DOI:
10.1039/C5RA24922H
(Paper)
RSC Adv., 2016,
6, 12616-12626
Modifying influences of micro crystalline and nanocellulose on the gelling characteristics of poly(methacrylic acid-co-2-hydroxyethylmethacrylate)
Received
24th November 2015
, Accepted 16th January 2016
First published on 19th January 2016
Abstract
Cellulose dispersions were incorporated in the methacrylic acid (MAc)–2-hydroxyethyl methacrylate (HEMA) copolymer hydrogel matrix formed in situ and the effects of such incorporation on various properties of the resulting hydrogel were investigated by means of swelling–deswelling study, morphological study and rheological characterization. The absorption capacity of the hydrogels decreases on increasing HEMA content in the copolymer network. The introduction of cellulose, particularly nanocellulose in the MAc–HEMA co-polymer network enhances remarkably the swelling efficiency of the hydrogels. Such incorporation also affects the rheological and morphological characteristics of the hydrogels. The deposition or adsorption of cellulose in and around the pores of the polymer matrix is clearly revealed by SEM images. The cellulose based hydrogels are found to be efficient particularly with respect to the removal of cationic dyes and heavy metal ions (Cu2+ and Fe3+) from aqueous solution.
1. Introduction
Hydrogels are crosslinked polymeric materials or self-assembled small molecules that have the ability to entrap water molecules in the polymer network.1–4 The crosslinking may be physical or chemical in nature. In the case of physical crosslinking the polymer chains are held by entanglements or by some physical interactions, like hydrogen bonding, ionic interactions etc. Covalent bond formation occurs in chemical crosslinking processes.2,5–7 On crosslinking, the material shows viscoelastic or sometimes elastic nature when compared to the Newtonian behaviour of the aqueous solution of ionic polymers in general.1
Polymethacrylic acid (MAc) cannot be crosslinked to form a gel that can entrap water in the crosslinked polymer network, though it has polar –COOH groups in the chain. On the other hand crosslinked poly(2-hydroxyethyl methacrylate) (HEMA) is a well-known gelling material but it has the drawback of low water absorptivity. In combination, the crosslinked poly(MAc-HEMA) is a very well-known hydrogel material having excellent water absorptivity and pH-dependent swelling behaviour as well. There are few studies reporting the MAc–HEMA based polymer hydrogels.8–11
Daniel Klinger et al. prepared stimuli responsive hydrogels based on HEMA, MAc and a photodegradable crosslinker by emulsion polymerization method. Swelling of the gel depends on the pH and UV light irradiation. Water absorption of the gel increases with the pH of the medium while it decreases on irradiation with UV light.9
Cellulose is the most abundant natural polymer. It is now widely used in the synthesis of super absorbent hydrogels due to its biocompatible and biodegradable nature, so that the hydrogels may find applications in biomedical fields.12–17 Cellulose–PVA based hydrogels were prepared by Chunyu Chang et al.12 Water absorption of the porous hydrogel was found to increase with the cellulose incorporation in their work. Niladri Roy et al. prepared NVP–carboxy methyl cellulose (CMC) based hydrogel and they reported enhancement in swelling and modification of rheological properties of the gel in relation to CMC content.14 Acrylic acid–acrylamide-2-acrylamido-2-methylpropane sulphonic acid based terpolymer was grafted to sodium carboxymethyl cellulose to form super absorbent hydrogel in presence of montmorrillonite (MMT) clay by Bao et al. The hydrogels were observed to have the typical porous structure with undulant and coarse surface. Swelling of the gels however was found to depend on the pH and salt concentration in the swelling medium.15
Review of works studied so far, indicates almost no reports on the effect of incorporation of cellulose in the crosslinked network of poly(MAc-HEMA). Therefore the novelty of the present work lies in the detailed investigation of the hydrogel properties after incorporation of microcrystalline cellulose and nanocellulose separately in the crosslinked polymer network of MAc-co-HEMA. Studies were made on the spectroscopic properties, swelling efficiency, rheological and morphological analysis. A comparative study also was made on the cationic dye and heavy metal ion separation of the three series of hydrogels.
2. Materials and methods
2.1. Materials used
Methacrylic acid (MAc), 2-hydroxyethyl methacrylate (HEMA), N,N′-methylenebisacrylamide (MBA), methyl violet and fuchsine basic dye brought from Loba Chemie, India, were used without any purification.
Sodium bisulphite, ammonium persulfate (APS) and buffer capsules (pH 4 and 9.2) were supplied by Merck, Darmstadt, Germany.
Ethyleneglycol dimethacrylate was received from Aldrich, USA, and used as such.
Microcrystalline cellulose (MCC) was supplied by Loba Chemie, Mumbai, India.
Nanocellulose (NC) was synthesized from sugarcane bagasse by following the method reported by Mandal et al.18 The NC was in the form of stable suspension of which more than 90% of the volume fraction of particle was in the nano-metric range.
2.2. Method of hydrogel preparation
Measured amounts of methacrylic acid and HEMA were taken in a 100 mL conical flask. MBA and the constituents of redox initiator, like NaHSO3 (SBS) and (NH4)2S2O8 (APS) were dissolved in 10 mL water each in separate test tubes and added to the said conical flask containing the monomers. MBA, a potential crosslinker for both poly(HEMA) and poly(MAc) was added to the extent of 1 mol% (with respect to the total moles of HEMA and MAc taken). 40 mL of water was subsequently added to the flask to make the reaction mixture concentration, 5 mol L−1. Details of the chemicals used are given in Table 1. Purified nitrogen gas was then purged through the reaction mixture under constant stirring and the solution is then kept for 8 h in the sealed condition. The polymer gel formed in each flask containing different proportion of monomer was taken out, washed well with distilled water several times and cut into pieces. The pieces are further subjected to washing by keeping it immersed, first in water and then in methanol for seven days with the routine change of the solvent twice a day. This helped in removal of unreacted monomers from the gel.
