DOI:
10.1039/C5RA23870F
(Paper)
RSC Adv., 2016,
6, 24353-24360
One-step synthesis of bulk Mo and Ni–Mo carbides for methanation
Received
12th November 2015
, Accepted 26th February 2016
First published on 26th February 2016
Abstract
β-Mo2C and Ni/β-Mo2C catalysts were prepared by a single-step thermal decomposition method using hexamethylenetetramine (HMT) as a reducing agent and carbon source. The synthesized carbides were detailedly characterized and their catalytic performances in methanation were evaluated. The results showed that serious carbon deposition was observed in β-Mo2C, while for Ni/β-Mo2C, the carbon content was insufficient and Ni3Mo3N was produced. With respect to catalytic performances in methanation, the β-Mo2C catalyst exhibited a high CO conversion, but its CH4 selectivity was low and its activity was not stable due to hydrogenation of the carbidic carbon. While the Ni/β-Mo2C catalyst exhibited excellent activity and stability, the CO conversion and CH4 selectivity increased from 67.41% and 33.54% on β-Mo2C to 92.51% and 52.73% on Ni/β-Mo2C. This is ascribed to Ni3Mo3N in Ni/β-Mo2C, which is more active and stable during methanation.
1. Introduction
Synthetic natural gas (SNG) produced from coal or biomass has attracted more and more attention due to the limited reserves but rapidly expanding demand for clean natural gas.1,2 Several steps, such as gasification, gas cleaning, conditioning (i.e., water gas shift reaction) and methanation, are required to synthesize SNG from coal. Meanwhile, gas cleaning and conditioning are essential if coal or other sulfur-containing carbon materials are utilized as raw materials, due to their low H/C ratios and high sulfur contents. Thus, low-temperature separation processes (for example, low temperature methanol cleaning process) are usually integrated between the high-temperature gasifier and high-temperature methanizer to remove CO2 and other impurities, which cause further decrease in overall energy efficiency.
Supported Ni-based catalysts have been applied commercially on methanation due to their higher activity and selectivity to methane and lower cost,3,4 but traditional Ni-based catalysts must function at higher H2/CO ratios (i.e., H2/CO = 3/1), moreover, they could be easily deactivated as a result of very small amounts of sulfur compounds,5,6 so conditioning must be involved and the sulfur-bearing compounds must be completely removed before methanation. In this respect, novel catalysts need to be developed to overcome the above mentioned problems.
An ideal multi-functional catalyst, which could catalyze water gas shift reaction and methanation, working at lower H2/CO ratios and keeping higher activity in the presence of sulfur compounds, is expected. The metal carbides and nitrides have been identified as the potential catalysts for such applications, due to theirs sulfur-resistant properties,7–13 great catalytic activities and stabilities in the WGS reaction, and thus may work at lower H2/CO ratios and keep higher activity in the presence of sulfur compounds.14–17 Thus Mo or Ni–Mo-based carbonized catalysts have shown some potential for industrial utilization because of theirs sulfur tolerant properties and high activities for methanation and WGS reactions. However, to the best of our knowledge, few applications of Mo or Ni–Mo-based carbonized catalysts on methanation are found. Thus the performance of β-Mo2C and Ni/β-Mo2C on methanation should be paid more attentions and some detailed work should be done in this field. Therefore, in this paper, the β-Mo2C and Ni/β-Mo2C catalysts were synthesized by a simple but effective way,18–21 i.e. the single-step thermal decomposition method. Unlike other preparation methods, gaseous hydrocarbons with high flow rate and slow heating rate are not required in this simple method. And these advantages could decrease the preparation costs and enlarge its industrial utilization. In addition, their activities on methanation were investigated in terms of CO conversion and CH4 selectivity.
2. Experimental section
2.1. Preparation of the catalysts
2.1.1 Synthesis of β-Mo2C. Commercially available (NH4)6Mo7O24·4H2O and HMT with the molar ratio of 1
:
10 were dissolved in 15% NH3·H2O solution under stirring. Then the solution was evaporated at ambient conditions, until slurry was obtained. The slurry was dried under vacuum at 353 K for 8 h and the resulted solid was crushed to a fine powder. Then the prepared powder precursor was carbonized and passivated (deactivated), and the catalysts were obtained. While the carbonization, i.e. the process of thermal decomposition, could be described as follows: the precursor was placed in a down-flow quartz tube reactor and a flow of N2 (99.99%) was introduced to sweep. Then the reactor was heated to 973 K with a heating rate of 10 K min−1 and held at this temperature for 1.5 h. After that, the samples were naturally cooled to room temperature under N2. The so called passivation process was finished by exposing the thermally treated precursor at room temperature to a flow of 1% (v/v) O2/N2 for 2 h before exposing to air.
