DOI:
10.1039/C5RA23968K
(Paper)
RSC Adv., 2016,
6, 17995-18001
A chemosensor for Al3+ ions in aqueous ethanol media: photophysical and live cell imaging studies†
Received
13th November 2015
, Accepted 26th January 2016
First published on 28th January 2016
Abstract
A novel fluorescent chemosensor L has been designed and synthesized based on the rhodamine-B moiety. The dye L exhibits selective fluorescence enhancement towards the Al3+ ion over other biologically relevant metal ions in aqueous ethanolic media (EtOH–H2O, 2
:
3, v/v). The structure of the probe L has been established by 1H and 13C-NMR spectroscopy, single crystal X-ray diffraction, ESI-mass spectrometry and elemental analysis. The cleavage of the spirolactam bond of the rhodamine moiety induced by the Al3+ ion generates the delocalized xanthene fluorophore that is responsible for the emission enhancement of the probe L. The recognition behavior of the receptor L has been investigated experimentally with supports from theoretical DFT studies. Furthermore, the efficacy of L in cell imaging studies is also probed by confocal microscopy.
Introduction
The design and synthesis of fluorescent probes for environmentally hazardous metal ions has achieved considerable recent interest in terms of using these probes for chemosensing, live cell imaging, ion transport, metalloenzyme mimics, catalysis and nuclear waste treatment.1–3 Extensive use of aluminum in food additives, pharmaceuticals, electrical equipment, cooking utensils, computer parts etc.4 leads to environmental problems. In humans, it exists5 in its ionic form Al3+ with a long residence time before excretion through urine. Accumulation of this ion can be potentially hazardous affecting neurological order that may lead to Parkinson's disease, Alzheimer's disease etc.6,7 It also hampers the growth of plants and biological functions of metalloenzymes.8 Therefore, spatial as well as temporal distribution of Al3+ ions even at low concentration is highly desirable.
Our interest in developing chemosensors for the Al3+ ion led us to synthesize the dye L to utilize the excellent photophysical properties of rhodamine B as a turn-on sensor.9–11 Several rhodamine derivatives have been reported as sensors for the Al3+ ions.12–17 Most of them are functional only in organic solvents due to strong hydration of Al3+ in water thus making them difficult for practical applications.18 To increase aqueous solubility of the chemosensor, 2-methoxy-4-nitroaniline group, has been appended as the receptor to the rhodamine B moiety (Scheme 1) as it can increase the hydrophilic interaction by enhancing the polar character in the molecule.19
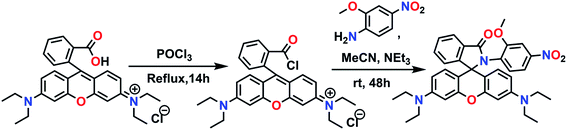 |
| Scheme 1 Synthetic route of the chemosensor L. | |
The dye is well-characterized by 1H- and 13C-NMR spectroscopy, single crystal X-ray diffraction, ESI-mass spectrometry and elemental analyses. It acts as a fluorescence turn-on chemosensor for the Al3+ ion in aqueous ethanol medium.
Experimental section
Materials and methods
Reagent grade rhodamine B and all metal perchlorate and nitrate salts were from Sigma Aldrich (USA). 2-Methoxy-4-nitroaniline was obtained from Alfa Aesar (USA). Other chemicals such as triethylamine, POCl3 and EDTA were obtained from S. D. Fine Chemicals (India). These chemicals were used as received without further purification. All the solvents were from S. D. Fine Chemicals and were dried following standard protocols prior to use. Chromatographic separation was done by column chromatography using basic Al2O3 from S. D. Fine Chemicals (India).
Caution! Perchlorate salts are potentially explosive especially if they are mixed with organic solvents and should be handled with utmost care.
