DOI:
10.1039/C5RA23875G
(Paper)
RSC Adv., 2016,
6, 7701-7711
Novel chitosan-based nanobiohybrid membranes for wound dressing applications
Received
12th November 2015
, Accepted 6th January 2016
First published on 11th January 2016
Abstract
The aim of the present work is the design and characterization of novel nanostructured biopolymer films with improved features for wound dressing applications. Nanohybrid films based on chitosan and biofunctionalized montmorillonite (MMT) with chitosan sulfate chains (SMMT), as the macromolecular intercalant, were fabricated by a solvent casting method. X-ray diffraction analysis confirmed the exfoliated microstructure of the films. The presented results reveal that incorporation of SMMT into a chitosan matrix not only improves the physico-mechanical properties of films such as tensile strength, Young's modulus and thermal stability, but also decreases their moisture vapor transmission rate. The improvement in properties was found to be more pronounced for nanohybrid systems compared with the corresponding chitosan/MMT nanocomposite films. The superior characteristics of the designed chitosan/SMMT nanohybrid films was discussed in terms of the formation of electrostatic interactions at nanointerfaces based on thermogravimetry, dynamic mechanical thermal analysis and atomic force microscopy techniques. The moisture vapor transmission rate of films was measured to be in the range of 690–860 g per m2 per day, which makes them appropriate candidates as wound dressing systems for low and moderate exudates. The fabricated films were also evaluated for their biological features including in vitro cytotoxicity and antibacterial activity. It was disclosed that films are cytocompatible, and chitosan/SMMT nanohybrid films show significant bacteriostatic activity against Gram negative Escherichia coli.
Introduction
Chitosan is a linear polysaccharide and the major derivative of chitin, the second most plentiful natural biopolymer after cellulose.1 Chitosan offers many desirable features like biocompatibility, biodegradability, hemostasis and antibacterial activity against various types of bacteria, which have made it a unique biopolymer for various biomedical applications such as drug delivery and tissue engineering.2–5 Intrinsic antibacterial and wound healing properties of chitosan make it especially useful for wound dressing applications.6 An ideal wound dressing should be biocompatible, provide a moist environment, and absorb exudates, as well as being hemostatic and antimicrobial. Considering exudates discharge and injury location, each type of wound requires a specific dressing; hence, the physicochemical and mechanical properties of the dressing should be adjusted.7 Film-forming ability is another especial aspect of chitosan in comparison with other biopolymers.8 The film features depend on several parameters like chitosan molecular weight, degree of deacetylation, and also the type of acid used to dissolve the biopolymer.9
Polymeric networks consisting of chitosan are produced through crosslinking of its chains with a wide spectrum of crosslinking reagents, like glutaraldehyde, tripolyphosphate, ethylene glycol, diglycidyl ether, diisocyanate, genipin, etc. Most of the synthetic crosslinking reagents cause cytotoxic side effects. Therefore, a crosslinking agent with low cytotoxicity, high stability and biocompatibility is quite desirable.10 Genipin, as a natural material, creates chemically crosslinked chitosan networks through reaction with amino groups.11 Swelling of crosslinked chitosan hydrogel membranes in biological fluids promise their application as wound dressings. Various chemical and physical approaches like chemical modification, blending and nanocomposites have been studied to improve properties of the chitosan biopolymer for wound dressing applications.12–14
Due to their high aspect ratios (100–1500), high surface area (700–800 m2 g−1),15 and exfoliation in water to form single anionic platelets (length to diameter, L/D ≈ 200),16 nanoclay particles have received significant attention for diverse applications. It has been shown introducing nanoclays into a polymer matrix can significantly improve the thermal stability and mechanical properties of the nanocomposites. Hence, many efforts have been dedicated to achieve exfoliation of layered silicate nanoparticle in polymeric matrices. Polymer/clay nanocomposites offer many applications as biomaterials such as nanostructured hydrogels, scaffolds, drug delivery systems and wound dressing membranes.17–19
Taking the advantages of chitosan and its functional groups to interact with nanoclay, this research aims to develop nanostructured hydrogel membranes with improved physicomechanical properties for wound dressing applications. Here we describe treatment of montmorillonite (MMT) with chemically modified chitosan sulfate chains, as a macromolecular intercalating agent, to obtain bio-functionalized nanoclay (SMMT). Considering electrostatic interactions between chitosan sulfate in Na+-MMT gallery spaces with chitosan chains in matrix offer improved nanointerfaces to achieve nanohybrid films with superior features.20–22 To the best of our knowledge, there is no report in the literature to design and characterize nanohybrid biocomposite materials for potential wound dressing applications.
Experimental
Materials
Chitosan (C3646, 85% DD, Mw = 8.1 × 105) was purchased from Sigma-Aldrich USA. Genipin was obtained from Wako Chemicals USA, Inc. Montmorillonite nanoclay (MMT) was acquired from Southern Clay Products. Acetic acid, phthalic anhydride, N,N-dimethylformamide, dimethylacetamide, pyridine, chlorosulfonic acid, hydrazine, methanol and acetone were all supplied by Merck. Cell culture study performed using RPMI-1640, (3-(4,5-dimethylthiazol-2-yl)-2,5 diphenyltetrazolium bromide) (MTT) purchased from Sigma-Aldrich, and penicillin–streptomycin (10
000 IU to 10
000 μg ml−1), 0.25% trypsin–EDTA, fetal bovine serum (FBS) and phosphate buffer saline (PBS) were purchased from GIBCO. Other reagents in cell study were of analytical or cell culture grade. Two types of bacteria, Gram positive and negative were used with commercial code of ATCC 25923 and ATCC 25922, respectively. To assess antibacterial activity Mueller-Hinton culturing media used in microbial studies purchased from Merck. All other reagents were of analytical grade and used without further purification. Deionized water was used in this study.
Synthesis of chitosan sulfate
Synthesis of chitosan sulfate was performed through novel 3 step reactions as follows: phthaloylation of chitosan chains (protection of amino groups), synthesis of chitosan sulfate derivative, and deprotection of amino groups on N-phthaloyl-chitosan sulfate chains.
Phthaloylated chitosan was obtained according to the procedure reported by K. Kurita et al.23 In brief, for phthaloylation reaction 2 g of chitosan was treated with 5.6 g phthalic anhydride in 40 ml of DMF/water (95
:
5) at 120 °C for 8 h. Afterwards, the mixture was poured into ice water, and the precipitation was collected through filtration. It was then thoroughly washed with methanol and dried to give a phthaloylated derivative as a powdery product.
In the next step, 8 g phthaloylated chitosan powder added to 200 ml dimethylacetamide (DMAC) and stirred, heated up to 80 °C and 3 g dried lithium chloride added to mixture. Then, reaction temperature reduced to −5 °C in an ice/water/sodium chloride bath. 20 ml pyridine added to balloon, then 6 ml chlorosulfonic acid added dropwise, and reaction continued for 5 h. Finally, the reaction product was washed by acetone, washed again with ethanol, and dried at room temperature.