2.1.2 Synthesis of Ni/β-Mo2C. Mixed-salt precursor to Ni/β-Mo2C was prepared by dissolving Ni(NO3)2·6H2O, (NH4)6Mo7O24·4H2O and HMT with the mole ratio of 7
:
2
:
20 in 15% NH3·H2O solution under stirring. Then the solution was evaporated to dryness and slurry was obtained. The subsequent steps for the synthesis of Ni/β-Mo2C were carried out as described above for the synthesis of β-Mo2C.
2.2. Catalyst characterization
X-ray powder diffraction (XRD) patterns of the tested catalysts were obtained on a Rigaku D/Max 2500 powder diffractometer using CuKα radiation as the X-ray source, the diffractograms were analysed using the standard JCPDS files. Scanning electron microscopy (SEM) and high-resolution transmission electron microscope (HRTEM) images were obtained using a JSM-7001F SEM (10KV) and JEM-2010 HRTEM respectively. The X-ray photoelectron spectras (XPS) were recorded on an AXIS ULTRA DLD spectrometer using an Al Kα X-ray source and was operated at 14 kV and 20 mA. A TRACOR Northern accumulator was used for data acquisition and for signal averaging. The residual pressure inside the analysis chamber was about 1 × 10−7 Pa. The binding energy BE was obtained by reference to the C 1s line set at 284.8 eV. We checked this line at the beginning and at the end of the measurements. No change in its position was observed. Under our operating conditions, the precision of the BE values was 0.2 eV. The pulsed CO chemisorption and temperature-programmed surface reaction (H2-TPSR) experiments were performed using a Micromeritics AutoChem 2910 Chemisorption Analyzer equipped with thermal conductivity (TCD) and on line mass spectrometer detectors.
2.3. Catalyst performance tests
The performances of the catalysts on methanation were evaluated in a pressured fixed-bed continuous-flow reactor (i.d. = 12 mm) and the loading amounts of catalysts were 4.64 g (2.0 ml) and 3.81 g (2.0 ml) for β-Mo2C and Ni/β-Mo2C respectively (pellets, 60–80 mesh). The gas flow rate of reaction gas was measured by mass flow controller, which was calibrated regularly by a bubble flow meter. The carbon mass balances were generally fulfilled to less than 10%. In order to remove a possible passivated layer on the surface, all catalysts were heated to 673 K with a heating rate of 10 K min−1 and held at 673 K for 2 h in H2 atmosphere prior to the reaction. Then the reducing gas was switched to syngas. Catalytic activity measurements were carried out under the reaction conditions of P = 3.0 MPa, T = 773 K, n(H2)
:
n(CO) = 2
:
1, and GHSV = 4100 h−1. All the experiments were repeated two or three times to check the reproducibility.
3. Results and discussion
3.1. Physico-chemical properties of catalysts
3.1.1 XRD analysis. The XRD pattern of β-Mo2C (Fig. 1) shows diffraction peaks at 34.4°, 38.0°, 39.4°, 52.1°, 61.5°, 69.6° and 74.6°, corresponding to β-Mo2C [100], [002], [101], [102], [110], [103] and [200] (JCPDS card no. 35-0787) respectively with hexagonal closed packed structure, and no peaks of molybdenum oxides or nitrides appear. In other words, pure phase β-Mo2C has been successfully prepared by this one-step thermal decomposition method. For Ni/β-Mo2C catalyst, the intensity of peaks assigned to β-Mo2C become weaker. Moreover, in comparison with the diffraction peaks of β-Mo2C, the peak positions of β-Mo2C in Ni/β-Mo2C shift to higher angular values. This difference is attributed to the promoting effects of Ni. In addition, the new diffraction peaks at 23.2°, 30.2°, 40.8°, 43.1°, 45.4°, 51.6°, 59.6°, 62.7° and 71.0° agree quite well with the crystallographic planes data of Ni3Mo3N (JCPDS card no. 49-1336). Additional signals at 2θ = 26.1°, 66.2° and 69.6° could be corresponded to the MoO2 phase, this may be ascribed to insufficient carbon content due to the addition of Ni or the higher oxidation ability of nitrate introduced in Ni(NO3)2·6H2O.
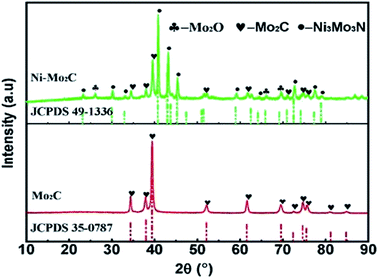 |
| Fig. 1 XRD patterns of β-Mo2C and Ni/β-Mo2C. | |
3.1.2 Pore structure, CO uptake and elemental analysis. The surface area (SBET), CO uptake and elemental analysis of prepared catalyst are summarized in Table 1. The SBET of β-Mo2C (Table 1) is 10.26 m2 g−1, and it decreases to 6.49 m2 g−1 when promoted by Ni, this is consistent with the results obtained from Cheng,22 the specific surface area decreased when the Ni/Mo atomic ratio exceeded 0.2, probably because more phase separation would occur with increasing Ni/Mo atomic ratio.