Analysis and measurements
Both 1H NMR (500 MHz) and 13C NMR (125 MHz) spectra of the compounds were recorded on a JEOL JNM-LA500 FT spectrometer in CDCl3 with tetramethylsilane as the internal standard. The ESI-mass data were obtained in acetonitrile using a WATERS-Q-TOF Premier Mass Spectrometer. UV-vis absorption spectra were recorded on a Shimadzu 2450 UV-vis spectrophotometer in aqueous ethanol medium at 298 K. Steady-state fluorescence spectra were obtained using a Perkin-Elmer LS 50B Luminescence spectrometer at 298 K with excitation and emission band-pass of 10 nm. The excitation wavelength was 520 nm and the spectra were recorded in the range 540–700 nm. Fluorescence quantum yields were determined by comparing the corrected spectra with that of pure rhodamine B in ethanol (ESI†).
Single-crystal X-ray data of compound L was collected at 100 K on a Bruker SMART APEX CCD diffractometer using graphite monochromated MoKα radiation (λ = 0.71073 Å). The data reduction, structure solution and refinements were carried out using the SHELXL-97 package. The details are also provided in the ESI.†
Jobs plot and Benesi–Hildebrand plot
Job's plot experiment was conducted, keeping the total concentration of L and L–Al3+ complex at 1 × 10−4 mol L−1 and the mole fraction of Al3+ varied from 0 to 1. The sensor L (2.96 mg) was dissolved in 5 mL ethanol to make a solution of 10−3 M order and diluted to 25 mL 1.0 × 10−4 M order by addition of ethanol and water (EtOH
:
H2O = 2
:
3, v/v). 2.7, 2.4, 2.1, 1.8, 1.5, 1.2, 0.9, 0.6 and 0.3 mL stock solution of L were taken and kept them into vials. 25 mL 1.0 × 10−4 M stock solution of Al(NO3)3·9H2O was also prepared in similar way in ethanol. 0.3, 0.6, 0.9, 1.2, 1.5, 1.8, 2.1, 2.4 and 2.7 mL Al3+ stock solution were to the vials containing solution of sensor in such a way that total volume of each vial were 3 mL. After shaking the vials UV-vis absorbance were performed at room temperature.
Similarly for Benesi–Hildebrand experiment a series of stock solution of Al(NO3)3·9H2O were prepared from 1 × 10−6 to 1 × 10−3 M range in different vials and added separately to 1 × 10−5 M solution of L. After mixing the vials fluorescence spectra were recorded at room temperature.
Cell culture and imaging
MTT [3-(4,5-dimethylthiazol-2-yl)-2,5-diphenyltetrazolium bromide] and dimethyl sulphoxide (DMSO) of analytical grade were procured from Sigma-Aldrich Inc. (St-Louis, MO, USA); Dulbecco's modified Eagle's medium (DMEM), fetal bovine serum, and antibiotics were procured from Gibco BRL (Grand Island, NY, USA). All organic solvents used were of HPLC grade. Briefly, the rat skeletal muscle cells of L6 cell line obtained from National Centre for Cell Science, Pune, India, were grown in Dulbecco's modified Eagle's Medium (DMEM) supplemented with 10% foetal bovine serum and 1% antibiotic in a 5% carbon dioxide incubator maintained at 37 °C and plated in different Petri-plates.20 All the control and experimental sets of cells were grown till they attained 70–80% confluence, and then the different treatment procedures commenced. The concentration of the sensor optimized for the study was estimated at 7 × 10−5 M (20 mL of total volume of stock solution); it was incubated for 15 min and the concentration of that of Al(NO3)3 salt solution standardized at 4 × 10−3 M (5 mL of total volume of stock solution) was also incubated for 15 min. Cell images were taken under an Andor Spinning Disk Confocal Microscope. The images were then evaluated against untreated control set of cells. The fluorescence intensity profile was derived using Analysis Software, NIS Elements AR Version 4.00.
Synthesis of the chemosensor L
Synthesis of the chemosensor was easily achieved in two steps as illustrated in Scheme 1.