In order to achieve chitosan sulfate, deprotection of N-phthaloyl-derivative was performed as described elsewhere.23 In brief, a mixture of 100 mg of N-phthaloyl chitosan derivative in 10 ml of hydrazine monohydrate was stirred at 80 °C for 16 h in nitrogen and poured into 250 ml of ice water. The product was collected, washed with ethanol in a Soxhlet extractor for 3 h and with methanol at room temperature for 1 h, and dried.
Biofunctionalization of montmorillonite
Modification of montmorillonite (MMT) with chitosan was carried out according to a previously reported method.21,22 In brief, 1 g of MMT was dispersed in 50 ml of deionized water and 1 g chitosan was separately dissolved in 1% (v/v) acetic acid aqueous solution. The prepared mixtures were then poured in an Erlenmeyer flask and stirred at 60 °C for 48 h. After the heating treatment, the mixture was centrifuged, and washed with acetic acid solution and water. The product was dried in an air circulating oven at 60 °C for 6 h. It was ground and kept in glass capped bottle for further usage. The biofunctionalization of MMT with chitosan sulfate chains was also performed according to a similar procedure and the product denoted as SMMT. To ensure incorporation of clay (MMT and SMMT) as nanolayers, their suspensions were prepared at concentration of 1% w/v in aqueous media using magnet stirring for a week to obtain well-dispersed exfoliated mixtures, and finally freeze-dried.
Preparation of chitosan-based nanostructured films
Chitosan solution was prepared by dissolving polymer (1% w/w) in an aqueous acetic acid solution (1% w/w) under stirring overnight, then filtered using Büchner funnel to separate undissolved residues. Required amount of nanoparticle was added to 10 ml water and stirred for 30 minutes, then 5 ml chitosan solution was gradually added to mixture. Genipin dissolved in water (1%w/v) and added to the mixture. Finally, the mixture was cast into a Petri dish.
Crosslinking of the films
Cross-linking of chitosan films was carried out using genipin. The concentration of crosslinking agent was 1% w/w over the total content of polymer. After 12 h incubation at room temperature, chitosan films were peeled off from the dish and were further dried in a vacuum oven at 60 °C for 24 hours. In order to remove the residual crosslinking agent, films were immersed in deionized water for 12 h and washed, then dried at 60 °C. The schematic representation of crosslinking reaction between amine groups of chitosan and genipin has been displayed in Scheme 1.10
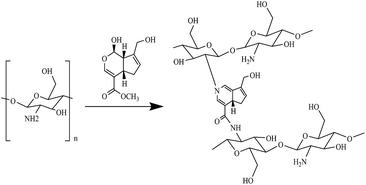 |
| Scheme 1 Schematic representation of crosslinking reaction between amine groups of chitosan and genipin. | |
Fourier transform infrared spectroscopy (FTIR)
The chitosan sulfate derivative was characterized using Fourier transform infrared (FTIR) spectroscopy. FTIR analysis was performed by means of a FTIR spectrometer (Bomem, RG1100G) with a resolution of 20 scans cm−1, in transmission mode. The specimens prepared using the powder samples carefully mixed with KBr (infrared grade) and palletized. Samples were analyzed in the wavelength range of 400–4000 cm−1.
X-ray diffraction analysis
The X-ray diffraction (XRD) patterns of pristine and modified MMT and composite films were obtained using INEL (EQUINOX 3000, France) diffractometer with Cu Kα radiation (λ = 1.541874 Å) at room temperature, operating at a voltage of 40 kV and current of 30 mA. The measurements were performed at a scan rate of 6 min−1.
Dynamic mechanical thermal analysis
Dynamic mechanical thermal analysis (DMTA) of fabricated chitosan-based films was performed on tension mode using a Triton, Tritec 2000DMA instrument at a heating rate of 5 °C min−1 and frequency of 1 Hz scanning over the temperature range from 40 to 220 °C. The dynamic storage modulus (E′), loss modulus (E′′), and loss tangent (tan
δ = E′′/E′) were obtained. The specimens were incubated in vacuum oven at 60 °C for 24 h before tests.
Thermogravimetric analysis
The thermal properties of pristine and nanohybrid chitosan-based films were investigated by thermogravimetric analysis (TGA) and differential thermogravimetric analysis (DTG) carried out by means of a Mettler Toledo (STARe SW) system. All analyses were performed with a 12 mg sample in aluminum pans under nitrogen atmosphere over the temperature range of 30–600 °C. The experiments were run at a scanning rate of 10 K min−1.
Tensile tests
The mechanical properties of the plain and nanohybrid chitosan films were determined with a tensile strength instrument, GALDABINI (Sun 2500). The prepared films were cut into 50 mm length and 10 mm width, and incubated in a vacuum oven at 60 °C overnight. All mechanical tests were performed in triplicates. The measurements were carried out at a stretching rate of 1 mm s−1, and the length between the two grips was set at 10 mm to determine Young's modulus, tensile strength, and elongation at break (εb) for each specimen.
Water uptake
Samples were uniformly cut into 1 × 1 cm2 and dried in a vacuum oven at T = 35 °C for 4 h and weighted (W0). Afterward, samples were immersed in an excess amount of PBS (pH = 7.4) as well as DI water at room temperature. Swollen samples were then taken out carefully at 1 h time intervals; the surface water was carefully removed using a piece of filter paper and samples were weighed (Wt). This procedure was repeated to reach a constant weight. The water uptake (WU%) of samples in PBS and water was calculated using eqn (1),24 |
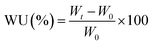 | (1) |
Moisture vapor transmission rate (MVTR)
Moisture vapor transmission rate (MVTR) through the films was measured according to the following procedure using a homemade instrument. A filled glass bottle with 30 g deionized water was fully capped by the prepared films (60 mm diameter in exchange area) and then fastened. The assembly was put in a desiccator, containing anhydrous silica gel with relative humidity <5% at T = 32 ± 1 °C. Evaporation of water through the test samples was tracked by measuring weight loss of the assembly. So, it weighed 8 times at time intervals of 1 h. Weight variations of the bottles were plotted versus time. MVTR was calculated from the slope of this plot obtained by fitting a linear function according to eqn (2) as below,12 |
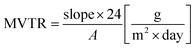 | (2) |
where A is the vapor exchange area under test. MVTR experiments were repeated thrice for each membrane.
Wettability and surface tension
The static mode contact angle and surface energy of chitosan and different nanocomposite films were characterized using a contact angle goniometer. A drop of two different standard liquids, deionized water (γT = 72.8 mN m−1, γd = 21.8 mN m−1, γp = 51.0 mN m−1) and diiodomethane (γT = 50.8 mN m−1, γd = 50.8 mN m−1, γp = 0 mN m−1),25 was separately placed onto the surface of the films. At least three measurements were carried out for each sample. The surface free energy was calculated using Kaelble's eqn (3),12 |
 | (3) |
where θ is the contact angle between film and liquid drop, L is surface tension of the liquid (water or diiodomethane), and d, p, S, L are the dispersive and polar components, solid and liquid, respectively.