Table 1 Pore structure, CO uptake and elemental analysis of catalysts
Sample |
Specific surface area (m2 g−1) |
Pore volume (cm3 g−1) |
Average pore diameter (nm) |
CO uptake (μmol g−1) |
Atom content (wt%) |
Moa |
Nia |
Cb |
Nb |
Hb |
Oc |
ICP-AES. Elemental analysis. Calculated by the subtraction. |
β-Mo2C |
10.26 |
0.0399 |
15.57 |
22 |
89.08 |
— |
7.52 |
0.15 |
0.07 |
3.18 |
Ni–Mo2C |
6.49 |
0.0447 |
27.56 |
76 |
69.35 |
20.96 |
2.12 |
1.85 |
0.07 |
5.65 |
The content of C in β-Mo2C is 7.52 wt%, which is much higher than theoretical value of 5.88 wt%, suggesting the existence of redundant pyrolytic carbon originated from the thermal decomposition of excess HMT. The content of O in β-Mo2C is 3.18 wt%, but the MoOx phase is not detected by XRD, which is possibly because the surface of β-Mo2C is just oxidized slightly during passivation and the formed minute MoOx phase is below the detection limit of XRD. For the Ni/β-Mo2C sample, the Ni/Mo (n/n) = 0.494, closing to the nominal composition of 0.5, implies that Ni is well scattered in the bulk phase of Ni/β-Mo2C. The MoO2 phase is detected in Ni/β-Mo2C but not in β-Mo2C, suggesting the introduction of Ni causes the sample easier to be oxidized during the process of passivation. The contents of C and N are 2.12 and 1.85 wt% respectively, which are in close proximity to the nominal composition of C and N, 2.30 and 1.79 wt% respectively, these agree with the assumption that the Ni/β-Mo2C sample is a mixture of β-Mo2C and Ni3Mo3N, i.e. the results of elemental analysis further confirm the above assumption that the Ni/β-Mo2C sample is a mixture of β-Mo2C and Ni3Mo3N.
CO adsorption is used to quantify the amounts of adsorption sites, and the normalized pulsed CO chemisorption (μmol g−1) of β-Mo2C and Ni/β-Mo2C are listed in Table 1. The results show that the CO uptake of Ni/β-Mo2C catalyst is 76 μmol g−1 and it is much higher than that of β-Mo2C (22 μmol g−1). This implies that Ni/β-Mo2C has more catalytic active sites than β-Mo2C, and thus it shows better catalytic activity on methanation.
3.1.3 Morphology of the catalysts. The morphology of β-Mo2C shows platelet-like particles with an average 3.6 μm in size (Fig. 2a), while Ni/β-Mo2C shows an average size of 0.9 μm and it is comprised of homogeneously nucleated spherical particles and a few platelet-like particles embedded in a matrix that primarily consists of large agglomerates of irregularly shaped particles. Moreover, the enlarged images (Fig. 2c and d) show that Ni/β-Mo2C presents as spherical particles with an average size of about 35–55 nm, accumulating on a matrix of large agglomerates, which is much more homogeneous than β-Mo2C with an average size of about 40–130 nm.
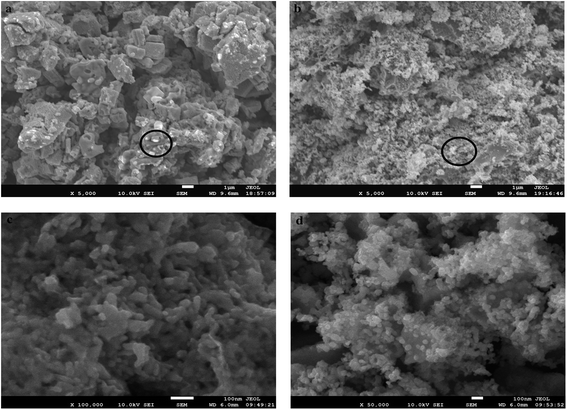 |
| Fig. 2 SEM images of the bulk samples. (a) β-Mo2C, (b) Ni/β-Mo2C, (c) an enlarged image of β-Mo2C and (d) an enlarged image of Ni/β-Mo2C. | |
The TEM micrograph (Fig. 3a) shows agglomerations of β-Mo2C particles, which are coated with a thick layer of carbons. The agglomerations of Ni/β-Mo2C particles are reduced (Fig. 3b) and the carbon deposition could not been observed, indicating that the carbon deposition is inhibited due to the addition of Ni. The d-spacing value of 0.261 nm, which is shown in the HRTEM micrograph of β-Mo2C (Fig. 3c), corresponding to the crystallographic planes of β-Mo2C, is in good agreement with the XRD pattern for single-phase β-Mo2C. Likewise, the d-spacing values of 0.261 nm and 0.639 nm (Fig. 3d) are coincident with the (100) and (111) crystallographic planes of β-Mo2C and Ni3Mo3N respectively based upon the data of XRD results.
