of sugar compounds derived from lignocellulosic biomass, Green Chem., 2013, 15, 1740–1763 RSC.
- P. Singh née’ Nigam, N. Gupta and A. Anthwal, Biotechnology for Agro-Industrial Residues Utilisation, Springer, 2009, vol. 2, pp. 13–33 Search PubMed.
- X. Li, T. H. Kim and N. P. Nghiem, Bioethanol production from corn stover using aqueous ammonia pretreatment and two phase simultaneous saccharification and fermentation (TPSSF), Bioresour.
Technol., 2010, 101, 5910–5916 CrossRef CAS PubMed.
- I. Ballesteros, M. Negro, J. Oliva, A. Cabañas, P. Manzanares and M. Ballesteros, Ethanol production from steam explosion pretreated wheat straw, Appl. Biochem. Biotechnol., 2006, 130, 496–508 CrossRef.
- R. Kumar, G. Mago, V. Balan and C. E. Wyman, Physical and chemical characterizations of corn stover and poplar solids resulting from leading pretreatment technologies, Bioresour. Technol., 2009, 100, 3948–3962 CrossRef CAS PubMed.
- C. Cara, E. Ruiz, M. Ballesteros, P. Manzanares, M. J. Negro and E. Castro, Production of fuel ethanol from steam explosion pretreated olive tree pruning, Fuel, 2008, 87, 692–700 CrossRef CAS.
- S. V. Vassilev, D. Baxter, L. K. Andersen and C. G. Vassileva, An overview of the chemical composition of biomass, Fuel, 2010, 89, 913–933 CrossRef CAS.
- J. Werther, M. Saenger, E. U. Hartge, T. Ogada and Z. Siagi, Combustion of agricultural residues, Prog. Energy Combust. Sci., 2000, 26, 1–27 CrossRef CAS.
- T. R. Miles, T. R. J. Miles, L. L. Baxter, R. W. Bryers, B. M. Jenkins and L. L. Oden, Alkali deposits found in biomass power plants: A preliminary investigation of their extent and nature, 1995, pp. 131–135, http://www.osti.gov/scitech//servlets/purl/251288-9ynoie/webviewable/ Search PubMed.
- J. M. O. Scurlock, D. C. Dayton and B. Hames, Bamboo: an overlooked biomass resource?, Biomass Bioenergy, 2000, 19, 229–244 CrossRef CAS.
- S. Kumar, R. Gupta, G. Kumar, D. Sahoo and R. C. Kuhad, Bioethanol production from Gracilaria verrucosa, a red alga, in a biorefinery approach, Bioresour. Technol., 2013, 135, 150–156 CrossRef CAS PubMed.
- R. P. John, G. S. Anisha, K. M. Nampoothiri and A. Pandey, Micro and macroalgal biomass a renewable source for bioethanol, Bioresour. Technol., 2011, 102, 186–193 CrossRef CAS PubMed.
- C. S. Goh and K. T. Lee, A visionary and conceptual macroalgae based third generation bioethanol (TGB) biorefinery in Sabah Malaysia as an underlay for renewable and sustainable development, Renewable Sustainable Energy Rev., 2010, 14, 842–848 CrossRef CAS.
- J. Adams, J. Gallagher and I. Donnison, Fermentation study on Saccharina latissima for bioethanol production considering variable pretreatments, J. Appl. Phycol., 2009, 21, 569–574 CrossRef CAS.
- S. G. Wi, H. J. Kim, S. A. Mahadevan, D.-J. Yang and H.-J. Bae, The potential value of the seaweed ceylon moss (Gelidium amansii) as an alternative bioenergy resource, Bioresour. Technol., 2009, 100, 6658–6660 CrossRef CAS PubMed.
- D. Hernández, B. Riaño, M. Coca and M. C. García-González, Saccharification of carbohydrates in microalgal biomass by physical, chemical and enzymaticpre-treatments as a previous step for bioethanol production, Chem. Eng. J., 2015, 262, 939–945 CrossRef.
- M. Daroch, S. Geng and G. Wang, Recent advances in liquid biofuel production from algal feedstocks, Appl. Energy, 2013, 102, 1371–1381 CrossRef.
- R. Harun and M. K. Danquah, Influence of acid pre-treatment on microalgal biomass for bioethanol production, Process Biochem., 2011, 46, 304–309 CrossRef CAS.
- M. A. Kassim, R. Potumarthi, A. Tanksale, S. C. Srivatsa and S. Bhattacharya, Enzymatic saccharification of dilute alkaline pretreated microalgal (Tetraselmis suecica) biomass for biobutanol production, International Journal of Food, Agriculture and Veterinary, 2014, 8, 967–972 Search PubMed.
- I. S. Tan and K. T. Lee, Enzymatic hydrolysis and fermentation of seaweed solid wastes for bioethanol production an optimization study, Energy, 2014, 78, 53–62 CrossRef CAS.
- G. P. B. Marquez, W. J. E. Santiañez, G. C. Trono Jr, M. N. E. Montaño, H. Araki, H. Takeuchi and T. Hasegawa, Seaweed biomass of the Philippines sustainable feedstock for biogas production, Renewable Sustainable Energy Rev., 2014, 38, 1056–1068 CrossRef.
- M. Borines, R. de Leon and M. McHenry, Bioethanol production from farming non-food macroalgae in Pacific island nations: chemical constituents, bioethanol yields, and prospective species in the Philippines, Renewable Sustainable Energy Rev., 2011, 15, 4432–4435 CrossRef CAS.
- Y. Peng, E. Xie, K. Zheng, M. Fredimoses, X. Yang, X. Zhou, Y. Wang, B. Yang, X. Lin and J. Liu, Nutritional and chemical composition and antiviral activity of cultivated seaweed Sargassum naozhouense Tseng et Lu, Mar. Drugs, 2012, 11, 20–32 CrossRef PubMed.
- P. Ratana-arporn and A. Chirapart, Nutritional evaluation of tropical green seaweeds Caulerpa lentillifera and Ulva reticulata, Kasetsart J., 2006, 40, 75–83 CAS.
- T. Burton, H. Lyons, Y. Lerat, M. Stanley and M. B. Rasmussen, A review of the potential of marine algae as a source of biofuel in Ireland, Sust Energ Ireland, 2009, pp. 1–92 Search PubMed.