Surface morphology analysis and surface modulus pattern
To evaluate the surface morphology and modulus in order to provide a better understanding of superficial features, atomic force microscopy method (AFM, Ambios Technologies USPM instrument) was performed. A silicon cantilever at a resonant frequency of 180 kHz was used. The scan size of films was 40 × 40 μm2 in tapping mode at room temperature. Average roughness of the membranes was measured with scanning areas of 40 × 40 μm2 in 5 different strait lines. A Digital Instruments multimode atomic force microscope (AFM) in tapping mode was used to produce topography images.
Cell culture
Cell culture assays were done using L929 Cell Line from mouse according to the same procedures as reported elsewhere.26 In brief, cells were cultured in flasks with RPMI-1640 supplemented by 10% FBS and 1% penicillin/streptomycin in a humidified incubator at 37 °C with 5% CO2 and 95% air. Having reached the confluence of cells to 80%, cells were harvested from flasks using 0.25 trypsin–EDTA, then re-suspended, counted and seeded onto the films which were cut into a square shape with dimensions of 10 × 10 mm2 and sterilized using UV radiation for 2 h on each side in advance. 50 μl cell suspension containing approximately 25
000 cells were seeded drop-wise on the specimens. Cell-seeded samples were incubated for 3 h at 37 °C allowing the cells to adhere. Afterward, 200 μl of culture medium added to each well and incubated. The medium was changed every day for 5 days and prepared for further analysis such as mitochondrial function study (MTT assay) and cell morphology using scanning electron microscopy.
Proliferation test
The proliferation of L929 cells on the films was assessed with measurement of 3-(4,5-dimethylthiazol-2-yl)-2,5-diphenyltetrazolium bromide (MTT) formazan crystals by viable cell mitochondrial dehydrogenases using the optical density at 570 nm measured by an microplate reader (BioTek). The cell density was adjusted to 2 × 104 cells per ml in RPMI medium containing 10% FBS. 1 ml of cell suspension was added to each well of the 24-well tissue culture plates containing specimens and the cells were allowed to proliferate on the membranes for 5 days. The experiments were also conducted in serum-free medium. To perform the MTT assay, 500 μl of the culture medium was aspirated and replaced with 500 μl of fresh medium at different culture times (1, 3 and 5 days after cell seeding). Then, MTT solution (5 mg ml−1 in PBS) was added into each well followed by incubation in darkness at 37 °C for 4 h. Thereafter, the MTT solution was removed and dimethyl sulfoxide (DMSO) was used to dissolve the formazan crystals. Optical density (OD) of solutions was then measured at 570 nm.
Antibacterial activity assay
To assess the antibacterial activity of films, their effect on E. coli ATCC 25922 and S. aureus ATCC 25923 were studied. Mueller-Hinton broth (MHB) and Mueller-Hinton agar (MHA) were used as culturing media. Colony counting method was performed to evaluate the antibacterial activities of specimens.27 According to the dynamic shake flask method, to claim antibacterial activity of a material at least one log reduction of bacteria load is required.28 To this end, E. coli and S. aureus were cultured overnight, then a standard suspension of bacteria with turbidity of 0.5 McFarland number was prepared; afterward, 100 μl of the suspension added to 1900 μl deionized water to obtain 5% of the primary concentration. Besides control media containing 9 cm3 of MHB and 1 cm3 of the prepared concentration of bacteria suspension 5 mg of each film were put in falcons and placed in an incubator at 37 °C and 100 rpm for 24 hours. To obtain different dilution of bacterial suspension for each sample providing countable colony, finally six serial dilution were performed, and then 10 μl (10 λ) poured on MHA and spread on it using a pipet Pasteur; after all these steps, the plates incubated at 37 °C for 24 hours to calculate the number of bacterial colonies.
Statistical analysis
All results are reported as means ± standard deviations. Statistical comparisons were made by analysis of variance (ANOVA). In all statistical evaluations, p < 0.05 was considered as statistically significant, besides to determine difference significance the Tukey (α = 0.05, 95% confidence intervals) was used between specific means.
Result and discussion
Synthesis and characterization of chitosan sulfate
Taking into account the potential of chitosan chains to enter gallery space of montmorillonite (MMT),20–22 novel hybrid nanostructured chitosan films comprising bio-functionalized MMT with chitosan sulfate (SMMT) were designed to improve molecular interactions at nanointerfaces among the chitosan sulfate bio-modified silicate nanolayers with matrix chitosan chains, hence achieve superior physicomechanical properties. Considering the essential role of amino groups to interact with MMT layers, chitosan chains were chemically modified through a three-step procedure to substitute O-sulfate groups, while retaining amino groups. In order to protect amino groups and provide controlled chemical modification, phthaloylation of chitosan chains was carried out using phthalic anhydride;23 subsequently, substitution of O-sulfate functional groups on phthaloylated chitosan was performed and finally deprotection of N-phthaloyl groups was conducted. Reaction pathway for synthesis of chitosan sulfate is shown in Scheme 2.
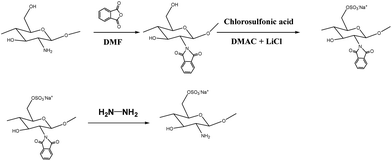 |
| Scheme 2 Reaction pathway for synthesis of chitosan sulfate. | |
The FT-IR spectrum of chitosan has been compared with phthaloylated chitosan and chitosan sulfate Fig. 1. As shown, the common band of 3100–3500 cm−1 is assigned to N–H and O–H stretching vibration, and intermolecular hydrogen bonding of chitosan backbone. Synthesis of N-phthaloyl chitosan is confirmed by the twin particularly sharp peaks at 1776 and 1712 cm−1 (ref. 29) attributed to imide (C
O) and a peak at 721 cm−1 related to the aromatic groups. In the case of O-sulfate chitosan, in the lower wavenumber regions of 1210 and 800 cm−1, it is noticed that the band shape is clearly different from the intact chitosan. This could be attributed to the formation of O-sulfate groups. The characteristic peak at 1220 cm−1 is assigned to the S–O vibration band from sulfate groups.30 The peak around 800 cm−1 confirms synthesis of O-sulfate chitosan, which is related to C–O–S.31 The presence of band at 1171 cm−1 related to C–O–C vibrations in the phthaloylated chitosan and chitosan sulfate spectrum indicate the retaining the main chitosan backbone in the derivatives.30
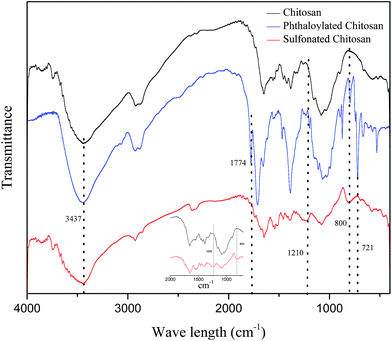 |
| Fig. 1 FT-IR spectrum of chitosan, phthaloylated chitosan and chitosan sulfate. | |
X-ray diffraction analysis
In the next step, MMT layers were treated with the synthesized chitosan sulfate chains. In this process, the cation exchange ability of nanoclay in aqueous media provides replacement of Na+ ions with amino groups of chitosan derivative.22 The obtained bio-functionalized MMT with chitosan sulfate chains, SMMT, was characterized using XRD technique. XRD allows monitoring the interlayer spacing of the clay nanolayers and also provides observation of intercalated or exfoliated microstructure of the resultant nanocomposites. As shown in Fig. 2, d001 for the neat MMT-Na+ was obtained as 11.69 Å, calculated using Bragg's equation nλ = 2d
sin
θ. The d001 for the functionalized SMMT was found to be 13.0491 Å, which confirms the entrance of chitosan sulfate chains into the gallery space of silicate layers through cation exchange and peak position shifts to smaller diffraction angles.20 A schematic representation for the SMMT and corresponding chitosan based nanohybrid structures is displayed in Scheme 3.