To rhodamine B (2 g, 4.2 mmol) taken in a three-necked round bottom flask, 50 mL of freshly distilled phosphorous oxychloride was added and the mixture was heated reflux for 24 h under a dinitrogen blanket. Excess POCl3 was then distilled off and the acid chloride formed was dried under reduced pressure and used for the next step without further purification. The crude acid chloride was dissolved in dry acetonitrile (70 mL) and treated with 2-methoxy-4-nitroaniline (1.2 equivalents) in presence of triethylamine (2 mL) taken in the same solvent (50 mL) over a period of 20 min. After the addition was complete, the reaction mixture was allowed to stir at room temperature for 48 h and then all the solvent was removed under reduced pressure. The crude reaction mixture was dissolved in dichloromethane (3 × 50 mL) and washed several times with water. The organic layer after drying over anhydrous Na2SO4, was evaporated off completely to afford a dark pink solid. Column chromatography on basic Al2O3 using DCM/hexane (70
:
30, v/v) as the eluent provided the desired product as a pale yellow crystalline sold in ∼58% yield. Mp ∼ 210 °C (uncorrected); 1H NMR (500 MHz, CDCl3, 25 °C, Si(CH3)4) δ: 1.13 (t, 12H, J = 7.45 Hz), 3.29 (q, 8H, J = 6.85 Hz), 3.58 (s, 3H), 6.19 (d, 2H, J = 2.3 Hz), 6.29 (dd, 2H, J = 2.3, 8.75 Hz), 6.38 (d, 1H, J = 8.05 Hz), 6.65 (d, 2H, J = 8.55 Hz), 7.21 (s, 1H), 7.53–7.55 (m, 4H), 8.00–8.02 (m, 1H) (Fig. S1†); 13C NMR (125 MHz, CDCl3, 25 °C, Si(CH3)4) δ: 12.66, 44.44, 56.07, 68.38, 97.48, 105.76, 106.85, 107.68, 115.51, 123.59, 124.39, 128.47, 129.59, 129.72, 131.46, 131.92, 133.02, 147.99, 148.94, 152.89, 153.54, 156.56, 166.69 (Fig. S2†). ESI MS: m/z (%): 593.2761 (100) [M + H+]+ (Fig. S3†); anal. calcd for C35H36N4O5: C, 70.93; H, 6.12; N, 9.45%. Found: C, 71.07; H, 6.28; N, 9.32%.
Results and discussion
The chemosensor L can be synthesized from readily available chemicals in two steps. The rectangular parallelepiped pale yellow single crystals, suitable for X-ray crystallography were obtained by slow evaporation of the solution of the compound in ethyl acetate at room temperature. A perspective view of the molecule is shown in Fig. 1 while the crystallographic data are collected in Table S1 in the (ESI†).
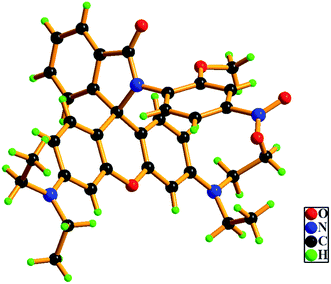 |
| Fig. 1 X-ray single crystal structure of L. | |
Photophysical properties
The probe is readily soluble in common organic solvents and also has sufficient solubility in aqueous-organic medium. All spectroscopic measurements were carried out in aqueous ethanol (EtOH–H2O, 2
:
3 v/v) medium. Perchlorate salts of metal ions that include Na+, K+, Mg2+, Ca2+, Mn2+, Fe2+, Fe3+, Co2+, Ni2+, Cu2+, Zn2+, Cd2+, Ag+, Pb2+ and Hg2+ were used in the photophysical studies. For Al3+ and Cr3+ ions nitrate salts were used. The metal free dye L exhibits negligible absorption and fluorescence above 500 nm implies the retention of the spirolactam bond in this region. However, addition of 10 equivalents of the Al3+ ion to a solution of L makes the solution from colorless to pink in about 20 min that can be detected with a naked eye. In the visible region, a strong band appears at 565 nm with a shoulder around 525 nm (Fig. 2) indicating breaking of the spirolactam bond in presence of the Al3+ ion. No such significant change is observed in presence of any of the aforementioned metals ions.