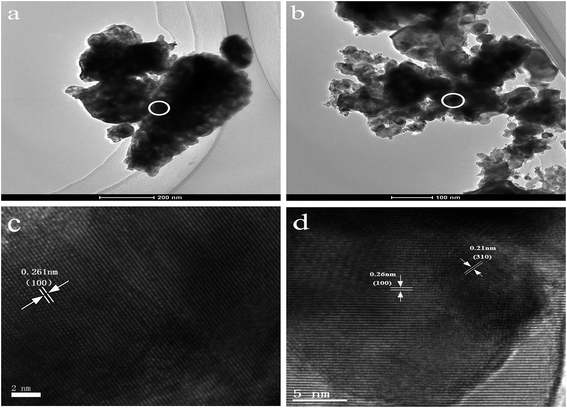 |
| Fig. 3 HRTEM images of the bulk samples. (a) β-Mo2C, (b) Ni/β-Mo2C, (c) an enlarged image of β-Mo2C and (d) an enlarged image of Ni/β-Mo2C. | |
3.1.4 XPS analysis. XPS is one of the important surface analysis techniques and it has been widely used in chemistry, material science, and surface science due to its high sensitivity and resolution. It could detect not only the surface chemical composition but also the chemical state of element. The Mo 3d, C 1s, N 1s, O 1s and Ni 2p spectra were decomposed using an interactive least-squares computer program, the curves being taken as 80% Gaussian and 20% Lorentzian. Surface atomic concentration ratios were calculated as the ratio of the corresponding peak intensities, corrected with the theoretical sensitivity factors based on Scofield's photoionisation cross-sections. The surface composition and the chemical environment of Mo, C, N, O and Ni are summarized in Tables 2 and 3 respectively. The atom ratio of C/Mo in the surface of β-Mo2C sample is 4.03 (Table 2), which is much higher than 0.675 in the bulk phase (Table 1) and the default value of 0.5, this proves that the surface of β-Mo2C has serious carbon deposition. While for Ni/β-Mo2C, the surface atom ratio of C/N = 0.83 is lower than the default value of 1.5, however, the Ni/Mo value of 1.01 (Table 2) in the surface is higher than the theoretical value of 0.5 (Table 1) in the bulk phase, both of these prove that the nitrides are enriched in the surface.
Table 2 Surface composition measured by XPS
Sample |
Surface area contenta (mol%) |
Atom ratioa |
Mo 3d |
Ni 2p |
C 1s |
N 1s |
O 1s |
C/Mo |
C/N |
Ni/Mo |
Calculated from XPS data. |
β-Mo2C |
14.00 |
— |
56.48 |
— |
29.52 |
4.03 |
— |
— |
Ni/β-Mo2C |
9.12 |
9.20 |
23.56 |
28.32 |
29.80 |
2.58 |
0.83 |
1.01 |
Table 3 Chemical environment of Mo, C, N, O and Ni estimated by XPS
Sample |
Mo 3d5/2 (content/%) |
C 1s (content/%) |
N 1s–Mo 3p3/2 (content/%) |
O 1s (content/%) |
Ni 2p3/2 (content/%) |
Mo2+ |
Moδ+ |
Mo5+ |
Mo6+ |
C–Mo |
C–C |
C–O |
N–Mo |
N–O |
O–Mo |
O–C |
O–H |
Ni–Mo |
Ni–O |
Nisatel |
β-Mo2C |
19.82 |
24.06 |
23.10 |
33.02 |
8.98 |
54.85 |
36.17 |
— |
— |
47.34 |
23.20 |
29.46 |
— |
— |
— |
Ni/β-Mo2C |
9.99 |
21.64 |
18.95 |
49.42 |
10.06 |
81.00 |
8.94 |
39.42 |
60.58 |
67.34 |
24.23 |
8.43 |
8.45 |
59.89 |
31.66 |
The XPS peaks of Mo 3d, C 1s, N 1s, O 1s and Ni 2p3/2 spectra for the samples are shown in Fig. 4. For β-Mo2C, the fitting results to the raw data reveal the presence of four species of Mo (Fig. 4a1), one is at the binding energy of 228.3 eV (Mo 3d5/2), denoted as Mo2+, which is consistent with the carbide form. The peak at the binding energy of 228.9 eV (Mo 3d5/2), which is between the values of 228.3 eV (Mo2+) and 229.5 eV (Mo4+), is believed to correspond to the oxidation state of Moδ+ (2 < δ < 4). The remaining two peaks at the binding energy of 231.0 eV (Mo 3d5/2) and 232.6 eV (Mo 3d5/2) are characteristic of Mo5+ and Mo6+ respectively, which should come from the surface oxidation of carbidic Mo in the passivation process. Moreover, four species of Mo are also found in Ni/β-Mo2C (Fig. 4b1), but the Mo 3d spectra shift to a litter lower binding energy, which indicates that Ni has an electronic interaction with Mo. As an electronic promoter, the electron density released by Ni is transferred to Mo. It is noteworthy that the percentage of Mo2+, Moδ+ and Mo5+ in Ni/β-Mo2C is lower than that of β-Mo2C, while the percentage of Mo6+ is higher than that of β-Mo2C (Table 3), this further proves that the introduction of Ni could promote the oxidization of Mo, i.e. Ni/β-Mo2C is easier to be oxidized by nitrate in the raw material of Ni(NO3)2·6H2O or in the process of passivation.