Table 1 Compositions of the reactants used in hydrogel preparation
Hydrogel samples |
Methacrylic acid (mol%) |
2-Hydroxyethyl methacrylate (mol%) |
N,N′-Methylenebisacrylamide (mol%) |
Microcrystalline cellulose (wt%) |
Nano cellulose (wt%) |
MH2 |
98 |
2 |
1 |
— |
— |
MH6 |
94 |
6 |
1 |
— |
— |
MH10 |
90 |
10 |
1 |
— |
— |
MH14 |
86 |
14 |
1 |
— |
— |
MH18 |
82 |
18 |
1 |
— |
— |
MHC2A |
98 |
2 |
1 |
0.25 |
— |
MHC2B |
98 |
2 |
1 |
0.5 |
— |
MHC2C |
98 |
2 |
1 |
0.75 |
— |
MHC2D |
98 |
2 |
1 |
1 |
— |
MHC6A |
94 |
6 |
1 |
0.25 |
— |
MHC6B |
94 |
6 |
1 |
0.5 |
— |
MHC6C |
94 |
6 |
1 |
0.75 |
— |
MHC6D |
94 |
6 |
1 |
1 |
— |
MHC10A |
90 |
10 |
1 |
0.25 |
— |
MHC10B |
90 |
10 |
1 |
0.5 |
— |
MHC10C |
90 |
10 |
1 |
0.75 |
— |
MHC10D |
90 |
10 |
1 |
1 |
— |
MHNC2A |
98 |
2 |
1 |
— |
0.25 |
MHNC2B |
98 |
2 |
1 |
— |
0.5 |
MHNC2C |
98 |
2 |
1 |
— |
0.75 |
MHNC2D |
98 |
2 |
1 |
— |
1 |
In case of the cellulose modified hydrogels, the aqueous cellulose suspension is added to the monomer solution drop wise with constant vigorous stirring of the mixture before it was subjected to polymerization, as before. Then the similar methods were followed in the subsequent steps.
In all the cases of hydrogel preparation, the hydrogel samples were dried by keeping in the vacuum oven of temperature 45–50 °C until constant weights were reached.
2.3. Method of characterizations
2.3.1. FTIR spectroscopy. FTIR spectra of the dried gels were taken by using a Perkin Elmer Spectrum Express version 1.03.00 FTIR spectrometer in the range of 4000–500 cm−1. FTIR samples were prepared by mixing one part of the sample with around ten part KBr (IR grade) and then kept under pressure to obtain the thin pellet.
2.3.2. Swelling study. Dry hydrogel samples were allowed to swell in distilled water at different temperatures and in buffer solutions of different pH. The swollen gels were taken out at certain time intervals, wiped on its surface with tissue paper and then weighed and the process was repeated until the constant weight was reached. Equilibrium swelling is measured from the following equation: |
 | (1) |
where We is the weight of the swollen gel after it has reached equilibrium and Wd is the weight of the dry gel.
2.3.3. Deswelling study. Previously weighed dry hydrogel sample was first swollen in distilled water to its equilibrium and then the sample was kept into the NaCl salt solution of strength 0.5 M after wiping its surface with the blotting paper. The gel samples were taken out at certain time intervals, wiped on its surface with tissue paper and then weighed and the process is repeated until the constant weight is reached. Water retained by the hydrogel at any time t is measured from the following equation: |
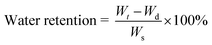 | (2) |
where Wt is the weight of the hydrogel at any time t, Wd is the weight of the dry gel and Ws is the weight of the water absorbed by the hydrogel in equilibrium condition.
2.3.4. Rheological study. In order to investigate the mechanical stiffness of the hydrogel, the rheological measurements of the cylindrical hydrogel samples were performed by strain frequency sweep in Anton Paar, UK oscillatory rheometer at room temperature (25 °C) with a parallel plate geometry (plate diameter = 25 mm, gap = 10 mm) from 100 to 0.1 rad s−1 frequency.
2.3.5. Cryo SEM study. The scanning electron micrographs of the hydrogel samples in swollen condition are taken by using cryogenic SEM apparatus, CARL ZEISS EVO18, Special edition, Germany. At first, the samples were cryo fractured at −187 °C followed by gold coating with coating time of 2 minutes in argon atmosphere at −150° to −160 °C temperature.
2.3.6. Dye separation study. To investigate the efficiency in removal of organic dye (fuchsine) from aqueous solution, poly(AAc-HEMA) hydrogels were placed in aqueous solutions of dye having strength; 50 mg L−1 and allowed to reach equilibrium for 7 days at room temperature. After that period the concentration of the dye remaining in the solution is determined spectrometrically by using UV spectrophotometer Perkin Elmer USA Lambda 25. Aqueous solution of fuchsine showed the maximum absorption peak at about 549 nm respectively. The equilibrium concentration of dye molecules was then determined from the calibration curve obtained with the solutions of known concentration of the dye.
2.3.7. Heavy metal ion removal study. Efficiency of the poly(MAc-HEMA) hydrogels in separating heavy metal ions is determined by keeping the gel immersed in standard metal ion solution (0.1 mol L−1) for 7 days. Then the concentration of the metal ions remaining in the solution is determined conductometrically from a previously established conductance versus metal ion concentration calibration curve.
2.3.8. Dynamic light scattering (DLS) study. DLS study of the nanocellulose suspension was carried out in dynamic light scattering instrument (DLS, Zetasizer, model: Nano-ZS90) procured from Malvern Instruments Ltd., United Kingdom at 25 °C.