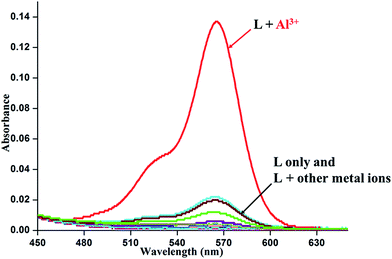 |
| Fig. 2 Absorption spectra of L in EtOH–H2O (2 : 3, v/v) medium in the presence of 10 equivalents of metal ions. [L] = 1 × 10−4 M. | |
Upon excitation at 520 nm, the metal-free chemosensor L (Φ = 0.0065) exhibit only a very weak fluorescence. In sharp contrast, addition of Al3+ ion to the solution of L leads to a strong emission (∼50 fold enhancement) giving a band centering around 580 nm (Φ = 0.328) (Fig. 3).
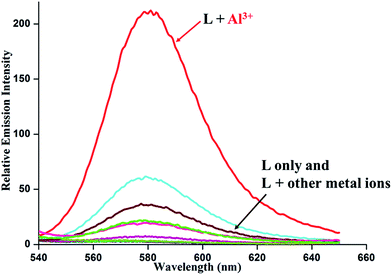 |
| Fig. 3 Relative emission spectra of L in EtOH–H2O (2 : 3, v/v) in presence of 10 equivalents of metal ions. [L] = 10 μM. Slit width = 10/10 nm. λex = 520 nm. | |
Other metal ions listed above do not elicit any noticeable emission when added to the solutions of L. These results are consistent with the absorption spectral results. To confirm the spirocyclic ring opening, the 13C NMR spectra of the complexes were also recorded. The absence of the characteristic peak of the quaternary carbon in the region, 67.0–69.0 ppm (ref. 21 and 22) in the metal complex (Fig. S4†) reveals the rupture of the spirolactam bond to the ring-open form. In the IR spectra of the metal free sensor L the amide carbonyl frequency appears at 1709 cm−1. The downward shift around 1589 cm−1 (Fig. S5†) of the amide carbonyl stretching in the complexes is consistent with fact that the carbonyl oxygen is involved in the coordination. In addition, a peak in the region 1370–1384 cm−1 suggests the presence of coordinated nitrate ion (NO3−) in the complex.
Theoretical study
For better understanding about the nature of binding of the ligands, theoretical calculations were performed using Gaussian-09 software. For the comparative study, various molecular interactions of the ligand L and L–Al3+ complex has been studied using density functional theory (DFT) with the B3LYP/6-31G (d,p+) functional model and basis set. Energy optimization calculations have revealed that L–Al3+ complex is energetically much more stable compared to the ligand L. Here the energy gap between the HOMO and LUMO of the complex (2.28 eV) was less compared to that of the corresponding sensor L (2.79 eV) (Fig. 4).
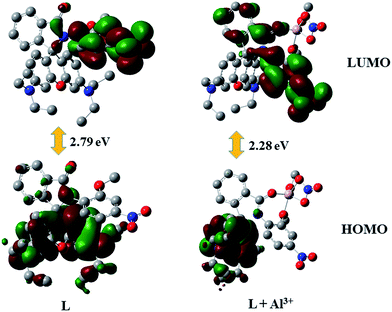 |
| Fig. 4 Optimized structures of HOMO and LUMO of the ligand L and L–Al3+ complex. | |
From the spatial electronic distribution in the frontiers orbitals of the ligand L it can be easily pointed out that in the free ligand the electron density mainly resides on the xanthene moiety and some electron clouds on C
O moiety. In the complexes, spirolactam bonds were opened when the Al3+ ions bind to the ligand. These structural change introduced by the attachment of the metals ions lead to significant charge transfer from the xanthene moiety towards the metal binding moiety that could be well observed from the frontiers orbitals of the complex. Here the electron charge cloud was pulled towards the electron withdrawing nitro group in the LUMO. So here the extent of these transfer of electron cloud have played crucial role to this tuning of selectivity towards the tripositive ions. The optimized structures of the sensor L and L–Al3+ complexes are summarized in Fig. S9.†
Selectivity experiment
In order to check the selectivity of L towards Al3+ ions over the other tested metal ions competitive studies were carried out (Fig. 5). The emission responses of the sensor L towards Al3+ ion in the presence of excess of background metal ions are also recorded. In presence of other competing metal ions, no significant change in the emission intensity has been noticed in the sensing of Al3+ ion confirming its selectivity towards the Al3+ ion.