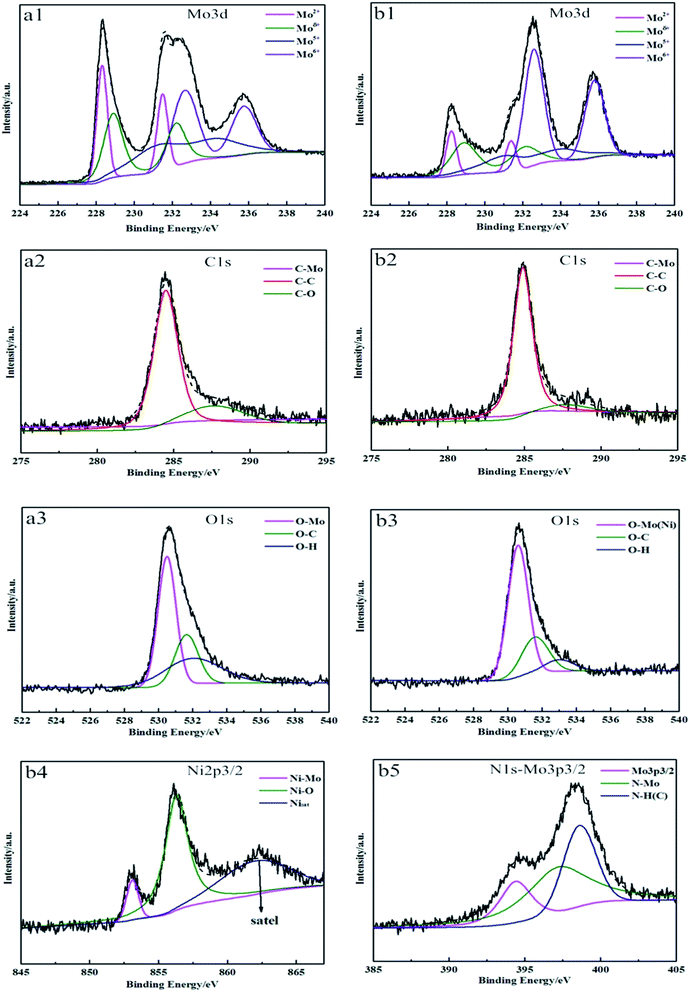 |
| Fig. 4 XPS spectra of Mo 3d, C 1s, O 1s and Co 2p3/2 of (a) β-Mo2C and (b) Ni/β-Mo2C. Thick curves represent the raw data, color curves represent the results of deconvolution of the spectrum into Lorentzian-broadened Gaussian peaks, and dotted curves represent the resulting fit to the raw data. | |
Three types of carbon are detected in β-Mo2C and Ni/β-Mo2C (Fig. 4a2 and b2). The C 1s spectrum presents a main peak at around 284.8 eV, which is attributed to graphitic carbon enriched in the surface. The remaining components at 283.4 eV and 287.5 eV could be assigned to carbidic carbon and oxidized carbon respectively. It is noticeable that, the proportion of free carbon in the surface of Ni/Mo2C is much higher than that of β-Mo2C (Table 3) although the total surface carbon of Ni/Mo2C is much less than that of β-Mo2C (Table 2).
Likewise, in the case of O 1s spectra, three species of O are present in β-Mo2C and Ni/β-Mo2C (Fig. 4a3 and b3). The most difference is the proportion of O in O–Mo form, at the binding energy of around 530.6 eV, is much higher in Ni/β-Mo2C than that in β-Mo2C, which results in higher percentage of Mo6+ in Ni/β-Mo2C (Table 3). This is other evidence that Ni/β-Mo2C is easier to be oxidized compared with the sample of β-Mo2C.