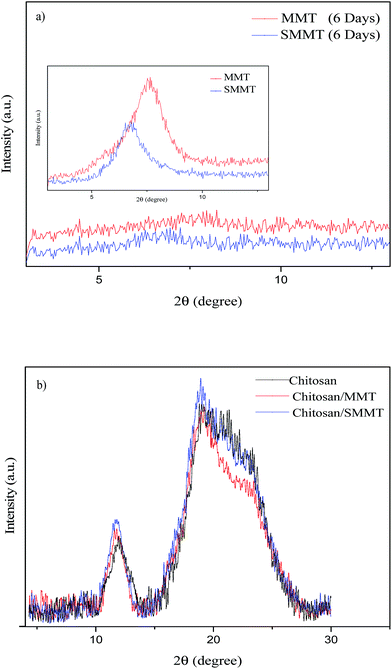 |
| Fig. 2 (a) XRD diffractogram of bio-functionalized montmorillonite with chitosan sulfate chains (SMMT) compared with intact montmorillonite (MMT) for freeze-dried MMT and SMMT after 6 days stirring in aqueous media at 50 °C. The inset shows corresponding diffractogram of MMT and SMMT before dispersion in the media. (b) XRD diffractograms of chitosan, chitosan/MMT, chitosan/SMMT membranes. | |
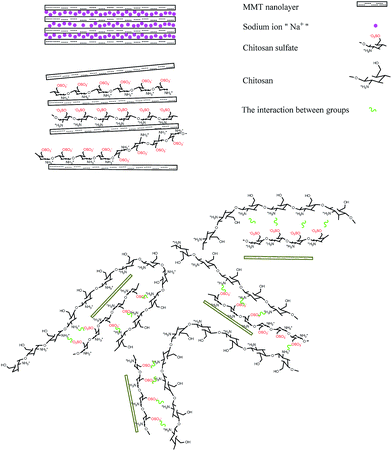 |
| Scheme 3 Representation of bio-functionalization of MMT with chitosan sulfate chains (SMMT) and microstructure of the chitosan/SMMT nanohybrid membranes. | |
In order to fabricate chitosan based nanostructured films comprising either MMT or SMMT, nanoclays were first fully dispersed in aqueous media. Indeed, in this way we assure that the difference in properties of films is solely attributed to the proposed effect of organic/inorganic nanointerfaces. As can be seen in Fig. 2a, the characteristic peak of MMT and SMMT disappeared after stirring in deionized water at 50 °C for 6 days, according to a procedure reported before for exfoliation of Cloisite Na+.32 The microstructure of the chitosan/MMT nanocomposite films as well as chitosan/SMMT nanohybrid membranes were also studied using XRD technique. As can be seen in Fig. 2b, pristine chitosan shows two peaks, one at 11.7° and a stronger peak at 18.9°, which are attributed to crystalline regions of chitosan.33 The peak occurred around 10° imputed to the hydrated polymorphs of bound water with chitosan lattice, which was observed even for freeze-dried chitosan film;34 however, the latter is related to anhydrous crystals in the chitosan structure.35
Dynamic mechanical thermal analysis
Fig. 3 demonstrates elastic modulus (E′) and damping factor (tan
δ) variations for chitosan/MMT and chitosan/SMMT composite films as a function of temperature at 1 Hz compared with pristine chitosan film. E′ is commonly considered as a measure of rigidity of the material. As it is seen in Fig. 3, storage modulus of films decreases with increasing temperature. tan
δ, as an index of material viscoelasticity, is defined as the ratio of energy dissipated to the maximum energy stored in the material.36 It is worthy of note the controversy in the literature on the glass transition temperature (Tg) for chitosan since various characteristics such as crystallinity, molecular weight, degree of deacetylation as well as source and method of extraction affect the Tg of chitosan.36–38
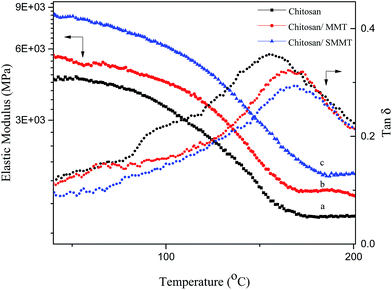 |
| Fig. 3 Elastic modulus and tan δ curves as a function of temperature for (a) chitosan, (b) chitosan/MMT, and (c) chitosan/SMMT membranes. | |
Pristine bulk chitosan exhibits a strong β-relaxation peak about 156 °C, a signature of glass transition. Introducing MMT nanoparticles not only shows a reduction in damping factor, but also causes the temperature of the β-relaxation peak to be shifted to higher temperatures (165 °C). It should be noticed that owing to improved interfacial interactions between chitosan matrix and modified SMMT nanolayers, the damping factor peak shifts to a higher temperature (169 °C). In other words, such increase in Tg originates from confinement of polymer chains in the vicinity of nanoparticles.39 Incorporation of MMT and SMMT nanosheets in chitosan matrix have no effect on damping pattern of composite film, but shift of the peak. It is also worthy of note that diminished tan
δ peak after introducing nanoclay to chitosan film is in good agreement with the previously reported observations,40,41 which implies nanoparticles have well distributed in the composite films.
Thermogravimetric analysis
Thermogravimetric analysis (TGA) curves for chitosan and composites films are shown in Fig. 4. The first step (50–170 °C) about 10 wt% weight loss is associated with the loss of absorbed and bound water as well as residue of acetic acid,42 while the second step (200–400 °C) corresponds to the degradation and deacetylation of chitosan and left about 40 wt% solid residue.43 Such observation is consistent with the results reported in other studies.44,45 As can be seen, the decomposition rate of the composite films was slightly decreased. The improved thermal stability of composite films can be attributed to the interaction between the silicate nanolayers and the matrix chitosan chains,46–48 which was found to be more pronounced in the case of chitosan/SMMT films. This is associated with the improved organic/inorganic interfacial interactions in the nanohybrid membrane.