- P. Fasahati, H. C. Woo and J. J. Liu, Industrial scale bioethanol production from brown algae effects of pretreatment processes on plant economics, Appl. Energy, 2015, 139, 175–187 CrossRef CAS.
- Z. Hossain, H. Kurihara and K. Takahashi, Biochemical composition and lipid compositional properties of the brown alga Sargassum horneri, Pak. J. Biol. Sci., 2003, 6, 1497–1500 CrossRef.
- M. G. Borines, R. L. de Leon and J. L. Cuello, Bioethanol production from the macroalgae Sargassum spp., Bioresour. Technol., 2013, 138, 22–29 CrossRef CAS PubMed.
- M. Zafar and S. Chowdhury, Water quality and biochemical components of Hydroclathrus clathratus in the tidal shore area of St. Martin’s Island Bangladesh, Int. J. Phycol. Phycochem., 2009, 5, 7–10 CAS.
- D. Chiaramonti, Improvement of crop plants for industrial end uses, Springer, 2007, pp. 209–251 Search PubMed.
- S. Chohnan, M. Nakane, M. H. Rahman, Y. Nitta, T. Yoshiura, H. Ohta and Y. Kurusu, Fuel ethanol production from sweet sorghum using repeated-batch fermentation, J. Biosci. Bioeng., 2011, 111, 433–436 CrossRef CAS PubMed.
- Y. Zhang, Thermochemical conversion of swine manure to produce fuel and reduce waste, Urbana, 1999, pp. 1–50 Search PubMed.
- J. Anjali and P. C. Satyendra, Bioethanol production in membrane bioreactor (MBR) system: a review, J. Environ. Res. Dev., 2014, 4, 387–394 Search PubMed.
- W. Tanthapanichakoon and S. W. Jian, Bioethanol production from cellulose and biomass derived syngas, Eng. J., 2012, 1, 1–4 CrossRef.
- P. F. H. Harmsen, W. Huijgen, L. Bermudez and R. Bakker, Literature review of physical and chemical pretreatment processes for lignocellulosic biomass, Food Biobased Res., 2010, 1, 1–49 Search PubMed.
- N. Eufrozina, Biotechnological production of bioethanol from different feedstocks a review, DOCT-US, 2009, vol. 1, pp. 1–6 Search PubMed.
- S. Mahalaxmi and C. Williford, Handbook of Climate Change Mitigation, Springer, 2012, vol. 26, pp. 965–999 Search PubMed.
- K. Rupar and M. Sanati, The release of terpenes during storage of biomass, Biomass Bioenergy, 2005, 28, 29–34 CrossRef CAS.
- C. Ricardo Soccol, V. Faraco, S. Karp, L. P. S. Vandenberghe, V. Thomaz-Soccol, A. Woiciechowski and A. Pandey, Biofuels: Alternative Feedstocks and Conversion Processes, Elsevier, 2011, pp. 101–122 Search PubMed.
- Y. Zheng, Z. Pan and R. Zhang, Overview of biomass pretreatment for cellulosic ethanol production, Int J Agri Biologi Eng., 2009, 2, 51–68 CAS.
- D. P. Maurya, A. Singla and S. Negi, An overview of key pretreatment processes for biological conversion of lignocellulosic biomass to bioethanol, Biotechnology, 2015, 1–13 CAS.
- J. S. Lee, B. Parameswaran, J. P. Lee and S. C. Park, Recent developments of key technologies on cellulosic ethanol production, J. Sci. Ind. Res., 2008, 67, 865 CAS.
- G. Brudecki, I. Cybulska and K. Rosentrater, Integration of extrusion and clean fractionation processes as a pre-treatment technology for prairie cordgrass, Bioresour. Technol., 2013, 135, 672–682 CrossRef CAS PubMed.
- C. Karunanithy and K. Muthukumarappan, Influence of extruder temperature and screw speed on pretreatment of corn stover while varying enzymes and their ratios, Appl. Biochem. Biotechnol., 2010, 162, 264–279 CrossRef CAS PubMed.
- C. Karunanithy and K. Muthukumarappan, Optimization of switchgrass and extruder parameters for enzymatic hydrolysis using response surface methodology, Ind. Crops Prod., 2011, 33, 188–199 CrossRef CAS.
- S. Zhang, D. R. Keshwani, Y. Xu and M. A. Hanna, Alkali combined extrusion pretreatment of corn stover to enhance enzyme saccharification, Ind. Crops Prod., 2012, 37, 352–357 CrossRef CAS.
- C. Karunanithy and K. Muthukumarappan, Effect of extruder parameters and moisture content of corn stover and big bluestem on sugar recovery from enzymatic hydrolysis, Bioengineering, 2009, 2, 91–113 CAS.
- S. Miller and R. Hester, Concentrated acid conversion of pine sawdust to sugars. Part I: Use of a twin-screw reactor for hydrolysis pretreatment, Chem. Eng. Commun., 2007, 194, 85–103 CrossRef CAS.
- W. H. Chen, B. L. Pen, C. T. Yu and W. S. Hwang, Pretreatment efficiency and structural characterization of rice straw by an integrated process of dilute-acid and steam explosion for bioethanol production, Bioresour. Technol., 2011, 102, 2916–2924 CrossRef CAS PubMed.
- P. B. Subhedar and P. R. Gogate, Intensification of enzymatic hydrolysis of lignocellulose using ultrasound for efficient bioethanol production: a review, Ind. Eng. Chem. Res., 2013, 52, 11816–11828 CrossRef CAS.
- S. Pilli, P. Bhunia, S. Yan, R. J. LeBlanc, R. D. Tyagi and R. Y. Surampalli, Ultrasonic pretreatment of sludge: A review, Ultrason. Sonochem., 2011, 18, 1–18 CrossRef CAS PubMed.
- X. Feng, H. Lei, J. Deng, Q. Yu and H. Li, Physical and chemical characteristics of waste activated sludge treated ultrasonically, Chem. Eng. Process., 2009, 48, 187–194 CrossRef CAS.
- A. Singh, D. Pant, N. E. Korres, A.-S. Nizami, S. Prasad and J. D. Murphy, Key issues in life cycle assessment of ethanol production from lignocellulosic biomass challenges and perspectives, Bioresour. Technol., 2010, 101, 5003–5012 CrossRef CAS PubMed.