The peak at 853.1 eV of Ni 2p3/2 spectrum in Fig. 4b4 is assigned to Ni atom in Ni–Mo carbide. The peak at 856.3 eV and the intense shake-up satellite structures are in agreement with the presence of NiOx at the surface.
Since the N 1s peak is enveloped in that of Mo 3p3/2, i.e. the peak measured is actually N 1s–Mo 3p3/2, and thus the intensity of N 1s need to be calculated from the N 1s–Mo 3p3/2 peak area. Since the theoretical value of Mo 3p3/2 to Mo 3p1/2 peak area ratio is 2/1, the area of N 1s could be obtained by subtracting an area that is double that of Mo 3p peak area from the N 1s–Mo 3p3/2 (Table 3). In addition, three peaks are present in the N 1s–Mo 3p3/2 spectra of Ni/β-Mo2C (Fig. 4b5), the peak at 394.4 eV is assigned to Mo 3p3/2, and that at 397.2 and 398.6 eV are corresponded to N–Ni(Mo) and N–H(C) respectively.
3.1.5 H2-TPSR analysis. The H2-TPSR results of Ni/β-Mo2C and β-Mo2C show considerable differences (Fig. 5). The main gaseous products obtained by H2-TPSR are CH4, H2O and NH3, no CO or CO2 are observed. Thus, all of the carbon in the samples, both carbidic and free, is essentially evolved as CH4. Likewise, all of the nitrogen is evolved as NH3. The β-Mo2C exhibits a peak at about 653 K, which is assigned to carbidic carbon. And another peak at about 993 K (Fig. 5a) is assigned to pyrolytic carbon.23,24 While for Ni/β-Mo2C, the intensity of peak assigned to carbidic carbon becomes weaker and shifts to higher temperature at about 720 K (Fig. 5a). In addition, the peak assigned to pyrolytic carbon is not observed, which indicates little pyrolytic carbon deposition in Ni/β-Mo2C. The NH3 desorption profiles during H2-TPSR are shown in Fig. 5b, three peaks at about 410 K, 700 K and 850 K, respectively are observed in Ni/β-Mo2C. The peak at about 700 K may be assigned to nitrogen in nitride. While for β-Mo2C, it also presents three peaks at about 410 K, 600 K and 950 K, respectively. It is noticeable that their intensities are much lower than that of Ni/β-Mo2C, which implies that the nitrogen content in β-Mo2C is much less than in Ni/β-Mo2C.
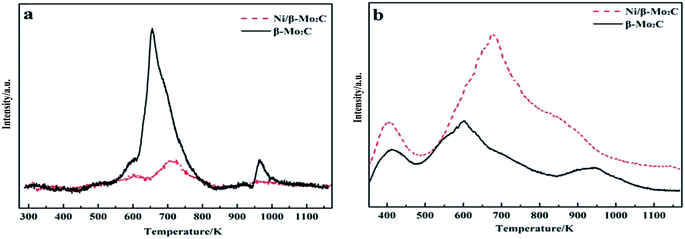 |
| Fig. 5 CH4 and NH3 desorption profiles during H2-TPSR over β-Mo2C and Ni/β-Mo2C (a) CH4 and (b) NH3. | |
3.2. Catalytic activities for methanation
The changes in CO conversion and CH4 selectivity with time are shown in Fig. 6. The stability tests were conducted under the conditions of T = 773 K, P = 3.0 MPa, n(H2)
:
n(CO) = 2
:
1 and GHSV = 4100 h−1, and every run was continued for 100 h. As shown in Fig. 6a, the XCO and SCH4 on β-Mo2C decrease from initial 75.93% and 36.79% to 67.41% and 33.54% respectively within 100 h, this is caused by hydrogenation of carbidic carbon in bulk β-Mo2C. At the same reaction conditions, the XCO and SCH4 on Ni/β-Mo2C are much higher than those of β-Mo2C, which increase from initial 90.86% and 45.11% to 92.90% and 53.28% (Fig. 6b) respectively and this is contrary to the variation trend of β-Mo2C. It could be concluded that the phase of Ni3Mo3N is responsible for the higher activity and stability. A. Erhan Aksoylu and Z. Ìlsen Önsan25 reported that the Ni–Mo synergetic interaction increased the total hydrocarbon production in CO hydrogenation reaction. M. Nagai14 applied Ni–Mo carbide catalysts in the WGS reaction and they found that the added Ni produced fine particles of Ni–Mo carbide and caused a high CO adsorption.
