DOI:
10.1039/C5RA23519G
(Paper)
RSC Adv., 2016,
6, 29127-29134
Efficient lipid extraction and quantification of fatty acids from algal biomass using accelerated solvent extraction (ASE)
Received
8th November 2015
, Accepted 7th March 2016
First published on 9th March 2016
Abstract
Accelerated solvent extraction (ASE), a commercially available pressurized fluid extraction technique and conventional manual extraction were compared to identify the most effective chloroform–methanol extraction method for algal lipids. Using optimal ASE operating conditions (methanol/chloroform = 2
:
1 by vol, 100 °C, static time of 5 min, and four static cycles), the lipid contents of Chlorella vulgaris, C. sorokiniana, C. zofingiensis and Nannochloropsis gaditana were 27.5%, 25.8%, 15.2%, 29.8% of dry biomass, respectively. The total fatty acid methyl ester (FAME) content of dry biomass from ASE extraction was found to be 1.3–2.7 fold higher than that from conventional manual extract from these species, demonstrating that ASE exhibited significant improvement for lipid and FAME recovery. Furthermore, ASE showed the capacity to extract all-cis-5,8,11,14,17-eicosapentaenoic acid (EPA) as 3.0% of dry biomass from Nannochloropsis gaditana suggesting that ASE has the potential to obtain polyunsaturated fatty acids (PUFAs) as well.
1. Introduction
The demand for biofuels is increasing due to limitations in oil supply, rising greenhouse gas emissions, and the associated impact on global climate. Third generation biofuels, such as microalgae, represent one potential alternative energy source going forward, since they do not compete with food and crops.1 Microalgal photosynthetic efficiency, growth performance and biomass productivity are also higher compared to other oleaginous cultures.2 Moreover, microalgae species can adapt to a variety of environmental conditions and can be genetically manipulated.3,4
Chlorella and Nannochloropsis genera of microalgae are known for producing significant amounts of lipid. C. vulgaris has shown to produce biodiesel precursors, and nitrogen limitation contributes to increased lipid content.5 C. sorokiniana is another promising freshwater alga for accumulating high amounts of lipids and has been found to be resistant to heat and high light intensity.6,7 C. zofingiensis is a particular green alga that can grow well photoautotrophically as well as heterotrophically and a candidate to generate astaxanthin and lutein.8 Nannochloropsis spp. are promising algae for biodiesel production due to their successful cultivation at larger scales using natural sunlight. These species can achieve high levels of biomass production and contain high lipid contents, including polyunsaturated fatty acids.9–11
Lipids existing in algae can be classified as:12,13 (i) neutral lipids, which serve as energy storage products, including triacylglycerols (TAG), diacylglycerols (DAG), monoacylglycerols (MAG), free fatty acids, hydrocarbons, wax, sterols etc.; (ii) phospholipids, which are mainly in cell membranes, including phosphatidylglycerol (PG), phosphatidylinositol (PI), phosphatidylethanolamine (PE), phosphatidylcholine (PC); and (iii) glycolipids, which are mainly in cell membranes, including sulfoquinovosyldiacylglycerol (SQDG), monogalactosyldiacylglycerol (MGDG), and digalactosyldiacylglycerol (DGDG). The major fatty acids in microalgae are comprised of 12–22 carbon atoms with between 0–6 double bonds.12 Esterifiable lipids can be converted into fatty acid methyl esters (FAME), which are the main components of biodiesel. The FAME profiles of microalgae have a significant effect on the quality of biodiesel. Microalgae with FAME profiles containing predominantly C:16 and C:18 are best suited for biodiesel production, which has been investigated previously.7,14–16
Lipid extraction is an important, yet time-consuming and cost intensive, step of algal species selection and biodiesel production. Organic solvent extraction is widely used to extract lipid from microalgae, but the use of organic solvents is associated with health, security and regulatory issues. Cell wall disruption technologies have been reported along with organic solvent extraction in literature reports in order to achieve higher lipid extraction efficiencies,17 but the method can add costs and take time. Different disruption methods including sonication, osmotic shock, autoclaving, microwave, bead-beating treatments have been tested in Chlorella sp., Nostoc sp. and Tolypothrix sp. Sonication performed best among those treatments in Chlorella sp. and Nostoc sp., with efficiencies of 21% and 19% of dry biomass, respectively. Microwave treatment showed the highest efficiency (17%) in Tolypothrix sp.18 These findings indicate that the most efficient treatment of breaking microalgal cell wall depends on the particular microalgal species.