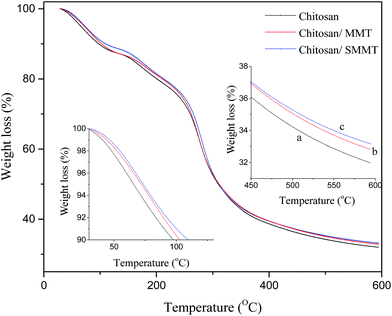 |
| Fig. 4 Thermogravimetric analysis curves for (a) chitosan, (b) chitosan/MMT, and (c) chitosan/SMMT films. | |
Tensile tests
The fabricated chitosan, chitosan/MMT, and chitosan/SMMT films were characterized for their mechanical properties at room temperature and obtained results are summerized in Table 1. As can be seen, nanocomposite films show higher tensile strength and Young's modulus compared to the plain chitosan membrane. Such observation is typically attributed to the reinforcing effect of nanoparticles due to restriction of polymer chains and possibility of stress transfer from soft matrix to the load bearing well-dispersed inorganic nanophase as confirmed by the XRD results.13 Accordingly, the superior tensile strength and Young's modulus for the chitosan/SMMT nanohybrid membrane compared to chitosan/MMT nanocomposite film is attributed to higher strength of the organic/inorganic nanointerfaces due to improved electrostatic interactions in the presence of chitosan sulfate chains.49,50 Despite the addition of MMT and SMMT improved Young's modulus and tensile strength, it caused a decrease in the elongation at break. Such observation originates from the hindered dynamic of chitosan chains for deformation in the vicinity of clay nanosheets,51 which is more pronounced in the case of SMMT.
Table 1 Tensile strength, Young's modulus, and elongation at break for chitosan, chitosan/MMT, and chitosan/SMMT films. Data are presented as mean ± SD
Specimen |
Tensile strength (MPa) |
Young's modulus (MPa) |
Elongation at break (%) |
Chitosan |
16.34 ± 1.23 |
547.64 ± 53.45 |
22.94 ± 1.17 |
Chitosan/MMT |
18.15 ± 0.54 |
1033.45 ± 38.93 |
16.34 ± 0.69 |
Chitosan/SMMT |
19.39 ± 0.34 |
1178.33 ± 43.51 |
14.87 ± 0.73 |
Wettability and surface tension
Surface wettability is one of the critical properties of films in biomedical applications like wound dressing.12 To determine the polar and dispersive components of surface tension, frequently Kaelble's equation (Table 2) is used.12 This equation calculates the surface tensions of polymeric materials based on contact angle measurement of two liquids on the polymer surface.52 Wettability results of films in terms of contact angle (water and diiodomethane) and surface tension components have been summarized in Table 2. The obtained results revealed that incorporation of clay nanoparticles (MMT and SMMT) in chitosan matrix increase the contact angle. This finding could be explained by the hydrophilic nature of MMT as well as surface roughness induced by nanoparticles which will be discussed in the atomic force microscopy section. The lower contact angle of chitosan/SMMT film compared with chitosan/MMT could be attributed to its surface topography formed because of intensified electrostatic interactions providing for more air pockets to be trapped on the surface and contact angle decrease as a consequence.12 The trends for diiodomethane contact angle could also be explained accordingly.
Table 2 Contact angle and surface tension of chitosan, chitosan/MMT, and chitosan/SMMT filmsa
Specimen |
Contact angle (°) |
Surface tension (mN m−1) |
Water |
DIM |
γTotal |
γp |
γd |
Note: DIM: diiodomethane; γd: surface free energy (dispersive); γp: surface free energy (polar); γTotal: total surface free energy. |
Chitosan |
98.02 |
58.2 |
30 |
0.38 |
29.62 |
Chitosan/MMT |
114.35 |
53.9 |
32.41 |
0.33 |
32.07 |
Chitosan/SMMT |
109.01 |
57.2 |
29.1 |
0.35 |
28.75 |
With respect to the total surface free energy, the changes in wettability property did not change significantly.25 However, with addition of MMT the dispersive component raised and with introducing SMMT to chitosan matrix the polar component in comparison to MMT increased. This could be because of polar functional groups (chitosan sulfate) on surface of the clay nanosheets.
Water uptake
One of the most important aspects of a membrane to be considered as a wound dressing is swelling and water uptake behavior, which determines its capacity to absorb exudates.53 The equilibrium water uptake (WU) results for prepared films at 25 °C have been summarized in Table 3. It should be noted that all films were swollen in water and reached equilibrium in 48 h. Data show that the incorporation of nanoparticles significantly reduces the equilibrium WU of the chitosan matrix. The inclusion of nanoparticles in the composite films resulted in decreasing equilibrium WU in DI water from 1820% for plain chitosan film to 1621% and 1197% for the MMT and SMMT composite films, respectively. Amino groups on chitosan chains play critical role in swelling of the chitosan-based networks. In this respect, the reduced WU for the nanocomposite and nanohybrid matrices could be explained by electrostatic interaction and ion exchange of protonated amino groups with nanoclay silicate layers, which prohibit them to be hydrated with water molecules.41 In other words, MMT nanoparticles because of their high surface area and cation exchange capacity absorb H+ in the aqueous media in order to minimize their surface energy which causes the expansion of chitosan networks to be decreased.54–56 In the case of chitosan/SMMT, the immobilized chitosan sulfate chains on the exfoliated clay nanosheets provide tighter networks with relatively lower water uptake capacity. To simulate the physiological condition, water uptake measurements were also performed using PBS solution (pH = 7.4). The obtained results revealed a similar trend but drastic drop in WU for all samples. This could be explained with screening of the ions and charged functional groups present in the polymeric networks by the counter ions in PBS media.