- B. Savun, U. Neis, N. H. Ince and O. Yenigün, Pretreatment of Sewage Sludge by Low-frequency Ultrasound, J. Adv. Oxid. Technol., 2012, 15, 374–379 CAS.
- R. Kidak, A.-M. Wilhelm and H. Delmas, Effect of process parameters on the energy requirement in ultrasonical treatment of waste sludge, Chem. Eng. Process., 2009, 48, 1346–1352 CrossRef CAS.
- Y. Sudiyani, K. C. Sembiring and I. B. Adilina, Biomass Bioenergy, Springer, 2014, pp. 345–364 Search PubMed.
- M. J. Taherzadeh and K. Karimi, Pretreatment of lignocellulosic wastes to improve ethanol and biogas production a review, Int. J. Mol. Sci., 2008, 9, 1621–1651 CrossRef CAS PubMed.
- Y. Sun and J. Cheng, Hydrolysis of lignocellulosic materials for ethanol production: a review, Bioresour. Technol., 2002, 83, 1–11 CrossRef CAS PubMed.
- J. Tang, K. Chen, F. Huang, J. Xu and J. Li, Characterization of the pretreatment liquor of biomass from the perennial grass, Eulaliopsis binata, for the production of dissolving pulp, Bioresour. Technol., 2013, 129, 548–552 CrossRef CAS PubMed.
- T. Marzialetti, M. B. Valenzuela Olarte, C. Sievers, T. J. C. Hoskins, P. K. Agrawal and C. W. Jones, Dilute Acid Hydrolysis of Loblolly Pine: A Comprehensive Approach, Ind. Eng. Chem. Res., 2008, 47, 7131–7140 CrossRef CAS.
- L. Canilha, A. K. Chandel, T. A. Suzane dos Santos Milessi, F. A. Fernandes, W. L. D. C. Freitas, M. das Gra as Almeida Felipe and S. S. da Silva, Bioconversion of sugarcane biomass into ethanol: an overview about composition, pretreatment methods, detoxification of hydrolysates, enzymatic saccharification and ethanol fermentation, J. Biomed. Biotechnol., 2012, 1–15 CrossRef PubMed.
- E. C. Bensah and M. Mensah, Chemical pretreatment Methods for the production of cellulosic ethanol: technologies and innovations, Int. J. Chem. Eng., 2013, 2013, 1–21 CrossRef.
- V. Chang and M. Holtzapple, Fundamental factors affecting biomass enzymatic reactivity, Appl. Biochem. Biotechnol., 2000, 84–86, 5–37 CrossRef CAS PubMed.
- A. B. Bjerre, A. B. Olesen, T. Fernqvist, A. Plöger and A. S. Schmidt, Pretreatment of wheat straw using combined wet oxidation and alkaline hydrolysis resulting in convertible cellulose and hemicellulose, Biotechnol. Bioeng., 1996, 49, 568–577 CrossRef CAS PubMed.
- V. Chang, M. Nagwani, C.-H. Kim and M. Holtzapple, Oxidative lime pretreatment of high-lignin biomass, Appl. Biochem. Biotechnol., 2001, 94, 1–28 CrossRef CAS PubMed.
- X. F. Sun, R. C. Sun, P. Fowler and M. S. Baird, Isolation and characterisation of cellulose obtained by a two-stage treatment with organosolv and cyanamide activated hydrogen peroxide from wheat straw, Carbohydr. Polym., 2004, 55, 379–391 CrossRef CAS.
- J.-Y. Park, R. Shiroma, M. I. Al-Haq, Y. Zhang, M. Ike, Y. Arai-Sanoh, A. Ida, M. Kondo and K. Tokuyasu, A novel lime pretreatment for subsequent bioethanol production from rice straw – calcium capturing by carbonation (CaCCO) process, Bioresour. Technol., 2010, 101, 6805–6811 CrossRef CAS PubMed.
- N. Mosier, C. Wyman, B. Dale, R. Elander, Y. Y. Lee, M. Holtzapple and M. Ladisch, Features of promising technologies for pretreatment of lignocellulosic biomass, Bioresour. Technol., 2005, 96, 673–686 CrossRef CAS PubMed.
- A. M. Kura, An overview of bioethanol production from cassava feedstock in Nigeria, J. Biol. Sci. Bioconserv., 2014, 6, 59–72 Search PubMed.
- X. Zhao, K. Cheng and D. Liu, Organosolv pretreatment of lignocellulosic biomass for enzymatic hydrolysis, Appl. Microbiol. Biotechnol., 2009, 82, 815–827 CrossRef CAS PubMed.
- L. Mesa, E. González, C. Cara, M. González, E. Castro and S. I. Mussatto, The effect of organosolv pretreatment variables on enzymatic hydrolysis of sugarcane bagasse, Chem. Eng. J., 2011, 168, 1157–1162 CrossRef CAS.
- P. Kumar, D. M. Barrett, M. J. Delwiche and P. Stroeve, Methods for pretreatment of lignocellulosic biomass for efficient hydrolysis and biofuel production, Ind. Eng. Chem. Res., 2009, 48, 3713–3729 CrossRef CAS.
- M. P. García Aparicio, M. Ballesteros, P. Manzanares, I. Ballesteros, A. González and M. J. Negro, Xylanase contribution to the efficiency of cellulose enzymatic hydrolysis of barley straw, Appl. Biochem. Biotechnol., 2007, 137, 353–365 CrossRef PubMed.
- V. Chaturvedi and P. Verma, An overview of key pretreatment processes employed for bioconversion of lignocellulosic biomass into biofuels and value added products, Biotechnology, 2013, 3, 415–431 Search PubMed.
- M. T. García-Cubero, G. González-Benito, I. Indacoechea, M. Coca and S. Bolado, Effect of ozonolysis pretreatment on enzymatic digestibility of wheat and rye straw, Bioresour. Technol., 2009, 100, 1608–1613 CrossRef PubMed.
- M. Coca, M. Peña and G. González, Variables affecting efficiency of molasses fermentation wastewater ozonation, Chemosphere, 2005, 60, 1408–1415 CrossRef CAS PubMed.