Accelerated solvent extraction (ASE) is a promising automated extraction method that offers various extraction parameters.19 Due to the use of elevated temperatures and pressures, the ASE method reduces consumption of solvents compared to other methods. Previous research suggested that as much as 90% of organic solvent usage could be reduced by using ASE.20 Higher amounts of lipids can be recovered using a optimal ASE method from cereal, egg yolk and chicken breast muscle samples compared to a modified Folch procedure.21 In addition to lipid extraction, ASE can be a promising technology to effectively extract valuable compounds under optimized conditions,12 such as polycyclic aromatic hydrocarbons, polychlorinated biphenyls and total petroleum hydrocarbons from solid samples22 and pigments from Haematococcus pluvialis and Dunaliella salina.23 Furthermore, ASE can be used to extract antioxidants from microalga Spirulina platensis and the solvent system used in ASE was found to have a significant effect on recovery.24
In this work, we report the utilization of ASE to extract lipids from C. vulgaris, C. sorokiniana, C. zofingiensis, and Nannochloropsis gaditana and compare the lipid recoveries from ASE to conventional manual extraction methods across these four commercially-relevant microalgal species. The optimal ASE conditions, including solvents, reaction temperature, static time and static cycles were investigated. The fatty acid methyl ester (FAME) and polyunsaturated fatty acids (PUFAs) recovery were also compared between ASE and conventional manual extraction in order to evaluate the full potential of these methods.
2. Materials and methods
2.1 Materials
Stock samples of C. vulgaris UTEX 395, C. sorokiniana UTEX 1230, C. zofingiensis UTEX 32 were obtained from the Culture Collection of Algae at University of Texas at Austin (http://www.web.biosci.utexas.edu/utex/). Nannochloropsis strain number Chl-2 was purchased from IOCAS (Institute of Oceanology, Chinese Academy of Sciences), Qingdao, China and identified as Nannochloropsis gaditana by the colony PCR and phylogenetic analysis methods of Rosenberg et al.7 Chlorella powders were purchased from Hoosier Hill Farm, USA. HPLC-grade organic solvents including chloroform, methanol, ethanol, acetone, butanol, heptane and hexane were obtained from Sigma, USA. FAME standards including methyl heptadecanoate, methyl palmitate, methyl palmitoleate, methyl oleate, methyl linoleate, methyl linolenate, cis-5,8,11,14,17-eicosapentaenoic acid methyl ester, heptadecanoic acid were purchased from Sigma, USA. Analytical-grade hydrochloric acid was supplied by Sigma, USA.
2.2 Microalgal cultivation conditions
C. vulgaris, C. sorokiniana, and C. zofingiensis were cultured in Bold's basal medium (BBM)25 containing the following components: 0.25 g L−1 NaNO3, 25 mg L−1 CaCl2·2H2O, 75 mg L−1 MgSO4·7H2O, 75 mg L−1 K2HPO4, 175 mg L−1 KH2PO4, 25 mg L−1 NaCl, and 1 mL of each of the trace element stock solutions in 1 L dH2O: 50 g EGTA and 31 g KOH in 1 L dH2O; 4.98 g FeSO4·7H2O, 1.0 mL H2SO4 in 1 L dH2O; 11.42 g L−1 H3BO3; 1.44 g MnCl2·4H2O, 8.82 g ZnSO4·7H2O, 1.57 g CuSO4·5H2O, 0.71 g MoO3, and 0.49 g Co(NO3)2·6H2O in 1 L dH2O. Nannochloropsis gaditana was cultured in BG-11 medium containing the following components: 1.5 g L−1 NaNO3, 40 mg L−1 KH2PO4·3H2O, 75 mg L−1 MgSO4·7H2O, 36 mg L−1 CaCl2·2H2O, 6.0 mg L−1 citric acid, 6.0 mg L−1 ferric ammonium citrate, 1.0 mg L−1 EDTA, 20 mg L−1 Na2CO3, and 1.0 mL L−1 A5+Co* solution. The A5+Co* solution contained 2.86 g L−1 H3BO3, 1.81 g L−1 MnCl2·H2O, 222 mg L−1 ZnSO4·7H2O, 79 mg L−1 CuSO4·5H2O, 390 mg L−1 Na2MoO4·2H2O, and 49 mg L−1 Co(NO3)2·6H2O.26 Growth experiments were carried out in 3 L batch cultures with ambient air bubbled at 1 L min−1. Cultures were illuminated with white fluorescent light at 100 μE m−2 s−1 with 12/12 h light/dark photoperiod at 25 °C throughout the experiment. Liquid cultures were harvested using a high-speed centrifuge (Beckman J2-21, Baltimore, USA) at 4000 × g for 10 min and lyophilized for 24 h at −40 °C under vacuum.