Table 3 Equilibrium water uptake (WU) for chitosan, chitosan/MMT, and chitosan/SMMT films at 25 °C. Data are reported as mean ± SD
Specimens |
WU in DI water (%) |
WU in PBS (%) |
Chitosan |
1820.61 ± 59.6 |
396.16 ± 12.61 |
Chitosan/MMT |
1621.25 ± 43.13 |
370.15 ± 14.42 |
Chitosan/SMMT |
1196.33 ± 35.63 |
341.53 ± 9.84 |
Moisture vapor transmission rate (MVTR)
Controlling the water loss from wounds at an optimal rate is one of the important features of an ideal wound dressing membrane. According to Queen et al., the moisture vapor transmission rate (MVTR) for normal skin is 204 g per m2 per day; however MVTR for injured skin can be increased to 279 g per m2 per day for the first-degree burns and 5138 g per m2 per day for a granulating wound at surface temperature of 35 °C.57 An ideal wound dressing not only should prevent excessive dehydration, but also be able to build up the exudate. As depicted in Fig. 5, the MVTR of the films are in the range of 690–860 g per m2 per day. As it was expected the MVTR of chitosan membrane decreased after introducing nanoparticles. Chitosan/SMMT nanohybrid membranes showed the lowest MVTR, which is well consistent with its tighter microstructure as discussed for water uptake data. In fact, chitosan sulfate chains in the interface of clay nanosheets and chitosan matrix provides higher interactions responsible for improved dispersion and obstructed interfacial voids for water vapor diffusion. The obtained WVTR results of the fabricated membranes promise their capability to be utilized for wounds with low to moderate exudate levels.58
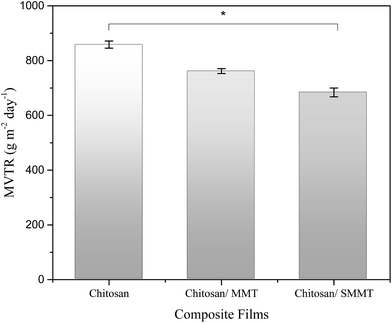 |
| Fig. 5 Moisture vapor transmission rate (MVTR) of chitosan, chitosan/MMT, and chitosan/SMMT membranes at T = 32 ± 1 °C, bar graphs show *p < 0.05. | |
Atomic force microscopy
The fabricated chitosan-based films were characterized for their high-resolution topography with atomic force microscopy (AFM) in the tapping mode. The surface morphology images for different samples are shown in Fig. 6 and the roughness average (Ra) is collected in Table 4.
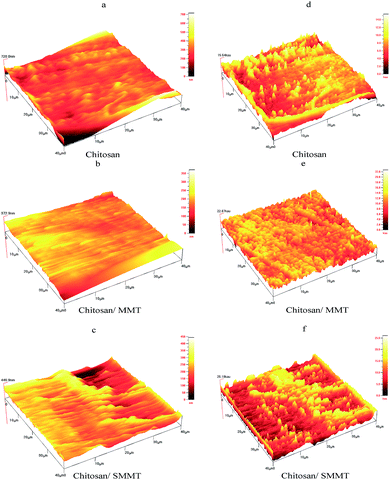 |
| Fig. 6 Atomic force micrographs of (a) chitosan, (b) chitosan/MMT, and (c) chitosan/SMMT films. Surface modulus patterns of (d) chitosan, (e) chitosan/MMT, and (f) chitosan/SMMT films. | |
Table 4 Average roughness (Ra) of chitosan, chitosan/MMT, and chitosan/SMMT films obtained from atomic force microscopy, data are presented as mean ± SD
Sample |
Chitosan |
Chitosan/MMT |
Chitosan/SMMT |
Ra (nm) |
13.3 ± 2.3 |
42.3 ± 0.8 |
45 ± 1.1 |
As can be seen, the pristine chitosan film showed smoother surface with uniform and well distributed shorter spikes compared with chitosan/MMT, and chitosan/SMMT membranes. It has been reported the surface morphology of chitosan-based films is typically rough, which may be because of the crystalline nature of this polysaccharide.42 In the case of chitosan/MMT and chitosan/SMMT composite membranes, it was found that inclusion of nanoparticles results in a significant increment in Ra. Relatively higher roughness of the chitosan/SMMT nanohybrid membranes could be explained in view of the electrostatic complexation between interfacial chitosan sulfate chains on silicate nanolayers and surrounding chitosan chains, which resulted in formation of secondary nanodomains on the surface. In fact, by taking into account the concurrent physical and chemical interplay of SMMT affecting the superficial features of the nanohybrid films, it could be inferred that the chitosan sulfate chains on the MMT nanolayers has dominated the physical features and roughness.12 It is worthy of note that such interpretation is well consistent with the above discussion regarding surface tension and wettability results.
The films were also characterized for surface modulus using AFM. The modulus patterns in tapping mode are collected in Fig. 6. As expected, the composite membranes showed higher surface modulus. It was found that the inclusion of SMMT has more significant influence on modulus improvement compared with MMT, which confirms the effect of modification of nanoparticles to form nanohybrid domains on the surface.
Cell viability study
MTT assay was performed to study viability of L929 fibroblast cells on membranes (Fig. 7). According to the statistical analysis, the cell viabilities of the films do not show significant difference (p < 0.05), which confirms the nanostructured films are not only cytocompatible, but also support growth and proliferation of fibroblasts.
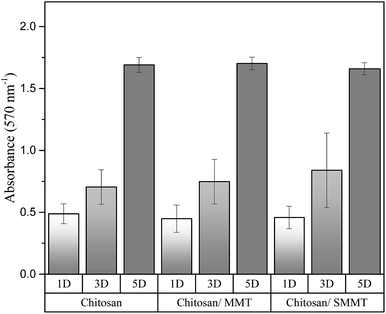 |
| Fig. 7 Viability of L929 fibroblast cells on chitosan, chitosan/MMT, and chitosan/SMMT films based on MTT assay. | |
Scanning electron micrographs of cell morphology
Morphology of cells on films on day 3 was observed by scanning electron microscopy (SEM). As depicted in Fig. 8, cell adhesion is well supported on all membranes as the cells spread on the surface. As can be seen, the micrographs shows morphology of cells on chitosan/SMMT to be more stretched, which could be associated with the surface roughness.