3. Result and discussions
3.1. FTIR spectroscopy
In the FTIR spectroscopy of the hydrogel (Fig. 1) the characteristic absorption peaks of the individual reactant units (i.e. methacrylic acid, 2-hydroxyethyl methacrylate, cellulose and N,N′-methylenebisacrylamide) appear at their usual wave numbers. The details of the observed and reference peaks are listed in Table 2.19,20
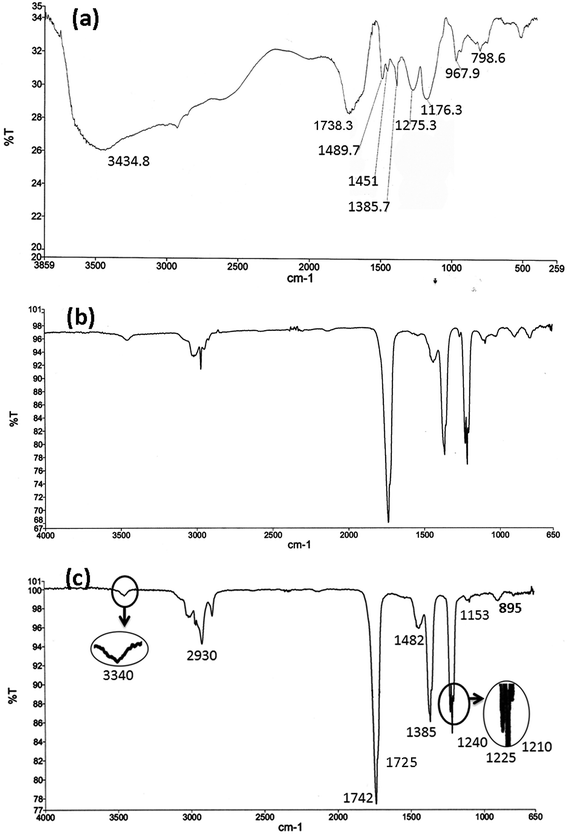 |
| Fig. 1 FTIR spectra of the hydrogel sample (a) MH2 and (b) MHNC2A. | |
Table 2 FTIR peak table of the hydrogel samples MH2, MHC2A, MHNC2A
Hydrogel sample |
Functionality |
Reference peak (cm−1) |
Observed peak (cm−1) |
MH2 |
C O of –COOH & ester |
1735–1750 |
1738.3 |
C–O |
1000–1300 (2 or more bands) |
1176 & 1272 |
O–H of acid |
2500–3300 |
∼3000 |
O–H of alcohol |
3400 |
∼3466 |
–CH2– |
1425 |
1449 |
C–H stretching |
2924 |
2930 |
MHC2A and MHNC2A |
O–H (free) |
3335 |
3340 |
C–C (ring breathing, asymmetric) |
1155 |
1153 |
C–OH |
1200 |
1225 |
C–OH (out of plane) |
1235 |
1240 |
C O |
1735 |
1742 |
CH2 (scissoring) |
1475 |
1482 |
C–H |
2900 |
2930 |
C–H & C–O of polysaccharide aromatic ring |
1373 |
1385 |
C–O–C (glycosidic) |
898 |
895 |
In the FTIR spectra of hydrogel sample MH2, the peaks at 1176, 1275 and 3000 cm−1 can be attributed to the C–O and O–H bonds respectively of –COOH group. C
O stretching peak of –COOH and ester group appear at 1738.3 cm−1. C–H stretching of methylene and methyne groups appears at 1449 and 2930 cm−1 respectively.
Almost similar spectra are observed in case of the cellulose based hydrogels. In both the cases, a broad peak is found to appear at about 3340 cm−1 which is attributed to the hydrogen bonded O–H group.18 C–C asymmetric stretching of ring breathing appears at 1153 cm−1.21 The absorption band at 895 cm−1 is assigned to C–O–C stretching of β(1-4) glycosidic linkage between glucose units of cellulose.22 The peak duos at 1225 cm−1 and 1240 cm−1 are due to the stretching of C–OH bond in the in plane and out of plane geometry respectively. C–H and C–O peaks of polysaccharide ring are observed at 1385 cm−1.21 The peak at 1742 cm−1 is due to stretching of carbonyl (C
O) groups of the poly(MAc-HEMA) unit. Thus it can be assumed that the cellulose particles either in micro or in nano size has been dispersed into the crosslinked polymer network of MAc-co-HEMA. Moreover as no new peaks have been generated or disappeared, it can possibly be inferred that no interactions have taken place in such type of blending.
3.2. Swelling study
3.2.1. Swelling in water. Crosslinked poly(methacrylic acid-HEMA) copolymer is a well-known hydrogel.10,11 It swells remarkably when immersed in water. Water absorption of the poly(methacrylic acid) hydrogel decreases on increasing the HEMA content from 2 to 10 mol% in the polymer network as apparent in the Fig. 2(a). This is as per our expectation. HEMA is a hydrophobic monomer. Incorporation of HEMA in the crosslinked network decreases the hydrophilicity of the network which ultimately leads to reduction in the water absorption of the hydrogel. Besides there is a probability of inter or intra molecular hydrogen bonding between the HEMA and MAc unit which acts as pseudo crosslinks. The extent of this type of H-bonding increases with increasing concentration of HEMA unit in the network. High crosslink density not only plays a role in reducing the water absorption of the hydrogel, but also makes it brittle.