- M. B. Roncero, A. L. Torres, J. F. Colom and T. Vidal, TCF bleaching of wheat straw pulp using ozone and xylanase. Part A: paper quality assessment, Bioresour. Technol., 2003, 87, 305–314 CrossRef CAS PubMed.
- A. A. Shatalov and H. Pereira, New perspectives for pulping and bleaching. Ozone-based TCF bleaching and organosolv pulps, Bioresour. Technol., 2008, 99, 472–478 CrossRef CAS PubMed.
- R. C. Remsing, R. P. Swatloski, R. D. Rogers and G. Moyna, Mechanism of cellulose dissolution in the ionic liquid 1-n-butyl-3-methylimidazolium chloride: a 13C and 35/37Cl NMR relaxation study on model systems, Chem. Commun., 2006, 1, 1271–1273 RSC.
- M. Francisco, A. van den Bruinhorst and M. C. Kroon, Low-Transition-Temperature Mixtures (LTTMs): A New Generation of Designer Solvents, Angew. Chem., Int. Ed., 2013, 52, 3074–3085 CrossRef CAS PubMed.
- A. M. da Costa Lopes, K. G. João, D. F. Rubik, E. Bogel-Łukasik, L. C. Duarte, J. Andreaus and R. Bogel-Łukasik, Pre-treatment of lignocellulosic biomass using ionic liquids: wheat straw fractionation, Bioresour. Technol., 2013, 142, 198–208 CrossRef CAS PubMed.
- J. Holm and U. Lassi, Ionic liquids in the pretreatment of lignocellulosic biomass, INTECH, 2011, vol. 1, pp. 1–17 Search PubMed.
- S. Zhang, J. Sun, X. Zhang, J. Xin, Q. Miao and J. Wang, Ionic liquid-based green processes for energy production, Chem. Soc. Rev., 2014, 43, 7838–7869 RSC.
- X. Q. Zhao, L. H. Zi, F. W. Bai, H. L. Lin, X. M. Hao, G. J. Yue and N. W. Ho, Biotechnology in China III: Biofuels and Bioenergy, Springer, 2012, pp. 25–51 Search PubMed.
- R. Kumar and C. E. Wyman, Does change in accessibility with conversion depend on both the substrate and pretreatment technology?, Bioresour. Technol., 2009, 100, 4193–4202 CrossRef CAS PubMed.
- J. Saini, R. Saini and L. Tewari, Lignocellulosic agriculture wastes as biomass feedstocks for second-generation bioethanol production: concepts and recent developments, Biotechnology, 2014, 1–17 Search PubMed.
- S. Decker, J. Sheehan, D. Dayton, J. Bozell, W. Adney, B. Hames, S. Thomas, R. Bain, S. Czernik, M. Zhang and M. Himmel, Industrial Chemical Biotechnology, Springer, 2007, vol. 33, pp. 1449–1548 Search PubMed.
- G. Emtiazi, N. Naghavi and A. Bordbar, Biodegradation of lignocellulosic waste by Aspergillus terreus, Biodegradation, 2001, 12, 257–261 CrossRef.
- J. Pérez, J. Munoz-Dorado, T. de la Rubia and J. Martinez, Biodegradation and biological treatments of cellulose, hemicellulose and lignin an overview, Int. Microbiol., 2002, 5, 53–63 CrossRef PubMed.
- F. A. Keller, J. E. Hamilton and Q. A. Nguyen, Microbial pretreatment of biomass: Potential for reducing severity of thermochemical biomass pretreatment, Appl. Biochem. Biotechnol., 2003, 105, 27–41 CrossRef PubMed.
- E. G. Shide, P. A. Wuyep and A. Nok, Studies on the degradation of wood sawdust by Lentinussquarrosulus (Mont.) singer, Afr. J. Biotechnol., 2004, 3, 395–398 CrossRef CAS.
- P. C. Badger, Ethanol from cellulose: a general review, Trends in New Crops and New Uses, 2002, 17–21 Search PubMed.
- H. O. Berg, Comparison of conversion pathways for lignocellulosic biomass to biofuel in mid-Norway, Energy Environ. Eng., 2013, 1–155 Search PubMed.
- S. N. Naik, V. V. Goud, P. K. Rout and A. K. Dalai, Production of first and second generation biofuels: a comprehensive review, Renewable Sustainable Energy Rev., 2010, 14, 578–597 CrossRef CAS.
- J. Peng, Z. Fan and G. Chen, Thermochemical conversion technology on lignocellulosic biomass to liquid fuel a critical review, Power Energy Eng., 2011, 1–6 Search PubMed.
- Z. L. Liu, P. J. Slininger and S. W. Gorsich, Enhanced biotransformation of furfural and hydroxymethylfurfural by newly developed ethanologenic yeast strains, Biotechnol. Fuel Chem., 2005, 451–460 CAS.
- H. Chen and W. Qiu, Key technologies for bioethanol production from lignocellulose, Biotechnol.
Adv., 2010, 28, 556–562 CrossRef CAS PubMed.
- H. A. Ruiz, R. M. Rodríguez-Jasso, B. D. Fernandes, A. A. Vicente and J. A. Teixeira, Hydrothermal processing as an alternative for upgrading agriculture residues and marine biomass according to the biorefinery concept a review, Renewable Sustainable Energy Rev., 2013, 21, 35–51 CrossRef CAS.
- F. Talebnia, D. Karakashev and I. Angelidaki, Production of bioethanol from wheat straw an overview on pretreatment, hydrolysis and fermentation, Bioresour. Technol., 2010, 101, 4744–4753 CrossRef CAS PubMed.
- M. Laser, D. Schulman, S. G. Allen, J. Lichwa, M. J. Antal and L. R. Lynd, A comparison of liquid hot water and steam pretreatments of sugar cane bagasse for bioconversion to ethanol, Bioresour. Technol., 2002, 81, 33–44 CrossRef CAS PubMed.
- A. W. Bhutto, K. Qureshi, K. Harijan, G. Zahedi and A. Bahadori, Strategies for the consolidation of biologically mediated events in the conversion of pre-treated lignocellulose into ethanol, RSC Adv., 2014, 4, 3392–3412 RSC.