2.3 Lipid extraction using ASE method
Lipid extraction was carried out using the ASE instrument (Dionex ASE 150). In brief, dry biomass was filled in 22 mL stainless steel cells and cellulose fiber filters (Dionex, USA) were used to prevent blockage of the frit in the bottom cap. The ASE program was set at different experimental conditions: extraction solvent was methanol and chloroform (2
:
1 and 1
:
2, v/v), methanol, ethanol, acetone, butanol, butanol and heptane (1
:
4, v/v) and hexane separately, extraction temperature was from 60 to 120 °C, static time was from 5 to 15 min, and a static cycle number were from 2 to 5. The extraction procedure included the following steps: (1) the cell was rinsed using the extraction solvent (2) extraction cell with biomass samples were loaded into the machine; (3) the cell was filled with solvents up to a pressure of 1500 psi; (4) the cell was preheated for 5 min (5) the extraction was performed for 35 min (flush volume: 100% of cell volume, purge time: 60 s); (6) the cell was rinsed again using the extraction solvent. The extracts were then collected in 60 mL collection vials for analysis.
The obtained extracts were air-dried and lipid content was determined gravimetrically. Purchased Chlorella powder was used to identify the optimal ASE conditions. Fresh C. vulgaris, C. sorokiniana, C. zofingiensis and Nannochloropsis gaditana biomass samples were cultivated for lipid extraction experiments under the optimized ASE conditions. All experiments were performed in duplicate.
2.4 Lipid extraction using conventional manual extraction
For the conventional extraction method, 102 mg of lyophilized biomass (C. vulgaris, C. sorokiniana, C. zofingiensis and Nannochloropsis gaditana) was blended with 10 mL solvent mixture of methanol and chloroform (2
:
1, v/v) in a flask.27 The mixture was ground using mortar and pestle for 10 min, then the mixture was transferred into a conical test tube and stirred for 30 min. After that, the mixture of the algal cells and the solvent was separated by centrifugation at 8000 × g for 10 min, and the extraction procedure was repeated. The supernatant containing lipid was collected in an amber vial and dried with airflow.
2.5 Transesterification of lipids and quantification by gas chromatography
Transesterification of pre-weighted and air-dried lipids was executed in the presence of 500 μg heptadecanoic acid (C17-fatty acid) as an internal standard. The procedure was conducted 3 mL H2SO4/MeOH (5%, v/v) at 70 °C for 1.5 h. The resulting FAME fraction was extracted by hexane at room temperature for 10 min and the process was performed duplicated.
Fatty acid methyl esters were ultimately analyzed by GC (Shimadzu 2010) with discharge ionization detection equipped with a capillary column (Stabilwax-DA, 30 m × 0.25 mm ID, film thickness 0.25 μm). The injector temperature of GC was set at 250 °C and the detector was set at 260 °C. The temperature program was started at 50 °C and then increased to 170 °C at a rate of 20 °C min−1, plateau for 1 min. The rate was decreased to 4 °C min−1 from 170 to 220 °C and kept constant remained for 14 min. Helium was used as the constant carrier gas.
The major FAME components (methyl palmitoleate (C16:1), methyl palmitate (C16:0), methyl cis-9,12-hexadecadienoate (C16:2), methyl cis-6,9,12-hexadecatrienoate (C16:3), methyl oleate (C18:1), methyl linoleate (C18:2), methyl linolenate (C18:3) and methyl all-cis-5,8,11,14,17-eicosapentaenoate (C20:5n3) were quantified by comparing the GC peak areas of the FAME corresponding standards. The 5-point calibration curves (0.5–2.5 mg mL−1) of each FAME standard were established.
3. Results and discussion
3.1 Optimization of ASE parameters
3.1.1 Effect of solvents on lipid recovery using ASE method. Total lipids from commercially available Chlorella powder were extracted using the ASE method under various operating parameters including solvent systems, temperature, static time and static cycles in order to identify the optimized ASE operating conditions. Initially, different solvents in the ASE instrument were compared. Methanol and chloroform (2
:
1 and 1
:
2, v/v), methanol, ethanol, acetone, butanol, butanol and heptane (1
:
4, v/v) and hexane were used as shown in Fig. 1A. It was found that methanol and chloroform (2
:
1, v/v) extracted up to 25.1 ± 0.2% lipid from the dry biomass, which was greater than the 19.7 ± 1.4% obtained using methanol and chloroform (1
:
2 v/v). These results show that higher chloroform content, and accordingly lower polarity solvent system, did not improve extraction efficiency. The lipid content was found to be 24.8 ± 0.3% using methanol alone, which was very close to that using methanol and chloroform (2
:
1, v/v). This finding indicates that an increase in the polarity of the solvent system may not be effective in extracting significantly more lipids. This result is consistent with a previous study that concluded that extraction efficiency may be inversely proportional to the polarity of the solvent.28 In addition, the carrying capacity of the solvent might also decline with a more polar solvent due to lower miscibility.12 Based on these trends, further increases in the methanol levels in the methanol/chloroform system were not evaluated in the present study. Compared to the chloroform/methanol solvent system, none of the other solvents were as effective in extracting lipids. While ethanol extracted 18.2 ± 2.0%, all other solvents (acetone, butanol, butanol and heptane (1
:
4, v/v), and hexane) were relatively ineffective at lipid extraction using ASE. The non-polar solvent hexane presented the lowest lipid content of 1.1 ± 0.1%, likely due to its low polarity. Indeed, lipid recovery depends strongly on the polarity of the solvent and our results were consistent with this.29 Polar solvents likely penetrate the microalgal cell wall more easily than non-polar solvents and have better contact between lipid and solvent for increased overall lipid extraction. Given these findings, polar and selective solvents are preferred and, in particular, methanol and chloroform (2
:
1, v/v) was chosen as the extraction solvent for subsequent evaluation of the ASE system.