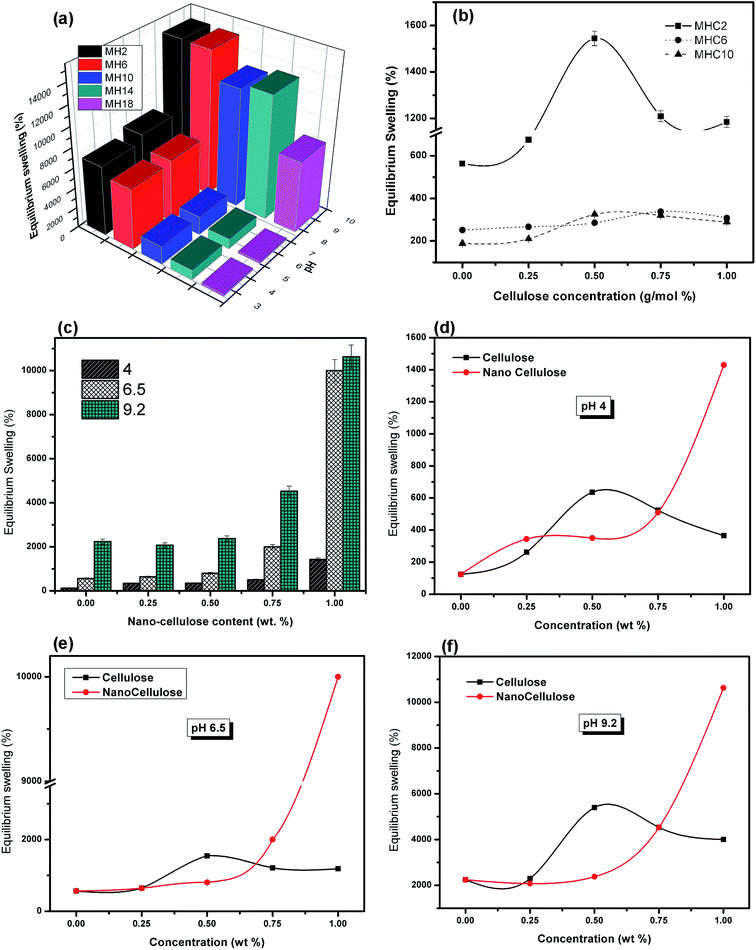 |
| Fig. 2 Equilibrium swelling of the hydrogel samples (a) with varying MAc–HEMA composition at different pH, (b) with different MCC content, (c) at different nanocellulose content and in different pH medium; swelling of the MCC and NC filled hydrogels with the varying feed concentration at pH (d) 4, (e) 6.5 and (f) 9.2 of the medium. | |
Now incorporation of micro crystalline cellulose (MCC) in the MAc–HEMA crosslinked network enhances the swelling (%) upto a certain limit (0.5 wt% of MCC as is observed in our case) in all the representative hydrogel samples under study, after which the swelling (%) decreases as shown in Fig. 2(b). Cellulose is a hydrophilic polymer having large number of pendent –OH groups (2 secondary and 1 primary per anhydro glucose unit). Therefore increase in MCC content is supposed to increase the water absorption of the hydrogels. It may be expected that, during the synthesis of cellulose modified hydrogels the cellulose moieties get deposited on the polymer skeleton of the crosslinked network of poly(MAc-HEMA) [as depicted by the Fig. 3(a)] which helps in increasing water absorption capacity of the hydrogel mainly in two ways – (1) by providing relatively more hydrophilic environment in the crosslinked network and (2) by giving a mechanical support to the pore-walls or acting as spacer in between the crosslinks which helps in extensive stretching of pore-walls (as water enters the pores, it exerts hydrostatic pressure on the pore walls) and also holding large amount of water molecules. But deposition of excess amount of MCC inside the pores (as it might have happened on addition of further amount of cellulose beyond certain optimum of 0.5 wt%) possibly decreases the available space for accommodating the absorbed water molecules and it also makes the pore-channels narrower offering resistance to the entry of water molecules and thus resulting in a decrease of water absorption by the hydrogel.
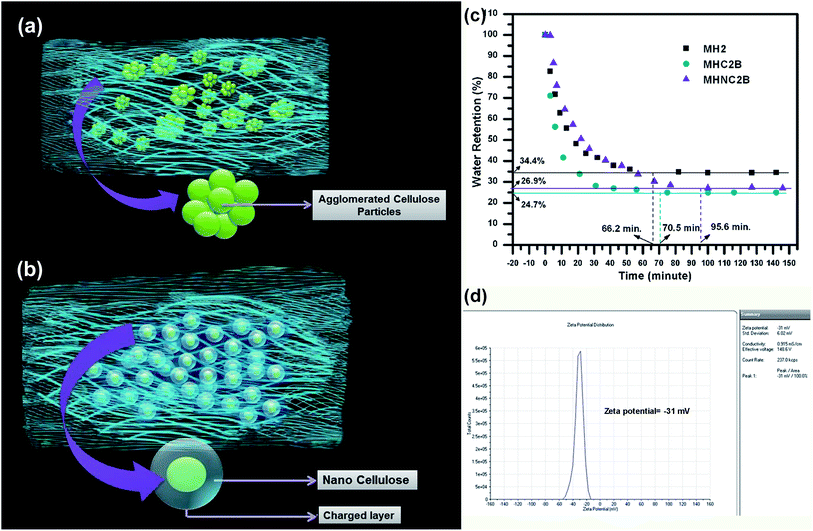 |
| Fig. 3 Schematic representation of the distribution of (a) MCC and (b) NC in the crosslinked polymer matrix of poly (MAc-HEMA); (c) deswelling study of the hydrogel samples MH2, MHC2B and MHNC2B; and (d) zeta potential plot of nanocellulose suspension. | |
On the other hand, increase in the proportion of nanocellulose (NC) in the hydrogel increases the equilibrium swelling of the hydrogel within the entire range of NC content under study (0.25–1 wt%) as revealed in the Fig. 2(c). In case of NC the finely dispersed cellulose particles with nanometric dimension are very well distributed throughout the polymer network in and around the pore walls as shown in the Fig. 3(b). There is little chance of localised deposition or agglomeration as the surfaces of such nanoparticles have been conferred with similar negative charges, SO42−.18 The stable colloidal solution of nanocellulose was confirmed by its zeta potential value of −31 mV determined by DLS study as shown in Fig. 3(d).23 These particles by virtue of having large specific area can pull a large volume of water and hold it. So its incorporation in the crosslinked poly(MAc-HEMA) network not only enhances the hydrophilic nature but also strengthen the elastic nature of the hydrogel system.