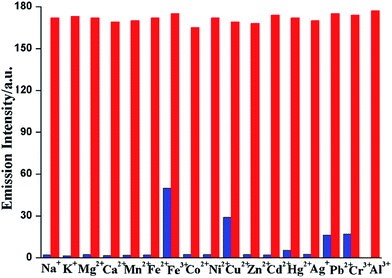 |
| Fig. 5 Selectivity of the dye L for Al3+ ion in EtOH–H2O (2 : 3, v/v) mixture. Blue bars indicate the fluorometric response of L with 50 equivalents of the metal ion of interest; red bars represent the final integrated fluorescence response after the addition of 10 equivalents of Al3+ ion to each solution containing other metals over the initial integrated emission. Dye concentration = 10 μM; λexc = 520 nm. | |
The binding stoichiometry was determined by the Job's plot experiments based on absorption keeping the total concentration of L and Al3+ ion at 1 × 10−4 M (Fig. 6).23 The experiments support 1
:
1 complexation between L and Al3+. A peak at 743.3220 due to [L + Al3+ + 2NO3−]+ in the ESI-mass spectrum of the Al3+ complex further confirms this 1
:
1 binding stoichiometry (Fig. S6†).
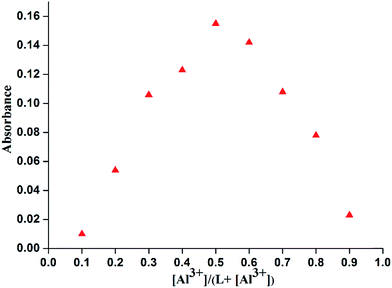 |
| Fig. 6 Job's plot of absorbance of L and Al3+ in EtOH–H2O (2 : 3, v/v) medium. Total concentration = 1 × 10−4 M. | |
The association constant for the complex and the detection limit were determined from emission titration data (Fig. 7).24 Following the Benesi–Hildebrand plot25 the association constant of L with Al3+ ion is found to be 6.53 × 103 M−1 (R2 = 0.99496) (Fig. 8a). This value matches well with the reported range (103 to 109 M−1) of the association constants for the Al3+ specific chemosensors reported in the literature.26,27 The detection limit is calculated to be 0.316 μM (Fig. 8b) which is smaller compared to the maximum concentration of 7.41 μM of Al3+ ion in drinking water approved by WHO.28 Also the calculated value of detection limit is found to lie in comparable range (1.0 × 10−6 M) for the reported Al3+ sensors.13,29–34
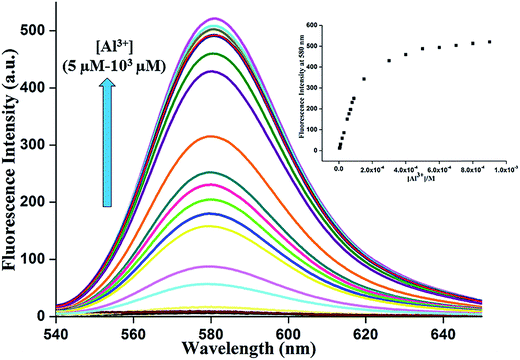 |
| Fig. 7 Change of fluorescence spectra of the dye L as a function of [Al3+] ion in neutral EtOH–H2O (2 : 3 v/v) medium. The arrow indicates the trend in increasing [Al3+]. | |
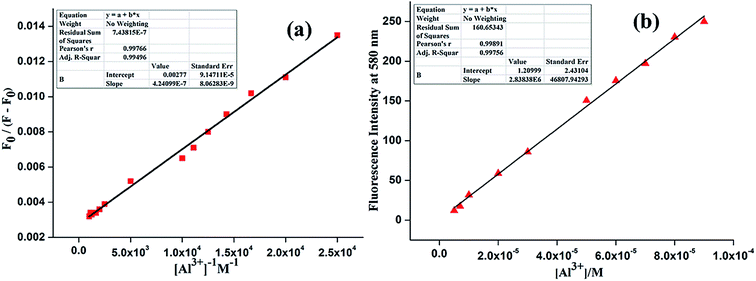 |
| Fig. 8 (a) Binding constant plot of L for Al3+. (b) Linear response curve of L at 580 nm depending on the Al3+ ion concentration for determination of lowest detection limit. | |
Reversibility of fluorescence is an imperative characteristic in developing chemosensors for practical aspects. The reversible nature of the sensor L is checked by adding an aqueous solution of Na2EDTA to the solution of metal complex. Addition of excess EDTA salt solution results in the lowering of emission intensity to the levels of metal-free sensors (Fig. 9).