- J. A. Pérez, I. Ballesteros, M. Ballesteros, F. Sáez, M. J. Negro and P. Manzanares, Optimizing Liquid Hot Water pretreatment conditions to enhance sugar recovery from wheat straw for fuel-ethanol production, Fuel, 2008, 87, 3640–3647 CrossRef.
- W. Jiang, S. Chang, H. Li, P. Oleskowicz-Popiel and J. Xu, Liquid hot water pretreatment on different parts of cotton stalk to facilitate ethanol production, Bioresour. Technol., 2015, 176, 175–180 CrossRef CAS PubMed.
- S. Imman, J. Arnthong, V. Burapatana, V. Champreda and N. Laosiripojana, Influence of alkaline catalyst addition on compressed liquid hot water pretreatment of rice straw, Chem. Eng. J., 2015, 278, 85–91 CrossRef CAS.
- H. Alizadeh, F. Teymouri, T. I. Gilbert and B. E. Dale, Pretreatment of switchgrass by ammonia fiber explosion (AFEX), Appl. Biochem. Biotechnol., 2005, 124, 1133–1141 CrossRef.
- B. Bryan, C. Rogers, J. Mingjie, B. Venkatesh and D. Bruce, Evaluation of ammonia fibre expansion (AFEX) pretreatment for enzymatic hydrolysis of switchgrass harvested in different seasons and locations, Biotechnol. Biofuels, 2010, 3, 1–11 CrossRef PubMed.
- G. Brodeur, E. Yau, K. Badal, J. Collier, K. Ramachandran and S. Ramakrishnan, Chemical and physicochemical pretreatment of lignocellulosic biomass: a review, Enzyme Res., 2011, 1–18 CrossRef PubMed.
- R. Munter, Advanced oxidation processes current status and prospects, Proc. Est. Acad. Sci., Chem., 2001, 50, 59–80 CAS.
- E. Arvaniti, A. B. Bjerre and J. E. Schmidt, Wet oxidation pretreatment of rape straw for ethanol production, Biomass Bioenergy, 2012, 39, 94–105 CrossRef.
- C. Chen, D. Boldor, G. Aita and M. Walker, Ethanol productionfrom sorghum by a microwave-assisted dilute ammonia pretreatment, Bioresour. Technol., 2012, 110, 190–197 CrossRef CAS PubMed.
- D. R. Keshwani and J. J. Cheng, Microwave based alkali pretreatment of switchgrass and coastal bermudagrass for bioethanol production, Biotechnol. Prog., 2010, 26, 644–652 CrossRef CAS PubMed.
- N. Rahnama, S. Mamat, U. K. M. Shah, F. H. Ling, N. A. A. Rahman and A. B. Ariff, Effect of alkali pretreatment of rice straw on cellulase and xylanase production by local Trichoderma harzianum SNRS3 under solid state fermentation, Bioresour. Technol., 2013, 8, 2881–2896 Search PubMed.
- D. Jones, T. Lelyveld, S. Mavrofidis, S. Kingman and N. Miles, Microwave heating applications in environmental engineering a review, Resour., Conserv. Recycl., 2002, 34, 75–90 CrossRef.
- C. Bramsiepe, S. Sievers, T. Seifert, G. Stefanidis, D. G. Vlachos, H. Schnitzer, B. Muster, C. Brunner, J. Sanders and M. Bruins, Low-cost small scale processing technologies for production applications in various environments Mass produced factories, Chem. Eng. Process., 2012, 51, 32–52 CrossRef CAS.
- S. Chittibabu, K. Rajendran, M. Santhanmuthu and M. Saseetharan, Optimization of microwave assisted alkali pretreatment and enzymatic hydrolysis of Banana pseudostem for bioethanol production, Int. J. Environ. Sci. Technol., 2011, 67–71 Search PubMed.
- S. Nikolić, L. Mojović, M. Rakin, D. Pejin and D. Savić, A microwave-assisted liquefaction as a pretreatment for the bioethanol production by the simultaneous saccharification and fermentation of corn meal, Chem. Ind. Chem. Eng. Q., 2008, 14, 231–234 CrossRef.
- S. Spatari, D. M. Bagley and H. L. MacLean, Life cycle evaluation of emerging lignocellulosic ethanol conversion technologies, Bioresour. Technol., 2010, 101, 654–667 CrossRef CAS PubMed.
- K. H. Kim and J. Hong, Supercritical CO2 pretreatment of lignocellulose enhances enzymatic cellulose hydrolysis, Bioresour. Technol., 2001, 77, 139–144 CrossRef CAS PubMed.
- A. T. Hendricks and G. Zeeman, Pretreatments to enhance the digestibility of lignocellulosic biomass, Bioresour. Technol., 2009, 100, 10–18 CrossRef PubMed.
- V. B. Agbor, N. Cicek, R. Sparling, A. Berlin and D. B. Levin, Biomass pretreatment: Fundamentals toward application, Biotechnol. Adv., 2011, 29, 675–685 CrossRef CAS PubMed.
- Y.-L. Cha, J. Yang, J.-W. Ahn, Y.-H. Moon, Y.-M. Yoon, G.-D. Yu, G. An and I.-H. Choi, The optimized CO2-added ammonia explosion pretreatment for bioethanol production from rice straw, Bioprocess Biosyst. Eng., 2014, 37, 1907–1915 CrossRef CAS PubMed.
- T. Gu, Green Biomass Pretreatment for Biofuels Production, Springer, 2013, vol. 5, pp. 107–125 Search PubMed.
- D. S. Zhang, Q. Yang, J. Y. Zhu and X. J. Pan, Sulfite (SPORL) pretreatment of switchgrass for enzymatic saccharification, Bioresour. Technol., 2013, 129, 127–134 CrossRef CAS PubMed.
- A. Graf and T. Koehler, Oregon cellulose-ethanol study: an evaluation of the potential for ethanol production in Oregon using cellulose based feedstocks, 2000, pp. 1–36, www.oregon.gov/energy/P-I/Biomass/docs/OCES/OCES.PDF Search PubMed.
- Y. Morikawa, X. Zhao and D. Liu, Biological co-production of ethanol and biodiesel from wheat straw: a case of dilute acid pretreatment, RSC Adv., 2014, 4, 37878–37888 RSC.
- R. Rao, C. Jyothi, R. Prakasham, P. Sarma and L. Rao, Xylitol production from corn fiber and sugarcane bagasse hydrolysates by Candida tropicalis, Bioresour. Technol., 2006, 97, 1974–1978 CrossRef CAS PubMed.