3.2.2. Influence of variation in pH on swelling. To determine the pH sensitivity of the neat poly(MAc-HEMA) hydrogels and cellulose modified hydrogels, the dried hydrogel samples were allowed to swell to equilibrium in buffer solutions having different pH at room temperature (25 °C). Due to the presence of MAc unit in copolymer hydrogel structure it may be assumed that the swelling of the poly(MAc-HEMA) hydrogel should depend on the pH of the swelling medium. Swelling of such hydrogels is mainly due to the electrostatic interactions among the charge centres present on the polymer chain. Hence the extent of swelling is supposed to be influenced by any change in physical parameters, such as pH, ionic strength, and type of counter-ions present in the swelling medium which may alter these electrostatic interactions.24 On increasing the pH of the medium, ionisation of the –COOH groups of MAc unit increases which pull the polar water molecules in the crosslinked network resulting in an increase in swelling of the hydrogel.25,26 The interesting part of the investigation lies in the fact that the incorporation of cellulose (MCC) in the poly(MAc-HEMA) hydrogel system enhances the sensitivity to swelling in response to changes in pH of the swelling medium as revealed by the Fig. 2(d)–(f). The swelling of the cellulose based hydrogels (MHC samples) however again increases up to 0.5 wt% of MCC and then undergoes a steady decline in different pH medium under study. Except in the case of acidic media where the equilibrium swelling (%) is relatively lower with respect to the unloaded (without NC), the swelling is remarkably higher in alkaline media. Swelling studies of the hydrogels filled with MCC and NC at different pH have been shown as a function of their loadings in Fig. 2(d)–(f). Water absorption of the cellulose filled poly(MAc-HEMA) hydrogel increases abruptly on increasing the pH, though the sensitivity is much prominent in hydrogels with low HEMA content. This is expected because the increasing proportion of HEMA imparts hydrophobicity and reduces affinity for water. As stated earlier, at higher pH most of the –COOH groups of MAc unit get ionised to form –COO− ions which attracts the water molecules and the hydrophilic networks of cellulose hold them which results in large amount of water absorption at higher pH. The influences of incorporation of cellulose (MCC) may be considered to remain the same at all varying pH media at a given loading of MCC.On the other hand incorporation of nanocellulose in the crosslinked network of poly(MAc-HEMA) improves the pH-sensitivity of the hydrogel even more than that observed with MCC, as evidenced by the Fig. 2(c)–(f). The tremendous increase in water absorptivity in the nanocellulose filled copolymer gel may possibly be ascribed to the synergistic effect of greater ionization of the acidic constituent of the copolymer in a neutral to alkaline medium coupled with the very high specific surface area offered by the tiny cellulose particles, which are also involved in repulsive interactions with the –COO− due to the presence of negative charges on its surface. This might have helped in increasing the pore size.
3.3. Deswelling studies
Deswelling studies of the hydrogels have been carried out with the three representative hydrogel samples MH2, MHC2B and MHNC2B, having the same HEMA content (2 mol%) but the different cellulose content (without cellulose, 0.5% MCC and 0.5% NC respectively). It is apparent from the Fig. 3(c) that the equilibrium water retention value is maximum (34.4%) for the sample without cellulose and minimum (24.7%) for NC based hydrogel sample. The pores of the NC filled hydrogel are stretched to the maximum possible extent when filled with water as it experiences a great force of repulsion between the ionized –COO− of the crosslinked gel network and the surface charges of NC as has already been mentioned. In a congenial atmosphere of deswelling the stretched cells get an opportunity to relax by allowing the water in it to drain out and thus the retained volume of water gets reduced.
Incorporation of MCC in the poly(MAc-HEMA) network results faster rate of deswelling by the hydrogel. More than 70% water was excluded by the hydrogel MHC2B within 30 minutes, but in case of the hydrogel MH2 it was observed to release 55–60% water within that time. Both the hydrogels however reach their equilibrium water retention within 60–65 minute. This may possibly be attributed to the fact that the MCC filled hydrogels have relatively more stretched pore walls compared to the unfilled one and are susceptible to undergo burst release in a favourable condition of deswelling. At the later stages however it behaves in an identical manner to that of MH2.
In case of the nanocellulose filled hydrogel MHNC2B the rate of deswelling was found to be slowest among the three. It took about 90–95 minutes to reach its equilibrium deswelling value and it expels more than 70% water at equilibrium. This is attributed to the fact that the nanocellulose particles are quite active at its surface and are capable of retaining water molecules quite firmly and thus causing obstruction to the exudation of the water and thus delaying the deswelling process.
Besides this, the time taken by the hydrogel to reach the equilibrium water retention value is 6.5% greater in case of MCC based hydrogel and 44.4% greater in case of NC containing hydrogel compared to the hydrogel without any cellulose. That is NC makes the nature of release of water from the hydrogel more slow and sustained.
3.4. Rheology
3.4.1. Storage and loss modulus. Rheological studies of the hydrogels have been carried out in frequency sweep mode. In frequency sweep study, storage and loss moduli of the gels have been plotted as a function of angular frequency. In case of poly(MAc-HEMA) hydrogels the storage and loss moduli (G′ and G′′ respectively) values are almost independent of the angular frequency as shown in Fig. 4(a) which represents the rheological behaviour of the copolymers of different composition. In all the cases the storage modulus is higher than the loss modulus which is typical gel behaviour of having higher elastic component than the viscous one.27 Storage modulus of the hydrogel increases with the HEMA content in the crosslinked network. This is quite expected as the hydrophobic HEMA unit improves the mechanical properties of gel. As has been discussed in the previous section that there is a probability of pseudo crosslink by H-bond formation between the –COOH group of the MAc and –OH group of the HEMA, which resist the slippage of polymer chain layers and thus increase the storage modulus. Similarly the corresponding loss moduli value of the hydrogels follows the similar trend, as those of the storage moduli.