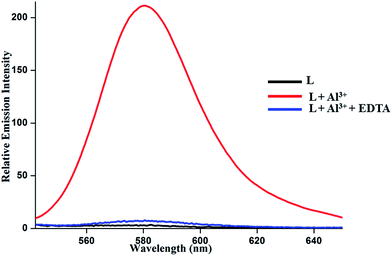 |
| Fig. 9 Emission responses of L in the presence of 10 equivalents Al3+ ion and subsequent addition of excess EDTA solution to the L complex of Al3+ ion in EtOH–H2O (2 : 3, v/v) medium. | |
Cell imaging studies
The potentiality of a sensor to determine the presence of guest species in living cells is of immense practical importance.35 To confirm the competence of the chemosensor in biological samples the intracellular confocal imaging studies have been carried out in the presence of Al3+ ion. No distinct intracellular fluorescence was observed for the series of cells incubated with normal untreated cells (B) or sensor (H) alone. However, significant increase in the fluorescence was gradually observed in case of sensor L when exogenous Al3+ stock solution was added into them via incubation with Al(NO3)3 salt solution (N) (Fig. 10). This finding corroborated well with the statistically analyzed fluorescence intensity profile (Fig. S7†). Counterstaining of the cells was also done with Hoechst dye to confirm the permeability range of the sensors which showed a more prominent distribution in the cytoplasm than that of the nucleus (Fig. 10).
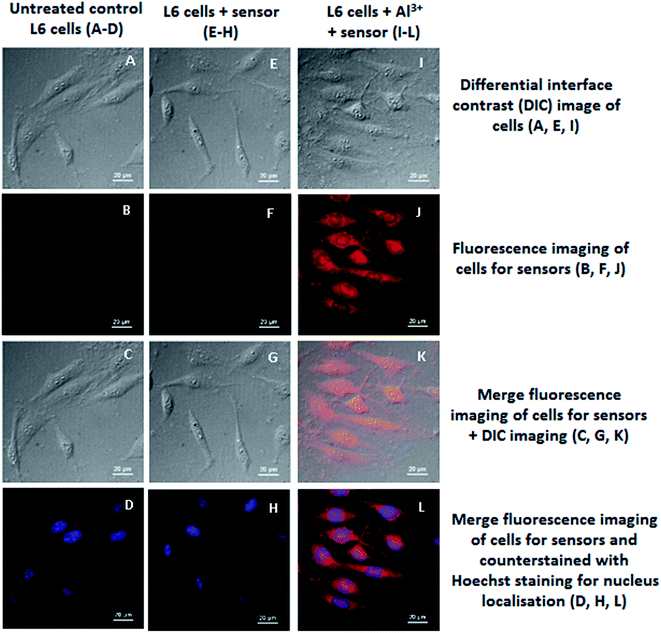 |
| Fig. 10 Confocal imaging of control and treatment series of L6 cells for sensor L viewed under 40× magnification; the scale bar provided in each image measures 20 μm in size: (A–D) Untreated control L6 cells; (E–H) L6 cells + sensor; (I–L) L6 cells + Al3+ + sensor; differential interface contrast (DIC) image of untreated control L6 cells (A), L6 cells + sensor (E) and L6 cells + Al3+ + sensor (I); fluorescence imaging of untreated control L6 cells (B), L6 cells + sensor (F) and L6 cells + Al3+ + sensor (J); merge fluorescence imaging + DIC imaging of untreated control L6 cells (C), L6 cells + sensor (G) and L6 cells + Al3+ sensor (K); merge fluorescence imaging of cells for sensors and Hoechst staining for nucleus localisation of untreated control L6 cells (D), L6 cells + sensor (H) and L6 cells + Al3+ + sensor (L). | |
MTT assay for cell viability assessment
For the sensor L, the MTT assay (Mosmann, 1983) has been performed to evaluate the extent of cytotoxicity it could induce in normal cells.36 The cell viability sustains ∼85–90% upon treatment of maximum dose of L (50 μL of stock solution), which indicates that the sensor L does not affect the cell viability to a significant extent, an important criterion for biological application and also supports the intracellular Al3+ ion detection in living cells as well. No significant difference was observed between the percentages of viable cells in the sensor-treated and the solvent-treated lots, indicating thereby that the sensors were non-cytotoxic and safe for biological uses (Fig. S8†).