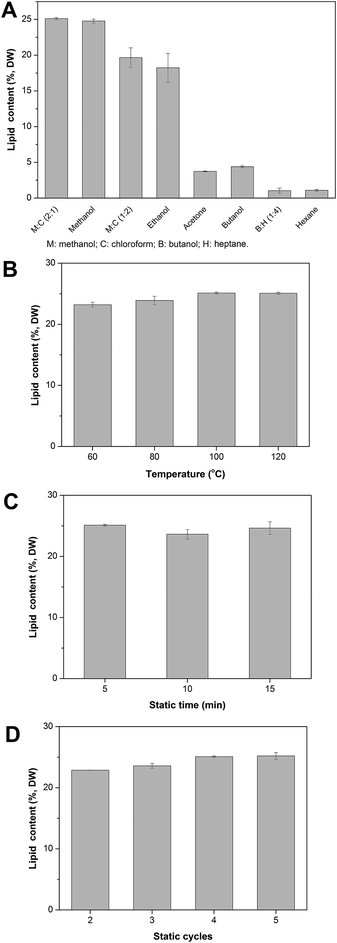 |
| Fig. 1 Effects of reaction parameters on lipid recovery using the ASE method. (A) Effect of extraction solvents. Reaction conditions: extraction temperature of 100 °C, static time of 5 min, static cycle number of 4. (B) Effect of temperature. Reaction conditions: methanol/chloroform (2 : 1, v/v), static time of 5 min, static cycle number of 4. (C) Effect of static time. Reaction conditions: methanol/chloroform (2 : 1, v/v), extraction temperature of 100 °C, static cycle number of 4. (D) Effect of static cycles. Reaction conditions: methanol/chloroform (2 : 1, v/v), extraction temperature of 100 °C, static time of 5 min. Error bars designate standard deviation from the average of duplicates. | |
Mulbry et al. also compared the lipid extraction efficiency using three solvents (chloroform/methanol, isopropanol/hexane, and hexane) in the ASE system with the green algae Rhizoclonium hieroglyphicum cultivated in an outdoor and indoor system with dairy manure effluent and fresh water aquarium. This study demonstrated that the lipid content was 6.1–11.0% of dry biomass using chloroform/methanol, 5.3–6.5% of dry biomass using isopropanol/hexane, while only 0.9–2.8% of dry biomass using hexane,30 which is consistent with our findings.
To design a rational extraction system, the polarity index and the solubility of the solvent and the lipid should be matched. Several factors such as volatility, solubility, selectivity, toxic, cost, safety etc. should be considered. There are some other solvent systems to extract lipid. For example, the isopropanol
:
hexane (2
:
3, v/v) system has been studied.21,31,32 Ryckebosch et al. found that total lipid yield obtained from the chloroform/methanol system was higher than that from the isopropanol and hexane system.31 Though the isopropanol and hexane system has lower toxicity compared to the standard chloroform/methanol system, it has a lower selectivity for membrane lipids such as polar lipids and glycolipids, and the chloroform/methanol system provides the most quantitative and reproducible recovery of all lipid classes.32 Schäfer also reported that the extracted muscle lipids by the chloroform/methanol system has a higher fatty acid content compared to the isopropanol and hexane system.21 Therefore, further investigation of the isopropanol and hexane system was not conducted in this work. Jones et al. indicated that 2-ethoxyethanol (2-EE) provides for excellent lipid recovery compared to the chloroform/methanol system,33 but 2-ethoxyethanol (2-EE) belongs to ionic liquids, which have high viscosity and are sensitive to impurities. These require strict reaction conditions and, thus, were not considered as a commonly used extraction solvent. However, ionic liquids (ILs) have low toxicity, flexible solubility and polarity and can serve as green solvents to extract lipids.34,35 Further study on a more environmentally friendly solvent system that could be applied in the ASE system will be worthwhile.