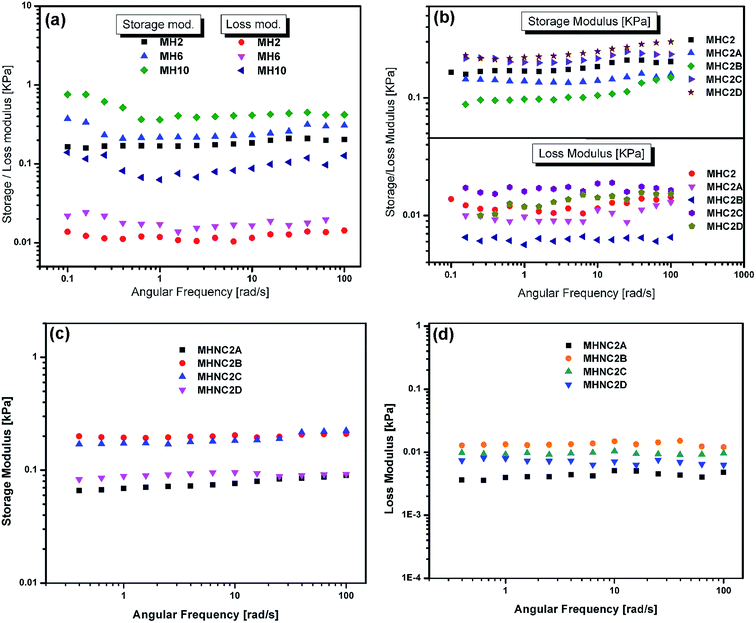 |
| Fig. 4 Rheological properties of the hydrogels: storage and loss modulus with the variation of angular frequency of hydrogel samples (a) MH2, MH6, MH10 (b) MHC2, MHC2A, MHC2B, MHC2C and MHC2D; variation of (c) storage and (d) loss modulus with the angular frequency of MHNC2A, MHNC2B, MHNC2C and MHNC2D. | |
In order to study the influence of MCC and NC the hydrogel containing 2 mol% of HEMA has been considered. Incorporation of MCC in the (MAc–HEMA) polymer network causes reduction in storage modulus up to 0.5%. Further incorporation of MCC causes enhancement in storage moduli as shown in Fig. 4(b). As the water absorption of the hydrogel was found to increase with the increase in MCC content up to 0.5% (as shown earlier), within this range it may be considered that the relative concentration of elastic component of the polymer network decreases as the MCC, incorporated in situ may help to reduce the degree of crosslinking by acting as spacer. In addition the increased absorptivity might help in increasing the plasticization effect. However beyond this optimum concentration (0.5%), the MCC can undergo both intra and inter molecular H-bonding which acts as pseudo crosslinks and increases the storage moduli.
Although the mode of changes in loss moduli follows almost similar trend as that of storage modulus, however all the values lie below that of the unloaded specimen in the entire range of frequency under study.
Similarly in case of NC incorporation, it is expected that the G′ should decrease with the increase in NC content and an increase in water absorption of the hydrogel should occur. But in actual practice G′ increases with the NC content from 0.25 to 0.5% and then it decreases on further incorporation as shown in Fig. 4(c). This may be explained by considering two contradictory factors – (i) with the increase in NC content, the hydrophilicity of the polymer network increases which results in an increase in swelling and a decrease of the elastic component, and (ii) the mechanical strength of the MAc–HEMA copolymer should increase due to homogeneous distribution of nano particles. Now, the incorporation of NC from 0.25 to 0.5% results little increase (18.1%) in water absorption. Hence the enhancement of mechanical strength of polymer backbone by incorporation of NC predominates over the water absorption factor in determining the ultimate rheological behaviour of the hydrogel and so G′ increases with the NC content. Further incorporation results large increase in swelling (90.6 and 134.7% respectively) of the hydrogel and thus G′ decreases as a consequence as the viscous component takes over. Here also the loss moduli follow the similar trend [Fig. 4(d)] as that of the storage moduli. At the initial stage of NC incorporation the strong adsorptive forces exerted by the NC particles on the pore walls helps to enhance the mechanical strength of the gel while at the later stages of its incorporation the excess NC over that adsorbed on the pore walls only help in absorbing water and thus enhanced swelling is observed.
3.5. Morphology by cryo SEM
Typical porous nature of the hydrogel has been revealed in all the hydrogels MH2, MHC2A, MHC2B and MHC2C [Fig. 5(a)–(f)]. Thin pore wall has been seen in Fig. 5(a) in case of poly(MAc-HEMA) hydrogel (MH2) which contains no cellulose. Incorporation of micro crystalline cellulose in the crosslinked network however causes deposition of the same on the pore walls of the MAc–HEMA polymer skeleton which results in mild thickening of the pore walls of the polymer backbone as revealed in the cryo SEM images of MHC2A and MHC2B [Fig. 5(b) and (c) respectively]. Fig. 5(e) and (f) are the portion-zoomed images of Fig. 5(c) and (d) respectively. Due to the cellulose deposition, the hydrophilicity of the polymer network increases resulting increase in swelling of the hydrogels as discussed earlier. But at higher cellulose content in case of hydrogel MHC2C, the pore wall become very much thick [Fig. 5(d)] making the pores narrow which prevents the entry of water molecules in the crosslinked network. At higher MCC content the space within the pores is greatly reduced to accommodate and hold water in much less amount compared to that obtained with gels containing lower doses of MCC.