Conclusions
In summary, a new rhodamine based chemosensor L has been synthesized readily. Metal assisted spirolactam ring opening of the dyes give noticeable change in fluorescence response. Sufficient water solubility, high selectivity, sensitivity, reversibility along with the noticeable color change made this probes suitable chemosensor for the Al3+ ion. The sensor L being non-cytotoxic in nature, and having easy cellular penetrability can be a possible candidate for some practical application as an additional tool for detection of cellular Al3+ ions.
Acknowledgements
This work was supported by Department of Science and Technology, New Delhi, India (SERB/CHM/20130239). PKB thanks SERB for the funding. Financial support from the CSIR, New Delhi to NC and PM are gratefully acknowledged. SBM thanks IIT Kanpur for financial assistance. ARK-B and AS sincerely thank to UGC, New Delhi for awarding an Emeritus Fellowship. The authors are thankful to Department of Zoology, The University of Kalyani.
Notes and references
- K. P. Carter, A. M. Young and A. E. Palmer, Chem. Rev., 2014, 114, 4564–4601 CrossRef CAS PubMed.
- D. T. Quang and J. S. Kim, Chem. Rev., 2010, 110, 6280–6301 CrossRef CAS PubMed.
-
J. P. Desvergne and A. W. Czarnik, Chemosensors of Ion and Molecule Recognition, in NATO Science Series C: Mathematical and Physical Sciences, Kluwer Academic, Dordrecht, London, 1997 Search PubMed.
- S. Kim, J. Y. Noh, K. Y. Kim, J. H. Kim, H. K. Kang, S. W. Nam, S. H. Kim, S. Park, C. Kim and J. Kim, Inorg. Chem., 2012, 51, 3597–3602 CrossRef CAS PubMed.
- D. Maity and T. Govindaraju, Inorg. Chem., 2010, 49, 7229–7231 CrossRef CAS PubMed.
- S. W. King, J. Savory and M. R. Willis, Crit. Rev. Clin. Lab. Sci., 1981, 13, 1–20 CrossRef PubMed.
- I. Shcherbatykh and D. O. Carpenter, J. Alzheimer's Dis., 2007, 11, 191–205 CAS.
- E. Delhaize and P. R. Ryan, Plant Physiol., 1995, 107, 315–321 CrossRef CAS PubMed.
- X. Chen, T. Pradhan, F. Wang, J. S. Kim and J. Yoon, Chem. Rev., 2012, 112, 1910–1956 CrossRef CAS PubMed.
-
J. R. Lakowicz, Principles of Fluorescence Spectroscopy, Springer, New York, 3rd edn, 2006 Search PubMed.
- S. Pal, N. Chatterjee and P. K. Bharadwaj, RSC Adv., 2014, 4, 26585–26620 RSC.
- S. B. Maity and P. K. Bharadwaj, Inorg. Chem., 2013, 52, 1161–1163 CrossRef CAS PubMed.
- I. H. Hwang, Y. W. Choi, K. B. Kim, G. J. Park, J. J. Lee, L. Nguyen, I. Noh and C. Kim, New J. Chem., 2016, 40, 171–178 RSC and references 21–61 therein.
- B. Sen, M. Mukherjee, S. Banerjee, S. Pal and P. Chattopadhyay, Dalton Trans., 2015, 44, 8708–8717 RSC and references therein.
- K. Ghosh, A. Majumdar and T. Sarkar, RSC Adv., 2014, 4, 23428–23432 RSC.