- A. A. Refaat, Comprehensive Renewable Energy, Elsevier, 2012, pp. 217–261 Search PubMed.
- J. Iranmahboob, F. Nadim and S. Monemi, Optimizing acid-hydrolysis: A critical step for production of ethanol from mixed wood c, Biomass Bioenergy, 2002, 22, 401–404 CrossRef CAS.
- N. Mosier, C. Ladisch and M. Ladisch, Characterization of acid catalytic domains for cellulose hydrolysis and glucose degradation, Biotechnol. Bioeng., 2002, 79, 610–618 CrossRef CAS PubMed.
- Y. Sun, Enzymatic hydrolysis of rye straw and Bermuda grass for ethanol production, Biological and Agricultural Engineering, 2002, 1–140 Search PubMed.
- X. Pan, C. Arato, N. Gilkes, D. Gregg, W. Mabee and K. Pye, Biorefining of softwoods using ethanol organosolv pulping: Preliminary evaluation of process streams for manufacture of fuel grade ethanol and co-products, Biotechnol. Bioeng., 2005, 90, 473–481 CrossRef CAS PubMed.
- A. Gusakov, T. Salanovich, A. Antonov, B. Ustinov, O. Okunev and R. Burlingame, Design of highly efficient cellulase mixtures for enzymatic hydrolysis of cellulose, Biotechnol. Bioeng., 2007, 97, 1028–1038 CrossRef CAS PubMed.
- Y. Y. Tye, K. T. Lee, W. N. W. Abdullah and C. P. Leh, Potential of Ceiba pentandra (L.) Gaertn. (kapok fiber) as a resource for second generation bioethanol: Effect of various simple pretreatment methods on sugar production, Bioresour. Technol., 2012, 116, 536–539 CrossRef CAS PubMed.
- Q. Kang, L. Appels, T. Tan and R. Dewil, Bioethanol from lignocellulosic biomass current findings determine research priorities, Sci. World J., 2014, 1–14 Search PubMed.
- C. Wyman and B. Yang, Cellulosic biomass could help meet California’s transportation fuel needs, Calif. Agric., 2009, 63, 185–190 CrossRef.
- M. Abbi, R. C. Kuhad and A. Singh, Bioconversion of pentose sugars to ethanol by free and immobilized cells of Candida shehatae (NCL-3501): fermentation behaviour, Process Biochem., 1996, 31, 555–560 CrossRef CAS.
- C. Martin, H. B. Klinke and A. B. Thomsen, Wet oxidation as a pretreatment method for enhancing the enzymatic convertibility of sugarcane bagasse, Enzyme Microb. Technol., 2007, 40, 426–432 CrossRef CAS.
- L. Korzen, I. N. Pulidindi, A. Israel, A. Abelson and A. Gedanken, Single step production of bioethanol from the seaweed Ulva rigida using sonication, RSC Adv., 2015, 5, 16223–16229 RSC.
- K. Olofsson, M. Bertilsson and G. Lidén, A short review on SSF an interesting process option for ethanol production from lignocellulosic feedstocks, Biotechnol. Biofuels, 2008, 1, 1–14 CrossRef PubMed.
- A. Wingren, M. Galbe and G. Zacchi, Techno economic evaluation of producing ethanol from softwood comparison of SSF and SHF and identification of bottlenecks, Biotechnol. Prog., 2003, 19, 1109–1117 CrossRef CAS PubMed.
- Z. H. Liu, L. Qin, J. Q. Zhu, B. Z. Li and Y. J. Yuan, Simultaneous saccharification and fermentation of steam exploded corn stover at high glucan loading and high temperature, Biotechnol. Biofuels, 2014, 7, 167 CrossRef PubMed.
- M. Balat and H. Balat, Recent trends in global production and utilization of bio-ethanol fuel, Appl. Energy, 2009, 86, 2273–2282 CrossRef CAS.
- B. C. Saha and M. A. Cotta, Ethanol production from alkali peroxide pretreated enzymatically saccharified wheat straw, Biotechnol. Prog., 2006, 22, 449–453 CrossRef CAS PubMed.
- P. Karagöz, I. V. Rocha, M. Özkan and I. Angelidaki, Alkali peroxide pretreatment of rapeseed straw for enhancing bioethanol production by same vessel saccharification and co-fermentation, Bioresour. Technol., 2012, 104, 349–357 CrossRef PubMed.
- J. Baeyens, Q. Kang, L. Appels, R. Dewil, Y. Lv and T. Tan, Challenges and opportunities in improving the production of bioethanol, Prog. Energy Combust. Sci., 2015, 47, 60–88 CrossRef.
- C. A. Cardona and Ó. J. Sánchez, Fuel ethanol production: process design trends and integration opportunities, Bioresour. Technol., 2007, 98, 2415–2457 CrossRef CAS PubMed.
- A. K. Chandel, E. Chan, R. Rudravaram, M. L. Narasu, L. V. Rao and P. Ravindra, Economics and environmental impact of bioethanol production technologies: an appraisal, Biotechnol. Mol. Biol. Rev., 2007, 2, 14–32 Search PubMed.
- A. Goel, L. Wati and C. Hisar, Ethanol production from lignocellulosic materials, International Journal of Innovations in Bio-Sciences, 2013, 3, 111–114 Search PubMed.
- S. Onuki, Bioethanol: Industrial production process and recent studies, J. Chem. Technol. Biotechnol., 2006, 1–12 Search PubMed.
- R. Wooley, M. Ruth, J. Sheehan, K. Ibsen, H. Majdeski and A. Galvez, Lignocellulosic biomass to ethanol process design and economics utilizing co-current dilute acid prehydrolysis and enzymatic hydrolysis current and futuristic scenarios, DTIC, 1999 Search PubMed.
- K. Gabriel and M. El Halwagi, Modeling and optimization of a bioethanol production facility, Clean Technol. Environ. Policy, 2013, 15, 931–944 CrossRef.
- A. McAloon, F. Taylor, W. Yee, K. Ibsen and R. Wooley, Determining the cost of producing ethanol from corn starch and lignocellulosic feedstocks, National Renew Energ Lab, 2000, pp. 1–44 Search PubMed.