In terms of solvent ratios used with other biomass matrices, Dufreche et al. established that the oil yield of dried municipal sewage sludge by conventional manual extraction using a hexane, methanol and acetone (3
:
1
:
1, v/v/v) solvent system was 27.4% of the dry biomass and the biodiesel generated from the extraction oil was 4.4% of the dry biomass. Dried sewage sludge could serve as another promising feedstock to generate biodiesel.36 Furthermore, Revellame et al. investigated the ASE method using hexane, methanol and acetone (3
:
1
:
1, v/v/v) solvent system to extract lipid from activated municipal sludge. The lipid content was around 12% of the dry biomass and the FAME yield generated from ASE was around 3.5% of the dry biomass.37 Compared to our study, it shows that the lipid contents of microalgae are generally higher than that of sludge using the ASE method, and more FAME was recovered from microalgae. The optimal ASE parameters and the superior characteristics of microalgal feedstocks could both contribute to the higher recovery of lipid and FAME. Revellame et al. also compared the ASE method to the Bligh & Dyer method and found there was no significant difference between the two methods.37 Lipid recovery could have been higher if the optimal ASE parameters were applied, and the biodiesel yield from sewage sludge might be improved as well. Collectively, these studies suggest that the ASE method has the potential to be widely used to extract lipids from different biomass samples.
3.1.2 Effect of temperature on lipid recovery using ASE method. The reaction temperature chosen for ASE also exhibited some effects on the efficacy of lipid extraction. Lipid contents obtained at 60 °C, 80 °C, 100 °C and 120 °C are summarized in Fig. 1B. The lipid content was 23.2 ± 0.4% at 60 °C. When the reaction temperature was increased to 80 °C, a higher lipid content of 23.9 ± 0.7% could be achieved and content increased progressively with temperature up to 25.1 ± 0.2% at 100 °C. The higher temperature may contribute to the disruption of the cell wall to enable the solvent contact the lipids more thoroughly. When the temperature was further increased to 120 °C, the lipid content did not increase significantly. Temperatures higher than 120 °C were not studied because the highest lipid content was very close at 100 °C and 120 °C to suggest that higher temperature may not contribute to higher lipid recovery. Hence, a reaction temperature of 100 °C was chosen as the optimum value in this study.
3.1.3 Effect of static time on lipid recovery using ASE method. The lipid contents achieved by adopting different static time periods are shown in Fig. 1C. Static time represents the number of minutes that the sample and solvent are maintained at the set temperature. The lipid content was 25.1 ± 0.2% at a 5 min static time and the content decreased slightly to 23.6 ± 0.8% at 10 min and increased slightly to 24.6 ± 1.0% at 15 min. Thus, it was found that lipid recoveries are nearly the same upon changing the static time, which means that static time has little effect on extraction efficiency. This result is consistent with a previous study.24 Thus, 5 min is sufficient for extracting lipids under the high temperature and pressure of ASE. Moreover, increased extraction times under high temperature and pressure could lead to partial degradation of some components. Since the longer contacting time was not found to not benefit lipid recovery, a shorter static time (5 min) was chosen as the optimal condition for this system.
3.1.4 Effect of static cycle on lipid recovery using ASE method. The static cycle is the number of times the static heating and rinsing steps are implemented. In the present study, the positive correlation found between static cycle on the lipid recovery is shown in Fig. 1D. As the number of static cycles increased, the lipid recovery was also enhanced. When static time was 2, the resulting lipid content was low at only 22.9 ± 0.02%. As static cycle increased to 4, the lipid content increased to 25.1 ± 0.1%. However, upon further increase of static cycles to 5, no obvious improvement in lipid recovery was observed. Considering the total time and the efficiency of the extraction process, a static cycle number of 4 was chosen as the optimum value for this system.
3.2 Comparison of optimized ASE method and conventional manual method for lipid extraction across different four algae species
After the optimal ASE operating conditions were determined using commercially purchased Chlorella powder, a comparison of the optimized ASE method and conventional manual method for lipid extraction were examined across four algae species: C. vulgaris, C. sorokiniana, C. zofingiensis and Nannochloropsis gaditana. These strains were cultivated photoautotrophically in 3 L batch cultures, then harvested and lyophilized for lipid extraction.