- S. Sahana, S. Bose, S. K. Mukhopadhyay and P. K. Bharadwaj, J. Lumin., 2016, 169, 334–341 CrossRef CAS.
- Y.-W. Wang and Y. Peng, Org. Lett., 2012, 14, 3420–3423 CrossRef PubMed.
- S. Gui, Y. Huang, F. Hu, Y. Jin, G. Zhang, L. Yan, D. Zhang and R. Zhao, Anal. Chem., 2015, 87, 1470–1474 CrossRef CAS PubMed and references therein.
-
L. Di and E. Kerns, Drug-Like Properties: Concepts, Structure Design and Methods from ADME to Toxicity Optimization, Elsevier Science, London, 2nd edn, 2015, pp. 61–90 Search PubMed.
- A. Samadder, S. Das, J. Das and A. R. Khuda-Bukhsh, Colloids Surf., B, 2013, 109, 10–19 CrossRef CAS PubMed.
- S. Saha, M. U. Chhatbar, P. Mahato, L. Praveen, A. K. Siddhanta and A. Das, Chem. Commun., 2012, 48, 1659–1661 RSC.
- K. Ghosh, T. Sarkar and A. Samadder, Org. Biomol. Chem., 2012, 10, 3236–3243 CAS.
- C. Yang, L. Liu, T.-W. Mu and Q.-X. Guo, Anal. Sci., 2000, 16, 537–539 CrossRef CAS.
- J. Bourson, J. Pouget and B. Valeur, J. Phys. Chem., 1993, 97, 4552–4557 CrossRef CAS.
- H. A. Benesi and J. H. Hildebrand, J. Am. Chem. Soc., 1949, 71, 2703–2707 CrossRef CAS.
- D. Maity and T. Govindaraju, Chem. Commun., 2010, 46, 4499–4501 RSC.
- S. H. Kim, H. S. Choi, J. Kim, S. J. Lee, D. T. Quang and J. S. Kim, Org. Lett., 2010, 12, 560–563 CrossRef CAS PubMed.
-
Guidelines for drinking-water quality, World Health Organization, Health criteria and other supporting information, Geneva, 2nd edn, 1996, vol. 2 Search PubMed.
- W.-H. Ding, D. Wang, X.-J. Zheng, W.-J. Ding, J.-Q. Zheng, W.-H. Mu, W. Cao and L.-P. Jin, Sens. Actuators, B, 2015, 209, 359–367 CrossRef CAS.
- J. Kumar, M. J. Sarma, P. Phukan and D. K. Das, Dalton Trans., 2015, 44, 4576–4581 RSC.
- Y. Fu, X.-J. Jiang, Y.-Y. Zhu, B.-J. Zhou, S.-Q. Zang, M.-S. Tang, H.-Y. Zhang and T. C. W. Mak, Dalton Trans., 2014, 43, 12624–12632 RSC.
- M. Shellaiah, Y. H. Wu and H. C. Lin, Analyst, 2013, 138, 2931–2942 RSC.
- V. P. Singh, K. Tiwari, M. Mishra, N. Srivastava and S. Saha, Sens. Actuators, B, 2013, 82, 546–554 CrossRef.
- Y.-Y. Guo, L.-Z. Yang, J.-X. Ru, X. Yao, J. Wu, W. Dou, W.-W. Qin, G.-L. Zhang, X.-L. Tang and W.-S. Liu, Dyes Pigm., 2013, 99, 693–698 CrossRef CAS.
- X. Peng, J. Du, J. Fan, J. Wang, Y. Wu, J. Zhao, S. Sun and X. Xu, J. Am. Chem. Soc., 2007, 129, 1500–1501 CrossRef CAS PubMed.
- T. Mosmann, J. Immunol. Methods, 1983, 65, 55–63 CrossRef CAS PubMed.
Footnote |
† Electronic supplementary information (ESI) available: Materials and physical methods, general procedures, tables, schemes, figures, characterization data, and some spectra. CCDC 1421529. For ESI and crystallographic data in CIF or other electronic format see DOI: 10.1039/c5ra23968k |
|
This journal is © The Royal Society of Chemistry 2016 |
Click here to see how this site uses Cookies. View our privacy policy here.