- P. W. Madson and D. A. Monceaux, Fuel ethanol production, The Alcohol Textbook, 1995, pp. 227–238 Search PubMed.
- A. A. Kiss, J. David and P. Suszwalak, Enhanced bioethanol dehydration by extractive and azeotropic distillation in dividing-wall columns, Sep. Purif. Technol., 2012, 86, 70–78 CrossRef CAS.
- G. P. D. Silva, E. F. D. Araújo, D. O. Silva and W. V. Guimarães, Ethanolic fermentation of sucrose, sugarcane juice and molasses by Escherichia coli strain KO11 and Klebsiella oxytoca strain P2, Braz. J. Microbiol., 2005, 36, 395–404 CrossRef.
- C. V. Ratnavathi, K. Suresh, B. S. V. Kumar, M. Pallavi, V. V. Komala and N. Seetharama, Study on genotypic variation for ethanol production from sweet sorghum juice, Biomass Bioenergy, 2010, 34, 947–952 CrossRef CAS.
- J. C. Ogbonna, H. Mashima and H. Tanaka, Scale up of fuel ethanol production from sugar beet juice using loofa sponge immobilized bioreactor, Bioresour. Technol., 2001, 76, 1–8 CrossRef CAS PubMed.
- S. S. Dhaliwal, H. S. Oberoi, S. K. Sandhu, D. Nanda, D. Kumar and S. K. Uppal, Enhanced ethanol production from sugarcane juice by galactose adaptation of a newly isolated thermotolerant strain of Pichia kudriavzevii, Bioresour. Technol., 2011, 102, 5968–5975 CrossRef CAS PubMed.
- S. Limtong, C. Sringiew and W. Yongmanitchai, Production of fuel ethanol at high temperature from sugar cane juice by a newly isolated Kluyveromyces marxianus, Bioresour. Technol., 2007, 98, 3367–3374 CrossRef CAS PubMed.
- J. Kawa-Rygielska, W. Pietrzak, P. Regiec and P. Stencel, Utilization of concentrate after membrane filtration of sugar beet thin juice for ethanol production, Bioresour. Technol., 2013, 133, 134–141 CrossRef CAS PubMed.
- W. W. Fish, B. D. Bruton and V. M. Russo, Watermelon juice: a promising feedstock supplement, diluent and nitrogen supplement for ethanol biofuel production, Biotechnol. Biofuels, 2009, 2, 1–9 CrossRef PubMed.
- S. R. A. Khalil, A. A. Abdelhafez and E. A. M. Amer, Evaluation of bioethanol production from juice and bagasse of some sweet sorghum varieties, Ann. Agric. Sci., 2015, 1–8 CAS.
- B. Buaban, H. Inoue, S. Yano, S. Tanapongpipat, V. Ruanglek, V. Champreda, R. Pichyangkura, S. Rengpipat and L. Eurwilaichitr, Bioethanol production from ball milled bagasse using an on-site produced fungal enzyme cocktail and xylose-fermenting Pichia stipitis, J. Biosci. Bioeng., 2010, 110, 18–25 CrossRef CAS PubMed.
- C. M. Takahashi, K. G. de Carvalho Lima, D. F. Takahashi and F. Alterthum, Fermentation of sugar cane bagasse hemicellulosic hydrolysate and sugar mixtures to ethanol by recombinant Escherichia coli KO11, World J. Microbiol. Biotechnol., 2000, 16, 829–834 CrossRef CAS.
- J. Nigam, Ethanol production from wheat straw hemicellulose hydrolysate by Pichia stipitis, J. Biotechnol., 2001, 87, 17–27 CrossRef CAS PubMed.
- M. Abbi, R. Kuhad and A. Singh, Fermentation of xylose and rice straw hydrolysate to ethanol by Candida shehatae NCL-3501, J. Ind. Microbiol. Biotechnol., 1996, 17, 20–23 CrossRef CAS PubMed.
- M. Moniruzzaman, Alcohol fermentation of enzymatic hydrolysate of exploded rice straw by Pichia stipitis, World J. Microbiol. Biotechnol., 1995, 11, 646–648 CrossRef CAS PubMed.
- M. Chen, J. Zhao and L. Xia, Enzymatic hydrolysis of maize straw polysaccharides for the production of reducing sugars, Carbohydr. Polym., 2008, 71, 411–415 CrossRef CAS.
- T. Dragos, D. Alexandru and B. Marin, Experimental investigation on emissions and performance of a spark ignition engine fueled with gasoline ethanol blends, Carbon, 2014, 4814, 285–288 Search PubMed.
- Renewable Fuels Association, Fuel ethanol industry guidelines, specification and procedures, 2010, pp. 1–26, http://www.ethanolrfa.3cdn.net/4eea401b7975120b97_nrm6bhv0i.pdf Search PubMed.
- L. M. Robert and P. Richard, Advanced petroleum based fuels program and renewable diesel program, National Renew Energ Lab, 2001, pp. 1–149 Search PubMed.
- R. E. Reynolds, Fuel specifications and fuel property issues and their potential impact on the use of ethanol as a transportation fuel, Downstream Alternatives Inc., 2002, pp. 1–100 Search PubMed.
- U. Larsen, T. Johansen and J. Schramm, Ethanol as a future fuel for road transportation, 2009, pp. 1–115, http://www.iea-amf.org/app/webroot/files/file/…/AMF_Annex_35-1.pdf Search PubMed.
- B. M. Masum, H. H. Masjuki, M. A. Kalam, I. M. Rizwanul Fattah, S. M. Palash and M. J. Abedin, Effect of ethanol gasoline blend on NOx emission in SI engine, Renewable Sustainable Energy Rev., 2013, 24, 209–222 CrossRef CAS.
- S. González-García, M. T. Moreira and G. Feijoo, Comparative environmental performance of lignocellulosic ethanol from different feedstocks, Renewable Sustainable Energy Rev., 2010, 14, 2077–2085 CrossRef.
- F. T. Ademiluyi and H. D. Mepba, Yield and properties of ethanol biofuel produced from different whole cassava flours, ISRN Biotechnol., 2013, 1–7 CrossRef PubMed.