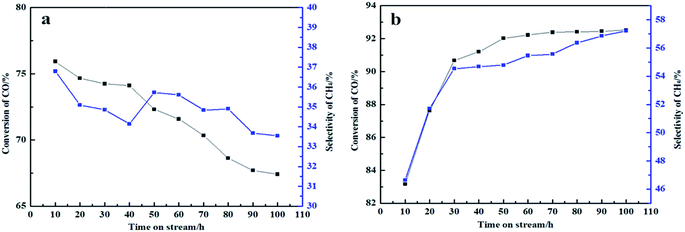 |
| Fig. 6 Stability tests over (a) β-Mo2C and (b) Ni/β-Mo2C catalysts. | |
As shown in Table 4, the CH4 selectivity increase remarkably and the CO2 selectivity decrease on account of the promotion of Ni and then the production of Ni3Mo3N, this is ascribed to the intrinsically nature that Ni is an activity element for carbon monoxide hydrogenation especially methanation. L. H. Zhao26 and M. L. Xiang27,28 studied the Ni–Mo bimetallic carbide catalysts in CO hydrogenation reaction, they found that the Ni–Mo bimetallic carbide catalysts were much more active than the molybdenum carbide catalyst and the CH4 selectively increased due to its CO dissociation and high hydrogenation activity.
Table 4 Performances of methanation on different catalystsa
Catalysts |
XCO (C%) |
Rate (mol h−1 gcatal−1) |
SCO2 (C%) |
Selectivity of hydrocarbon product (C%) |
Sliquid (C%) |
SCH4 |
SC2H6 |
SC2H4 |
Time on stream: 100 h. |
β-Mo2C |
67.41 |
1.85 |
38.34 |
33.54 |
16.15 |
4.34 |
7.63 |
Ni/β-Mo2C |
92.51 |
3.09 |
32.71 |
57.23 |
1.83 |
0.11 |
8.12 |
Moreover, Xiang29 also conducted CO hydrogenation over Ni promoted β-Mo2C catalyst, mixed alcohols were the main products and the selectivity of alcohols reached the maximum of 30%. However, no methanol, ethanol or other alcohols were found in liquid products under our reaction conditions, and only water, bits of glycol as well as isomers, and thimbleful acetic anhydride were detected because of higher temperature were used in this paper, this is complied with the law of thermodynamics. From the point of thermodynamics, lower temperature is in favor of synthesis of alcohols and higher temperature is advantageous to the increasing of selectivity of higher alcohols.
3.3. Characterization of spent catalysts
XRD patterns of spent β-Mo2C and Ni/β-Mo2C catalysts are shown in Fig. 7a and b respectively. The β-Mo2C is transformed to MoC after reaction due to hydrogenation of carbidic carbon (Fig. 7a). The temperature of CH4 desorption assigned to carbidic carbon during H2-TPSR in β-Mo2C is lower than the reaction temperature used in the stability tests, so the hydrogen in the syngas could not only react with CO, but also with carbidic carbon, and this causes crystal transfer of β-Mo2C, then the catalytic activity decrease.
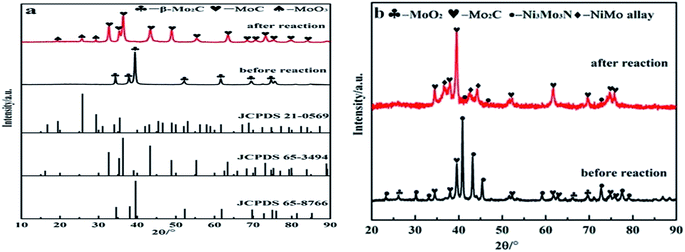 |
| Fig. 7 XRD patterns of spent catalysts, (a) β-Mo2C and (b) Ni/β-Mo2C. | |
As for Ni/β-Mo2C (Fig. 7b), the peaks that corresponded to the β-Mo2C phase become sharper with respect to the previous pattern. The peaks of Ni3Mo3N tend to broaden, their intensities decrease and even disappear. Instead, the peaks that corresponded to Ni–Mo alloy appear due to hydrogenation of nitrogen in Ni3Mo3N. From the point of thermodynamics, N is more easily reacted with H2 than C. Furthermore, the generation of Ni–Mo alloy and the better crystal form of β-Mo2C are beneficial to dissociative adsorption of CO (98 μmol g−1 after reaction), and these gradually increase the catalytic activity on methanation as the reaction is progressed.
4. Conclusions
The β-Mo2C was prepared successfully by the one-step thermal decomposition method, and it showed high CO conversion but low CH4 selectivity due to the crystal transformation from β-Mo2C to MoC, which was caused by the hydrogenation of carbidic carbon. While the bimetallic Ni–Mo carbide catalyst showed an excellent activity, the CO conversion and CH4 selectivity were significantly increased, and the stability was also increased due to the promotion of Ni. This is ascribed to the formation of Ni3Mo3N in Ni–Mo carbide, which is more active and stable than the β-Mo2C during methanation.