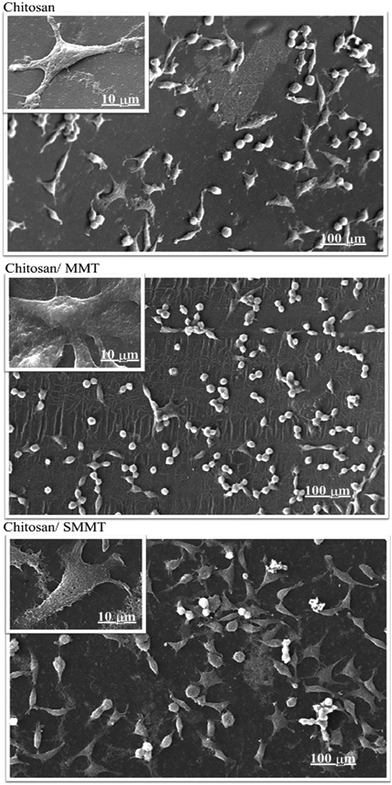 |
| Fig. 8 Scanning electron micrographs of L929 cells cultured on chitosan, chitosan/MMT, and chitosan/SMMT films after three days. | |
Antibacterial activity assay
The antibacterial activity of films in terms of killing efficiency and population reduction of bacteria are displayed in Fig. 9. Antibacterial activity of films was measured against S. aureus (Gram positive) and E. coli (Gram negative) bacteria. All chitosan films showed high activity toward S. aureus and E. coli bacteria. The plain chitosan film showed significant impact on S. aureus, while chitosan/SMMT were found to be more effective against E. coli. Chitosan/SMMT showed significant (p < 0.05) improvement in antibacterial activity among others. The result of log reduction also confirmed the killing efficiency data and illuminated the fact that MMT itself had not any antibacterial effect in chitosan structure in this method.15,59 The main mechanism of antibacterial activity of chitosan and its derivatives is vague almost universally accepted mechanism is polycationic properties of chitosan helps it to attack the negatively charged bacterial cell wall and cause cell wall leakage, leading to death of bacteria.60
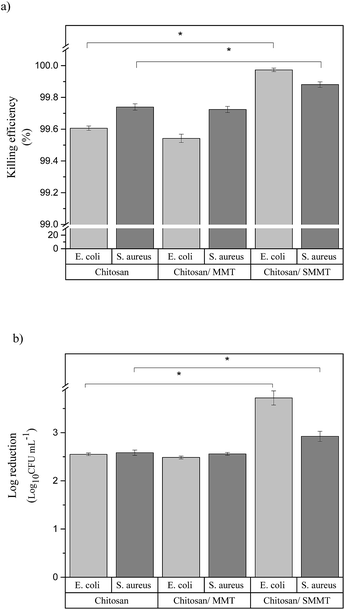 |
| Fig. 9 Killing efficiency of chitosan, chitosan/MMT and chitosan/SMMT substrates toward E. coli and S. aureus in MHB after 24 h, bar graphs show *p < 0.05 (a). Population reduction of the two tested bacteria (log10 CFU ml−1) 24 h exposed to chitosan, chitosan/MMT and chitosan/SMMT membranes in MHB, bar graphs show *p < 0.05 (b). | |
Conclusions
Montmorillonite nanolayers were treated with chitosan sulfate (SMMT), as a functional biocompatibilizer, and exfoliated in chitosan matrix to design nanohybrid membranes for potential wound dressing applications. Chitosan/SMMT showed improved physicochemical properties including higher modulus, wettability, and lower moisture vapor transmission rate than plain and chitosan/MMT nanocomposite films. In vitro biocompatibility studies revealed that membranes did not show any cytotoxicity against L929 fibroblast cells and SEM micrographs showed better cell morphology and attachment on chitosan/SMMT. In vitro antibacterial activity studies proved the claim that chitosan/SMMT had antibacterial activity toward Gram-positive bacteria (S. aureus) than Gram-negative bacteria (E. coli). The presented results converge to promise the potential of nanohybrid membrane as a proper wound dressing for burn, chronic and diabetic wound infections with low to moderate exudate.
Acknowledgements
The authors would like to thank Dr Zahra Jamalpoor from Iran University of Medical Sciences for assistance in cell culture and MTT assay, Dr Ali Hashemi from Shahid Beheshti University of Medical Sciences, Iran, and also Dr Akbar Jokar from Tarbiat Modares University, Food science, Tehran, Iran, for their unstinting help in antibacterial assessment.
References
- M. Rinaudo, Prog. Polym. Sci., 2006, 31, 603–632 CrossRef CAS.
- E. Dashtimoghadam, H. Mirzadeh, F. A. Taromi and B. Nyström, Polymer, 2013, 54, 4972–4979 CrossRef CAS.
- F. S. Majedi, M. M. Hasani-Sadrabadi, J. J. VanDersarl, N. Mokarram, S. Hojjati-Emami, E. Dashtimoghadam, S. Bonakdar, M. A. Shokrgozar, A. Bertsch and P. Renaud, Adv. Funct. Mater., 2014, 24, 432–441 CrossRef CAS.
- M. Aliaghaie, H. Mirzadeh, E. Dashtimoghadam and S. Taranejoo, Soft Matter, 2012, 8, 7128–7137 RSC.
- I.-Y. Kim, S.-J. Seo, H.-S. Moon, M.-K. Yoo, I.-Y. Park, B.-C. Kim and C.-S. Cho, Biotechnol. Adv., 2008, 26, 1–21 CrossRef CAS.
- H. Ueno, T. Mori and T. Fujinaga, Adv. Drug Delivery Rev., 2001, 52, 105–115 CrossRef CAS.
- J. J. Elsner, A. Shefy-Peleg and M. Zilberman, J. Biomed. Mater. Res., Part B, 2010, 93, 425–435 CrossRef.
- M. N. V. R. Kumar, R. A. A. Muzzarelli, C. Muzzarelli, H. Sashiwa and A. J. Domb, Chem. Rev., 2004, 104, 6017–6084 CrossRef.
- D. F. Xie, V. P. Martino, P. Sangwan, C. Way, G. A. Cash, E. Pollet, K. M. Dean, P. J. Halley and L. Avérous, Polymer, 2013, 54, 3654–3662 CrossRef CAS.
- R. A. A. Muzzarelli, Carbohydr. Polym., 2009, 77, 1–9 CrossRef CAS.
- M. F. Butler, Y.-F. Ng and P. D. A. Pudney, J. Polym. Sci., Part A: Polym. Chem., 2003, 41, 3941–3953 CrossRef CAS.
- A. Hashemi Doulabi, H. Mirzadeh, M. Imani and N. Samadi, Carbohydr. Polym., 2013, 92, 48–56 CrossRef CAS.
- T. Wu, R. Farnood, K. O'Kelly and B. Chen, J. Mech. Behav. Biomed. Mater., 2014, 32, 279–286 CrossRef CAS.
- A. M. Abdelgawad, S. M. Hudson and O. J. Rojas, Carbohydr. Polym., 2014, 100, 166–178 CrossRef CAS.
- S.-I. Hong and J.-W. Rhim, J. Nanosci. Nanotechnol., 2008, 8, 5818–5824 CrossRef CAS.
- G. Laufer, C. Kirkland, A. A. Cain and J. C. Grunlan, ACS Appl. Mater. Interfaces, 2012, 4, 1643–1649 CAS.
- C.-W. Chiu, T.-K. Huang, Y.-C. Wang, B. G. Alamani and J.-J. Lin, Prog. Polym. Sci., 2014, 39, 443–485 CrossRef CAS.
- I. Anghel, A. Holban, A. Grumezescu, E. Andronescu, A. Ficai, A. Anghel, M. Maganu, V. Lazǎr and M. Chifiriuc, Nanoscale Res. Lett., 2012, 7, 1–8 CrossRef.
- A. Contin, A. Biffis, S. Sterchele, K. Dörmbach, S. Schipmann and A. Pich, J. Colloid Interface Sci., 2014, 414, 41–45 CrossRef CAS.
- M. M. Hasani-Sadrabadi, E. Dashtimoghadam, F. S. Majedi and K. Kabiri, J. Power Sources, 2009, 190, 318–321 CrossRef CAS.
- M. M. Hasani-Sadrabadi, E. Dashtimoghadam, F. S. Majedi, K. Kabiri, N. Mokarram, M. Solati-Hashjin and H. Moaddel, Chem. Commun., 2010, 46, 6500–6502 RSC.
- M. Darder, M. Colilla and E. Ruiz-Hitzky, Chem. Mater., 2003, 15, 3774–3780 CrossRef CAS.