The lipid recovery using optimal ASE method and conventional manual extraction method are shown in Fig. 2. The ASE extraction method showed higher efficiencies than manual extraction across all the four algae strains. It was found to extract 6.9% more lipid from dry biomass of C. vulgaris than conventional manual extraction. Previous literature indicated that the lipid content from C. vulgaris can be roughly 10% by dry weight using autoclaving and microwave oven method, whereas the bead-beating, sonication and osmotic shock showed lower efficiencies.17 These prior results are all lower than the value obtained in the present study. The ASE method also showed a higher efficiency for C. sorokiniana than conventional manual extraction at 25.8 ± 1.4% of dry biomass. In the literature, total lipid content from C. sorokiniana analyzed by the gravimetric analysis with chloroform and methanol solvents (2
:
1, v/v) was 23.5% of dry biomass,38 and it was slightly lower than using the ASE method in this study. Conventional manual extraction method for C. zofingiensis was 8.8 ± 2.1%, 1.7-fold lower than the ASE method. For Nannochloropsis gaditana, the lipid content was found to be 29.8 ± 2.1% of dry biomass using ASE compared to 21.3 ± 3.1% using conventional manual extraction method, also higher than that reported in the literature using the Bligh and Dyer method at 25.3%.39
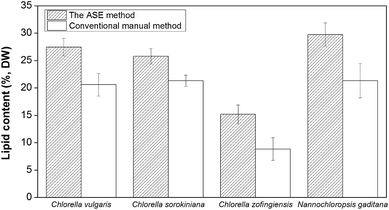 |
| Fig. 2 Comparison of ASE and conventional manual extraction on lipid recovery across Chlorella vulgaris, Chlorella sorokiniana, Chlorella zofingiensis and Nannochloropsis gaditana. Reaction conditions: methanol/chloroform (2 : 1, v/v), extraction temperature of 100 °C, static time of 5 min, static cycle number of 4. Error bars designate standard deviation from the average of duplicates. | |
3.3 Comparison of individual FAME recovery using ASE and conventional manual extraction across different four algae species
In order to evaluate the potential of the four strains as candidates for biodiesel production, detailed FAME compositions were elucidated in Fig. 3.
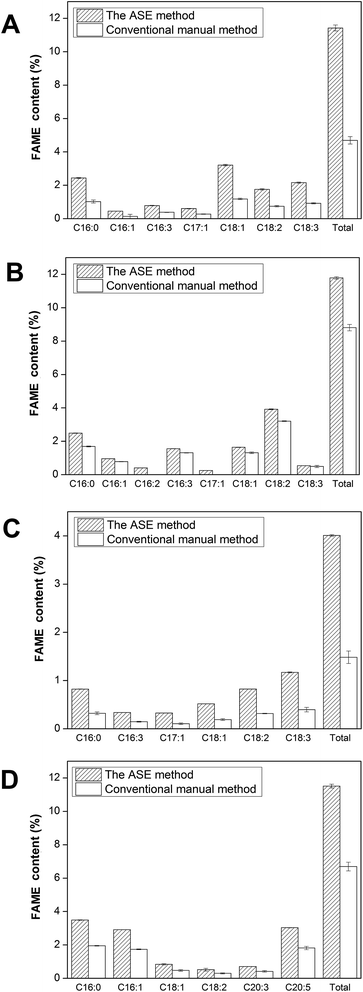 |
| Fig. 3 Comparison of ASE and conventional manual extraction on individual FAME recovery from (A) Chlorella vulgaris (B) Chlorella sorokiniana (C) Chlorella zofingiensis (D) Nannochloropsis gaditana. Error bars designate standard deviation from the average of duplicates. | |
3.3.1 Individual FAME content of C. vulgaris. ASE extraction was performed followed by GC analysis of FAME and the FAME contents of C. vulgaris using the ASE method and conventional manual extraction are shown in Fig. 3A. Sixteen- to eighteen-carbon fatty acids with no more than three degrees of unsaturation were observed in C. vulgaris grown photoautotrophically. The total FAME content obtained using the ASE extraction method followed by transesterification was 11.4 ± 0.2% of dry biomass, which is 2.4-fold higher than that using conventional manual extraction followed by transesterification. The ASE extraction method also exhibited a higher recovery for each individual FAME compared to conventional manual extraction method. The predominant FAME compositions in C. vulgaris were found to be C16:0 and C18:1 at 2.4 ± 0.02% and 3.2 ± 0.04% of dry biomass, respectively, from ASE extracted materials and the contents were at least 2.4- to 2.7-fold higher in extracted materials by conventional manual extraction. Previous research has shown that alternative treatment methods including high pressure steaming (HPS), similar to ASE, can alter the levels of FAME obtained.40
3.3.2 Individual FAME content of C. sorokiniana. The FAME compositions of C. sorokiniana are shown in Fig. 3B. The total FAME content was 11.8 ± 0.1% of dry biomass from the ASE extraction followed by transesterification, which is 1.3-fold higher than that from conventional manual extraction followed by transesterification. Small amounts of C16:2 and C17:1 were found using ASE extraction, but were not recovered by conventional manual extraction. Higher percentages of individual FAMEs were also obtained from the ASE extraction compared to conventional manual extraction, while the difference was not as pronounced in C. vulgaris. The principal FAME components in C. sorokiniana were found to be C16:0 and C18:2, accounting for 2.5 ± 0.01% and 3.9 ± 0.03% of dry biomass, respectively, from ASE extraction. The FAME profiles are quite similar to the results reported by Rosenberg et al.7Compared to C. vulgaris, the total FAME content of C. sorokiniana was similar, with 11.8 ± 0.1% and 11.4 ± 0.2% of dry biomass, respectively. Interestingly, C. sorokiniana exhibited greater enrichment in C18:2 relative to C18:1 and more unsaturated FAME such as C16:1, C16:3, C18:2 than C. vulgaris.