Acknowledgements
The work is financially supported by the Strategic Priority Research Program of the Chinese Academy of Sciences (XDA07050100), the Natural Science Fund of Shanxi Province (2013021007-2), the Research Supported by the CAS/SAFEA International Partnership Program for Creative Research Teams and Youth Innovation Promotion Association (2014156).
Notes and references
- A. L. Kustov, A. M. Frey, K. E. Larsen, T. Johannessen, J. K. Nørskov and C. H. Christensen, Appl. Catal., A, 2007, 320, 98–104 CrossRef CAS.
- M. Y. Kim, S. B. Ha, D. J. Koh, C. Byun and E. D. Park, Catal. Commun., 2013, 35, 68–71 CrossRef CAS.
- B. C. Enger and A. Holmen, Catal. Rev., 2012, 54, 437–488 CAS.
- G. A. Mills and F. W. Steffgen, Catal. Rev., 1974, 8, 159–210 Search PubMed.
- J. Oudar, Catal. Rev., 1980, 22, 171–195 CAS.
- E. J. Erekson and C. H. Bartholomew, Appl. Catal., 1983, 5, 323–336 CrossRef CAS.
- J. Frank, H. A. Dick, J. Goral, A. J. Nelson and M. Grätzel, J. Catal., 1990, 126, 674–676 CrossRef.
- Y. Li, R. Wang and L. Chang, Catal. Today, 1999, 51, 25–38 CrossRef CAS.
- J. Chen, S. L. Li, Q. Xu and K. Tanaka, Chem. Commun., 2002, 1722–1723 RSC.
- B. Wang, G. Ding, Y. Shang, J. Lv, H. Wang, E. Wang, Z. Li, X. Ma, S. Qin and Q. Sun, Appl. Catal., A, 2012, 432, 144–150 CrossRef.
- B. Wang, Y. Shang, G. Ding, J. Lv, H. Wang, E. Wang, Z. Li, X. Ma, S. Qin and Q. Sun, React. Kinet., Mech. Catal., 2012, 106, 495–506 CrossRef CAS.
- H. C. Woo, I. S. Nam, J. S. Lee, J. S. Chung, K. H. Lee and Y. G. Kim, J. Catal., 1992, 138, 525–528 CrossRef CAS.
- J. M. Christensen, P. M. Mortensen, R. Trane, P. A. Jensen and A. D. Jensen, Appl. Catal., A, 2009, 366, 29–43 CrossRef CAS.
- M. Nagai, A. M. Zahidu and K. Matsuda, Appl. Catal., A, 2006, 313, 137–145 CrossRef CAS.
- J. Patt, D. J. Moon and C. Phillips, Catal. Lett., 2000, 65, 193–195 CrossRef CAS.
- M. Nagai and K. Matsuda, J. Catal., 2006, 238, 489–496 CrossRef CAS.
- P. Liu and J. A. Rodriguez, J. Phys. Chem. B, 2006, 110, 19418–19425 CrossRef CAS PubMed.
- P. Afanasiev, Inorg. Chem., 2002, 41, 5317–5322 CrossRef PubMed.
- H. M. Wang, X. H. Wang and M. H. Zhang, Chem. Mater., 2007, 19, 1801–1807 CrossRef CAS.
- X. H. Wang, M. H. Zhang, W. Li and K. Y. Tao, Dalton Trans., 2007, 36, 5165–5170 RSC.
- H. M. Wang, M. H. Zhang and W. Li, Catal. Today, 2008, 131, 111–117 CrossRef.
- J. M. Cheng and W. Huang, Fuel Process. Technol., 2010, 91, 185–193 CrossRef CAS.
- T. Miyao, I. Shishikura, M. Matsuoka, M. Nagal and S. T. Oyama, Appl. Catal., A, 1997, 165, 419–428 CrossRef CAS.
- S. Izhar, H. Kanesugi, H. Tominaga and M. Nagai, Appl. Catal., A, 2007, 317, 82–90 CrossRef CAS.
- A. Erhan Aksoylu and Z. Ìlsen Önsan, Appl. Catal., A, 1998, 168, 399–407 CrossRef.
- L. H. Zhao, K. G. Fang, D. Jiang, D. B. Li and Y. H. Sun, Catal. Today, 2010, 158, 490–495 CrossRef CAS.
- M. L. Xiang, D. B. Li, H. C. Xiao, J. L. Zhang, W. H. Li, B. Zhong and Y. H. Sun, Catal. Today, 2008, 131, 489–494 CrossRef CAS.
- M. L. Xiang, D. B. Li, W. H. Li, B. Zhong and Y. H. Sun, Catal. Commun., 2007, 8, 88–93 CrossRef CAS.
- M. L. Xiang, D. B. Li, W. H. Li, B. Zhong and Y. H. Sun, Catal. Commun., 2007, 8, 503–507 CrossRef CAS.
|
This journal is © The Royal Society of Chemistry 2016 |