- K. J. Han, W. D. Pitman, M. Kim, D. F. Day, M. W. Alison, M. E. McCormick and G. Aita, Ethanol production potential of sweet sorghum assessed using forage fiber analysis procedures, GCB Bioenergy, 2013, 5, 358–366 CrossRef CAS.
- A. Küüt, K. Ritslaid and J. Olt, Study of potential uses for farmstead ethanol as motor fuel, Agricultural and Biosystems Engineering, 2011, 1, 125–134 Search PubMed.
- International standard organization, Ethanol for industrial use methods of test part 2 detection of alkalinity or determination of acidity to phenolphthalein, 2001, pp. 1–9, https://law.resource.org/pub/eac/ibr/eas.216.2.2001.pdf Search PubMed.
- R. G. Bates, G. D. Pinching and E. R. Smith, pH standards of high acidity and high alkalinity and the practical scale of pH, J. Res. Natl. Bur. Stand., 1990, 45, 418–429 CrossRef.
- L. Yue, Analysis of acidity in oil based matrices by infrared spectroscopy, Food Sci. Agric. Chem., 2009, 1–84 Search PubMed.
- C. Wyman, Handbook on bioethanol production and utilization, Appl Energ Technol., 1996, pp. 1–444 Search PubMed.
- M. Lapuerta, O. Armas and R. Garcia-Contreras, Stability of diesel bioethanol blends for use in diesel engines, Fuel, 2007, 86, 1351–1357 CrossRef CAS.
- R. Dominik and J. Rainer, Biofuel technology handbook, Renewable Energy, 2007, 1–149 Search PubMed.
- R. J. C. Brown, A. C. Keates and P. J. Brewer, Sensitivities of a standard test Method for the determination of the pHe of bioethanol and suggestions for improvement, Sensors, 2010, 10, 9982–9993 CrossRef CAS PubMed.
- P. Spitzer, P. Fisicaro, S. Seitz and R. Champion, pH and electrolytic conductivity as parameters to characterize bioethanol, Accredit. Qual. Assur., 2009, 14, 671–676 CrossRef CAS.
- A. Cernat and Ş. Velescu Elescu, Bioethanol available alternative fuel for SI engine, Termotehnica, 2013, 2, 110–118 Search PubMed.
- The Brazilian Development Bank, Sugarcane based bioethanol energy for sustainable development, Food Agri Organization, 2008, pp. 53–62 Search PubMed.
- E. Sadeghinezhad, S. N. Kazi, A. Badarudin, H. Togun, M. N. Zubir, C. S. Oon and S. Gharehkhani, Sustainability and environmental impact of ethanol as a biofuel, Rev. Chem. Eng., 2014, 30, 51–72 CAS.
- B. A. Adelekan, Investigation of ethanol productivity of cassava crop as a sustainable source of biofuel in tropical countries, Afr. J. Biotechnol., 2013, 9, 5643–5650 Search PubMed.
- A. Matuszewska, M. Odziemkowska and J. Czarnocka, Properties of bioethanol diesel oil mixtures, Materials Process Energy, 2013, 1, 352–359 Search PubMed.
- S. Prasad, A. Singh, N. Jain and H. C. Joshi, Ethanol production from sweet sorghum syrup for utilization as automotive fuel in India, Energy Fuels, 2007, 21, 2415–2420 CrossRef CAS.
- A. N. Ghanim, Bioethanol production from Iraqi date palm resources, J. Babylon Univ. Eng. Sci., 2013, 21, 239–248 Search PubMed.
- A. F. Kheiralla, E. A. Mohamed, Y. H. Mathani, M. A. Hussen and I. O. Hind, Effect of ethanol gasoline blends on fuel properties characteristics of spark ignition engines, Khartoum Univ. Eng. J., 2011, 1, 22–28 Search PubMed.
- M. Ghazikhani, M. Hatami, B. Safari and D. D. Ganji, Experimental investigation of performance improving and emissions reducing in a two stroke SI engine by using ethanol additives, Propulsion and Power Research, 2013, 2, 276–283 CrossRef.
- G. Najafi, B. Ghobadian, T. Tavakoli, D. R. Buttsworth, T. F. Yusaf and M. Faizollahnejad, Performance and exhaust emissions of a gasoline engine with ethanol blended gasoline fuels using artificial neural network, Appl. Energy, 2009, 86, 630–639 CrossRef CAS.
- I. Schifter, L. Diaz, R. Rodriguez, J. P. Gómez and U. Gonzalez, Combustion and emissions behavior for ethanol gasoline blends in a single cylinder engine, Fuel, 2011, 90, 3586–3592 CrossRef CAS.
- K. B. Siddegowda and J. Venkatesh, Performance and emission characteristics of MPFI engine by using gasoline ethanol blends, International Journal of Innovative Research in Science, 2013, 2, 4891–4897 Search PubMed.
- D. Tutunea, A. Dima and M. Bica, Experimental investigation on emissions and performance of a spark ignition engine fueled with gasoline ethanol blends, Carbon, 2014, 1, 285–288 Search PubMed.
- S. Gomasta and S. Mahla, An experimental investigation of ethanol blended diesel fuel on engine performance and emission of a diesel engine, Int. J. Emerging Technol., 2012, 74–79 Search PubMed.
- E. Sadeghinezhad, S. N. Kazi, F. Sadeghinejad, A. Badarudin, M. Mehrali, R. Sadri and M. Reza Safaei, A comprehensive literature review of bio-fuel performance in internal combustion engine and relevant costs involvement, Renewable Sustainable Energy Rev., 2014, 30, 29–44 CrossRef CAS.
- M. Koç, Y. Sekmen, T. Topgül and H. S. Yücesu, The effects of ethanol unleaded gasoline blends on engine performance and exhaust emissions in a spark ignition engine, Renewable Energy, 2009, 34, 2101–2106 CrossRef.
- C. Liang, C. Ji, B. Gao, X. Liu and Y. Zhu, Investigation on the performance of a spark ignited ethanol engine with DME enrichment, Energy Convers. Manage., 2012, 58, 19–25 CrossRef CAS.
- C. A. Srinivasan and C. Saravanan, Study of combustion characteristics of an SI engine fuelled with ethanol and oxygenated fuel additives, Journal of Sustainable Energy & Environment, 2010, 1, 85–91 Search PubMed.
|
This journal is © The Royal Society of Chemistry 2016 |