3.3.3 Individual FAME content of C. zofingiensis. The FAME composition of C. zofingiensis is shown in Fig. 3C. The ASE extraction clearly generated more total and individual FAME than conventional manual extraction for C. zofingiensis. The total FAME content was 2.7-fold higher with ASE extraction than with conventional manual extraction. C. zofingiensis produced C18:3, C18:2 and C16:0 as the principal FAME, which is a different distribution than the other Chlorella strains. In addition, a higher relative proportion of unsaturated FAME was produced in C. zofingiensis. The total FAME content of C. zofingiensis obtained using the ASE method was 2.9- and 3.0-fold lower compared to C. vulgaris and sorokiniana, respectively. Kobayashi et al.41 also observed that the FAME content of C. zofingiensis under ambient CO2 was only around 3% by dry weight—much lower than Chlorella vulgaris—and the study deduced that C. zofingiensis requires higher concentrations of nutrients in the medium to support its growth and accumulate more FAME.
3.3.4 Individual FAME content of Nannochloropsis gaditana. Nannochloropsis gaditana is another strain widely used to generate lipids and a candidate for the production of valuable PUFAs. A comparison of the FAME profiles from ASE and conventional manual extraction is shown in Fig. 3D. ASE extraction resulted in more total and individual FAME than observed with the three other Chlorella species. The total FAME recovered from ASE extraction was 11.5 ± 0.1% of dry biomass, while only 6.7 ± 0.3% of dry biomass was obtained by manual extraction. The predominant FAME in Nannochloropsis gaditana were found to be C16:0, C16:1 and C20:5.It should be noted that 3.0 ± 0.01% of the dry Nannochloropsis gaditana biomass was found to be all-cis-5,8,11,14,17-eicosapentaenoic acid (EPA) using ASE extraction—higher than that recovered by manual extraction. Previously, Pieber et al. achieved an EPA content of 3.7% of dry biomass from Nannochloropsis oculata using ASE method with ethanol as the extraction solvent.29 The study maintained a moderately lower temperature of 60 °C for ASE cycles recovering EPA and claimed that further optimization of ASE conditions could increase lipid recovery. Conversely, research by Islam et al. employed more optimal elevated temperatures between 90 and 120 °C with ASE for extraction of lipids that are amenable to biodiesel production, but not polyunsaturated fatty acids.42 In the current study, our optimized process was able to utilize high temperatures for efficient extraction of lipids while simultaneously yielding high levels of PUFAs such as EPA. The efficiency of supercritical CO2 extraction at optimized conditions from Nannochloropsis granulate was only 1.3% of dry biomass reported by Bjornsson et al.43 This demonstrates that ASE method has a potential for commercial extraction of microalgal PUFAs and is potentially superior to supercritical CO2 extraction method.
Of the four microalgae stains, C. vulgaris and C. sorokiniana appear to be good candidates for biodiesel production and Nannochloropsis gaditana has the potential to be used for EPA production.
4. Conclusions
The ASE method is an effective technique to extract lipids from algal biomass. Optimal ASE operating parameters for Chlorella powder were found to be: an extraction solvent system of 2
:
1 methanol
:
chloroform by volume, an extraction temperature of 100 °C, static time of 5 min, a static cycle number of 4. Compared with conventional manual extraction method, the ASE method can recover more lipid and FAME from C. vulgaris, C. sorokiniana, C. zofingiensis and Nannochloropsis gaditana. Furthermore, ASE showed the capacity to extract a significant component of the Nannochloropsis gaditana lipid fraction as EPA.
While ASE has shown to be efficient for the extraction of lipids and PUFAs such as EPA on the bench-top, commercial implementation of this approach at large scale will have to consider the energy input and other cost considerations of using the ASE. A major advantage of the ASE is that only minutes are needed for ASE method due to automated steps, whereas other extraction method such as sonication and Soxhlet can take hours to extract the target lipid. Also, the ASE does not require completely dry samples44 and this could reduce the number of dewatering steps applied prior to this method. Nonetheless, the use of ASE for algal lipid extraction in the present study support its utility at the laboratory scale for tasks ranging from strain selection based on total lipid content to detailed assessments of algal species based on fatty acid composition. Future work with ASE should examine additional parameters and applications in order to identify the optimal conditions for using the ASE for research, development, and commercial applications.