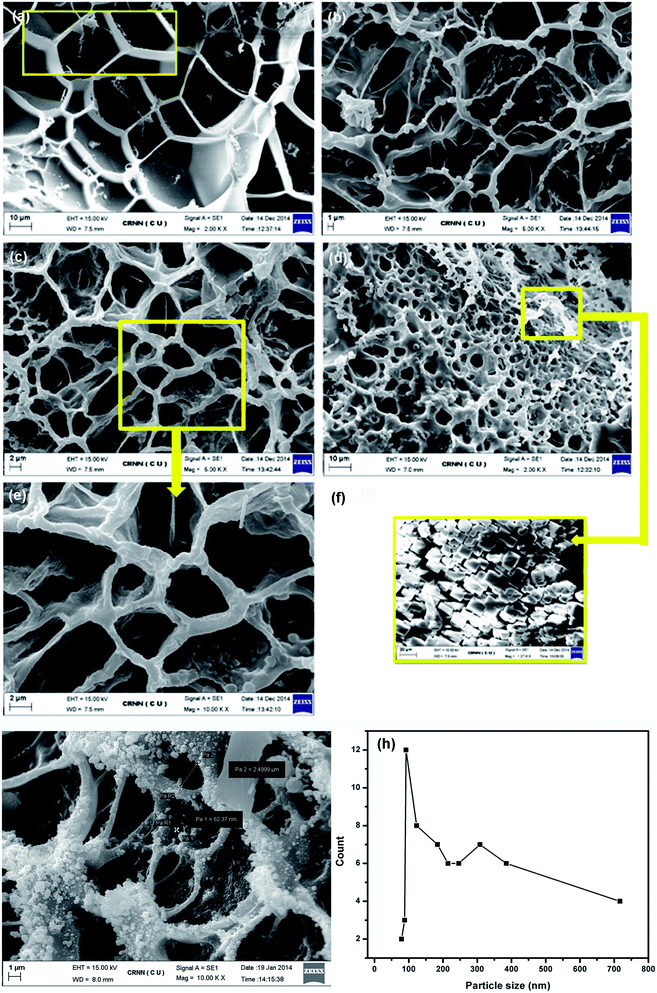 |
| Fig. 5 Cryo SEM images of (a) MH2, (b) MHC2A, (c) MHC2B, (d) MHC2C; portion zoomed image of (e) MHC2B (f) MHC2C; (g) MHNC2A and (h) particle size distribution of the sample MHNC2A. | |
In case of nanocellulose based hydrogel, the nano-size cellulose particles have been found on the cell wall with the diameter ranging from 80–700 nm and maximum at ∼107 nm as shown in the Fig. 5(g) and (h). The surfaces of the pores also hold some NC particles.
3.6. Application studies
3.6.1. Dye separation study. Poly(MAc-HEMA) hydrogels have the ability to adsorb cationic dyes from its aqueous solution. The adsorption study was performed with methyl violet and fuchsine dyes. The cationic dye molecules get trapped in polymer network by the electrostatic interaction with the –COOH group of the MAc unit. The hydrophobic segment of the HEMA unit also stabilizes the hydrophobic aromatic ring of the dye molecules.28,29 The dye adsorption capacity of the present hydrogel increases on increasing the cellulose content in the polymer network as shown in Fig. 6(a). Cellulose molecules cover the polymer and make a hydrophilic bed from which the pendent polar –OH groups grasp the cationic dye molecules by electrostatic interaction. No significant difference has been observed between the MCC and NC contained hydrogels in respect of dye removal within the range under studies.
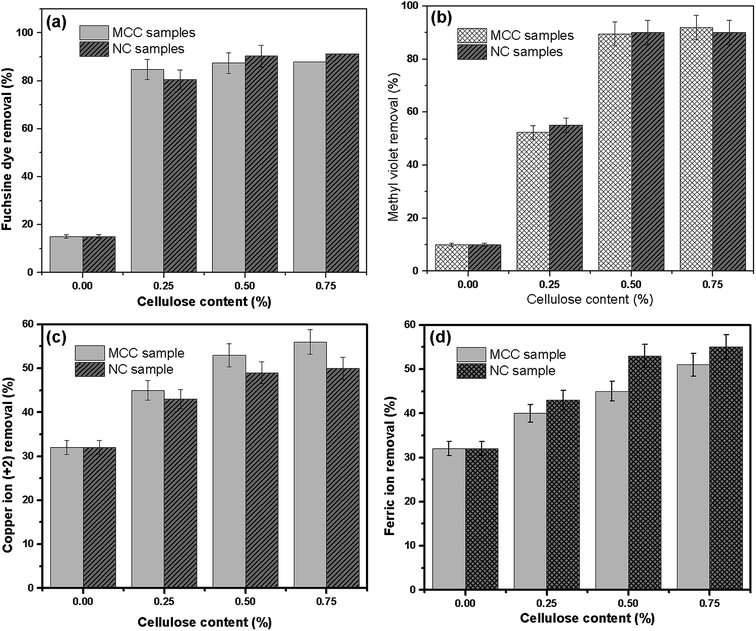 |
| Fig. 6 (a) Fuchsine, (b) methyl violet dye, (c) cupric ion and (d) ferric ion removal by the MCC and NC based hydrogels with the respective cellulose content of each type. | |
3.6.2. Heavy metal separation. The copolymer gel by itself anionic in nature is expected to attract or adsorb cationic species. Thus in absence of any cellulose the gel with 2 mol% of HEMA is observed to remove about 31% of Cu2+ ion from an aqueous solution of CuSO4 present to the extent of 0.1 mol L−1 on immersing the gel sample in it for seven days. Adsorption of metal ions increases with the increase in cellulose content in the polymer network of the hydrogel irrespective of its nature i.e. MCC or NC. Multivalent cations tend to form chelate complexes with the ionic pendent groups of the polymer when it comes in contact with the salt solutions and as a result the metals get trapped in the crosslinked polymer network.29 Cellulose molecules have large number of –OH groups; they can also form chelate complexes by donation of lone pair electron (present in oxygen) to the metal ion. Hence on increasing the cellulose content the adsorption of metal ions also increases within the concentration range under investigation [Fig. 6(c) and (d)]. However it is interesting to note that the efficiency of the metal ion removal is somewhat dependent on the nature of the metal ion and its cationic charge. On comparing the extent of removal of a cupric solution to that of a ferric solution we can find from the Fig. 6(c) and (d) that the gel is more efficient in removing the ferric ion than the cupric ion. Moreover although the MCC and NC do not differ too much in so far the extent of removal in cupric solution is concerned, the NC filled copolymer gel exhibits an increasing efficiency over the MCC filled samples in the entire range of NC incorporation under study.