- K. Kurita, H. Ikeda, M. Shimojoh and J. Yang, Polym. J., 2007, 39, 945–952 CrossRef CAS.
- J. S. Mao, L. G. Zhao, Y. J. Yin and K. D. Yao, Biomaterials, 2003, 24, 1067–1074 CrossRef CAS.
- J. Rotta, R. Á. Ozório, A. M. Kehrwald, G. M. de Oliveira Barra, R. D. de Melo Castanho Amboni and P. L. M. Barreto, Mater. Sci. Eng., C, 2009, 29, 619–623 CrossRef CAS.
- Z. Jamalpoor, H. Mirzadeh, M. T. Joghataei, D. Zeini, S. Bagheri-Khoulenjani and M. R. Nourani, J. Biomed. Mater. Res., Part A, 2015, 103, 1882–1892 CrossRef.
- P. T. Sudheesh Kumar, V.-K. Lakshmanan, T. V. Anilkumar, C. Ramya, P. Reshmi, A. G. Unnikrishnan, S. V. Nair and R. Jayakumar, ACS Appl. Mater. Interfaces, 2012, 4, 2618–2629 CAS.
- R. J. B. Pinto, S. C. M. Fernandes, C. S. R. Freire, P. Sadocco, J. Causio, C. P. Neto and T. Trindade, Carbohydr. Res., 2012, 348, 77–83 CrossRef CAS.
- K. Kurita, H. Ikeda, Y. Yoshida, M. Shimojoh and M. Harata, Biomacromolecules, 2001, 3, 1–4 CrossRef.
- P. H. L. Lima, S. V. A. Pereira, R. B. Rabello, E. Rodriguez-Castellón, M. M. Beppu, P. Chevallier, D. Mantovani and R. S. Vieira, Colloids Surf., B, 2013, 111, 719–725 CrossRef CAS.
- G. Vikhoreva, G. Bannikova, P. Stolbushkina, A. Panov, N. Drozd, V. Makarov, V. Varlamov and L. Gal'braikh, Carbohydr. Polym., 2005, 62, 327–332 CrossRef CAS.
- A. Delville, Langmuir, 1991, 7, 547–555 CrossRef CAS.
- P. Kolhe and R. M. Kannan, Biomacromolecules, 2002, 4, 173–180 CrossRef.
- K. V. Harish Prashanth, F. S. Kittur and R. N. Tharanathan, Carbohydr. Polym., 2002, 50, 27–33 CrossRef CAS.
- K. Ogawa, T. Yui and M. Miya, Biosci., Biotechnol., Biochem., 1992, 56, 858–862 CrossRef CAS.
- A. Hashemi Doulabi, H. Mirzadeh and M. Imani, Mater. Chem. Phys., 2013, 139, 515–524 CrossRef.
- M. Mucha and A. Pawlak, Thermochim. Acta, 2005, 427, 69–76 CrossRef CAS.
- Y. Wan, H. Wu, A. Yu and D. Wen, Biomacromolecules, 2006, 7, 1362–1372 CrossRef CAS.
- K. Kabiri, H. Mirzadeh, M. J. Zohuriaan-Mehr and M. Daliri, Polym. Int., 2009, 58, 1252–1259 CrossRef CAS.
- A. I. Yaich, U. Edlund and A.-C. Albertsson, Carbohydr. Polym., 2014, 100, 135–142 CrossRef CAS.
- M. Lavorgna, I. Attianese, G. G. Buonocore, A. Conte, M. A. Del Nobile, F. Tescione and E. Amendola, Carbohydr. Polym., 2014, 102, 385–392 CrossRef CAS.
- K. Lewandowska, A. Sionkowska, B. Kaczmarek and G. Furtos, Int. J. Biol. Macromol., 2014, 65, 534–541 CrossRef CAS.
- R. Jayakumar and H. Tamura, Int. J. Biol. Macromol., 2008, 43, 32–36 CrossRef CAS.
- S. F. Wang, L. Shen, Y. J. Tong, L. Chen, I. Y. Phang, P. Q. Lim and T. X. Liu, Polym. Degrad. Stab., 2005, 90, 123–131 CrossRef CAS.
- X. Qu, A. Wirsén and A.-C. Albertsson, J. Appl. Polym. Sci., 1999, 74, 3193–3202 CrossRef CAS.
- E. Günister, D. Pestreli, C. H. Ünlü, O. Atıcı and N. Güngör, Carbohydr. Polym., 2007, 67, 358–365 CrossRef.
- J.-H. Chang, Y. U. An and G. S. Sur, J. Polym. Sci., Part B: Polym. Phys., 2003, 41, 94–103 CrossRef CAS.
- K. E. Strawhecker and E. Manias, Chem. Mater., 2000, 12, 2943–2949 CrossRef CAS.
- W. D. Zhang, L. Shen, I. Y. Phang and T. Liu, Macromolecules, 2004, 37, 256–259 CrossRef CAS.
- J. N. Coleman, U. Khan, W. J. Blau and Y. K. Gun'ko, Carbon, 2006, 44, 1624–1652 CrossRef CAS.
- A. Giannakas, K. Grigoriadi, A. Leontiou, N.-M. Barkoula and A. Ladavos, Carbohydr. Polym., 2014, 108, 103–111 CrossRef CAS.
- C. M. Chan, Polymer Surface Modification Characterization, Hanser Gardner Publications, Cincinnati, Ohio, U.S.A, 1994 Search PubMed.
- M. M. Rahman, S. Pervez, B. Nesa and M. A. Khan, Polym. Int., 2013, 62, 79–86 CrossRef CAS.
- W. H. Slabaugh and G. H. Kennedy, J. Colloid Sci., 1963, 18, 337–342 CrossRef CAS.
- S. Sinha Ray and M. Okamoto, Prog. Polym. Sci., 2003, 28, 1539–1641 CrossRef.
- E. P. Giannelis, Adv. Mater., 1996, 8, 29–35 CrossRef CAS.
- D. Queen, J. D. S. Gaylor, J. H. Evans, J. M. Courtney and W. H. Reid, Biomaterials, 1987, 8, 367–371 CrossRef CAS.
- P. Wu, A. C. Fisher, P. P. Foo, D. Queen and J. D. S. Gaylor, Biomaterials, 1995, 16, 171–175 CrossRef CAS PubMed.
- P. Kanmani and J.-W. Rhim, Food Hydrocolloids, 2014, 35, 644–652 CrossRef CAS.
- P. Li, Y. F. Poon, W. Li, H.-Y. Zhu, S. H. Yeap, Y. Cao, X. Qi, C. Zhou, M. Lamrani, R. W. Beuerman, E.-T. Kang, Y. Mu, C. M. Li, M. W. Chang, S. S. Jan Leong and M. B. Chan-Park, Nat. Mater., 2011, 10, 149–156 CrossRef CAS.
|
This journal is © The Royal Society of Chemistry 2016 |