4. Conclusions
Micro crystalline cellulose and nanocellulose have been introduced separately into the hydrogel network of poly(MAc-HEMA) and the effect of such incorporation on the hydrogel properties has been investigated. The swelling of the hydrogels decreases on increasing the hydrophobic HEMA content and it increases on increasing the cellulose content in the polymer network. It occurs due to deposition of cellulose around the hydrogel pores formed by the polymer, which is confirmed by SEM images. Rheological properties of the hydrogel largely depend on the cellulose content. The hydrogels were capable of absorbing cationic dyes and heavy metal ions from aqueous solutions and the efficiency is much greater in cellulose based hydrogels compare to other.
5. Future scope of work
Both type hydrogels are pH responsive, cellulose containing hydrogels being the more efficient. The smartness of the hydrogels can be used in drug delivery applications. Hence there are some area of further investigation on it, such as drug encapsulation–release and their kinetics by using the hydrogel as drug delivery vehicle.
References
- W. E. Hennink and C. F. van Nostrum, Adv. Drug Delivery Rev., 2012, 64, 223–236 CrossRef.
- A. S. Hoffman, Adv. Drug Delivery Rev., 2012, 64, 18–23 CrossRef.
- S. H. Gehrke, Drugs Pharm. Sci., 2000, 102, 473–546 CAS.
- N. A. Peppas, Y. Huang, M. Torres-Lugo, J. H. Ward and J. Zhang, Annu. Rev. Biomed. Eng., 2000, 2, 9–29 CrossRef CAS PubMed.
- Y. Qiu and K. Park, Adv. Drug Delivery Rev., 2012, 64, 49–60 CrossRef.
- G. D. Prestwich, D. M. Marecak, J. F. Marecak, K. P. Vercruysse and M. R. Ziebell, J. Controlled Release, 1998, 53, 93–103 CrossRef CAS PubMed.
- D. Campoccia, P. Doherty, M. Radice, P. Brun, G. Abatangelo and D. F. Williams, Biomaterials, 1998, 19, 2101–2127 CrossRef CAS PubMed.
- M. Přádný, P. Lesný, J. Fiala, J. Vacík, M. Šlouf, J. Michálek and E. Syková, Collect. Czech. Chem. Commun., 2003, 68, 812–822 CrossRef.
- D. Klinger and K. Landfester, Macromolecules, 2011, 44, 9758–9772 CrossRef CAS.
- A. M. AL-Baradi, M. Mears, R. A. L. Jones and M. Geoghegan, J. Polym. Sci., Part B: Polym. Phys., 2012, 50, 1286–1292 CrossRef CAS.
- D. M. García, J. L. Escobar, N. Bada, J. Casquero, E. Hernáez and I. Katime, Eur. Polym. J., 2004, 40, 1637–1643 CrossRef.
- C. Chang, A. Lue and L. Zhang, Macromol. Chem. Phys., 2008, 209, 1266–1273 CrossRef CAS.
- C. Chang, B. Duan, J. Cai and L. Zhang, Eur. Polym. J., 2010, 46, 92–100 CrossRef CAS.
- N. Roy, N. Saha, T. Kitano and P. Saha, J. Appl. Polym. Sci., 2010, 117, 1703–1710 CAS.
- Y. Bao, J. Maa and N. Li, Carbohydr. Polym., 2011, 84, 76–82 CrossRef CAS.
- P. Liu, M. Zhai, J. Li, J. Peng and J. Wu, Radiat. Phys. Chem., 2002, 63, 525–528 CrossRef CAS.
- P. Liu, J. Peng, J. Li and J. Wu, Radiat. Phys. Chem., 2005, 72, 635–638 CrossRef CAS.
- A. Mandal and D. Chakrabarty, Carbohydr. Polym., 2011, 86, 1291–1299 CrossRef CAS.
- A. M. Adel, H. Abou-Youssef, A. A. El-Gendy and A. M. Nada, Nat. Sci., 2010, 8, 8 Search PubMed.
- E. Akar, A. Altınışık and Y. Seki, Carbohydr. Polym., 2012, 90, 1634–1641 CrossRef CAS PubMed.
- D. Ciolacu, F. Ciolacu and V. I. Popa, Cellul. Chem. Technol., 2011, 45, 13–21 CAS.
- C. Pappas, P. A. Tarantilis, I. Daliani, T. Mavromoustakos and M. Polissiou, Ultrason. Sonochem., 1999, 5, 163 CrossRef.
- Y. M. Zhou, S. Y. Fu, L. M. Zheng and H. Y. Zhan, eXPRESS Polym. Lett., 2012, 6, 794–804 CrossRef CAS.
- P. L. Ritger and N. A. Peppas, J. Controlled Release, 1987, 5, 23–36 CrossRef CAS.
- M. Jeria-Orell, G. del C. Pizarro, O. G. Marambio, M. Huerta and K. E. Geckeler, J. Appl. Polym. Sci., 2006, 100, 1735–1741 CrossRef CAS.
- R. Da Silva and M. Ganzarolli de Oliveira, Polymer, 2007, 48, 4114–4122 CrossRef CAS.
- Y. Chen, L. Chen, H. Bai and L. Li, J. Mater. Chem. A, 2013, 1, 1992–2001 CAS.
- E. Karadağ, D. Saraydin and O. Güven, Polym. Adv. Technol., 1997, 8, 574–578 CrossRef.
- R. Bera, A. Dey, A. Dutta sarma and D. Chakrabarty, RSC Adv., 2015, 5, 75870–75880 RSC.
|
This journal is © The Royal Society of Chemistry 2016 |