Acknowledgements
The authors gratefully acknowledge the financial support from grant number NSF-EFRI-1332344 from the National Science Foundation (MJB), DOE DE SC0012658 grant (MJB), NSF CBET-1236691 and a fellowship to JNR from the Johns Hopkins Environment, Energy, Sustainability & Health Institute (E2SHI, http://www.e2shi.jhu.edu/). Partial support was also provided by the Natural Science Foundation of Jiangsu universities (11KJA480001) and the national Natural Science Foundation of China (31170537). And this work was also supported by the Priority Academic Program Development of Jiangsu Higher Education Institutions (PAPD).
References
- L. Gouveia and A. C. Oliveira, J. Ind. Microbiol. Biotechnol., 2009, 36, 269–274 CrossRef CAS PubMed.
- Y. Chisti, Biotechnol. Adv., 2007, 25, 294–306 CrossRef CAS PubMed.
- T. M. Mata, A. A. Martins and N. S. Caetano, Renewable Sustainable Energy Rev., 2010, 14, 217–232 CrossRef CAS.
- J. N. Rosenberg, V. H. Oh, G. Yu, B. J. Guzman, G. A. Oyler and M. J. Betenbaugh, in Handbook of Marine Microalgae, ed. S. K. Kim, Academic Press, Boston, 2015, pp. 331–352 Search PubMed.
- Y. X. Li, F. J. Zhao and D. D. Yu, Braz. Arch. Biol. Technol., 2015, 58, 462–467 CrossRef CAS.
- M. Morita, Y. Watanabe and H. Saiki, Appl. Biochem. Biotechnol., 2000, 87, 203–218 CrossRef CAS PubMed.
- J. N. Rosenberg, N. Kobayashi, A. Barnes, E. A. Noel, M. J. Betenbaugh and G. A. Oyler, PLoS One, 2014, 9, e92460 Search PubMed.
- P.-F. Ip and F. Chen, Process Biochem., 2005, 40, 733–738 CrossRef CAS.
- Y. Ma, Z. Wang, C. Yu, Y. Yin and G. Zhou, Bioresour. Technol., 2014, 167, 503–509 CrossRef CAS PubMed.
- P. C. Hallenbeck, M. Grogger, M. Mraz and D. Veverka, Bioresour. Technol., 2015, 184, 161–168 CrossRef CAS PubMed.
- A. San Pedro, C. González-López, F. Acién and E. Molina-Grima, Bioresour. Technol., 2014, 169, 667–676 CrossRef CAS PubMed.
- M. Cooney, G. Young and N. Nagle, Sep. Purif. Rev., 2009, 38, 291–325 CrossRef CAS.
- V. Astrid, W. Christian, G. Reimund, S. Rosmarie and S. Jürgen, Chem. Phys. Lipids, 2008, 150, 143–155 Search PubMed.
- H. Wu and X. Miao, Bioresour. Technol., 2014, 170, 421–427 CrossRef CAS PubMed.
- M. J. Ramos, C. M. Fernández, A. Casas, L. Rodríguez and Á. Pérez, Bioresour. Technol., 2009, 100, 261–268 CrossRef CAS PubMed.
- I. A. Nascimento, I. T. D. Cabanelas, J. N. d. Santos, M. A. Nascimento, L. Sousa and G. Sansone, Algal Res., 2015, 8, 53–60 CrossRef.
- J.-Y. Lee, C. Yoo, S.-Y. Jun, C.-Y. Ahn and H.-M. Oh, Bioresour. Technol., 2010, 101, S75–S77 CrossRef CAS PubMed.
- P. Prabakaran and A. D. Ravindran, Lett. Appl. Microbiol., 2011, 53, 150–154 CrossRef CAS PubMed.
- D. Luthria, D. Vinjamoori, K. Noel and J. Ezzell, in Oil Extraction and Analysis: Critical Issues and Comparative Studies, ed. D. Luthria, AOCS, Champaign, IL, 2004, pp. 25–38 Search PubMed.
- K. Pilaniya, H. K. Chandrawanshi, U. Pilaniya, P. Manchandani, P. Jain and N. Singh, J. Adv. Pharm. Technol. Res., 2010, 1, 302–310 CrossRef CAS PubMed.
- K. Schäfer, Anal. Chim. Acta, 1998, 358, 69–77 CrossRef.
- B. E. Richter, B. A. Jones, J. L. Ezzell, N. L. Porter, N. Avdalovic and C. Pohl, Anal. Chem., 1996, 68, 1033–1039 CrossRef CAS.
- J. R. Denery, K. Dragull, C. S. Tang and Q. X. Li, Anal. Chim. Acta, 2004, 501, 175–181 CrossRef CAS.
- M. Herrero, P. J. Martín-Álvarez, F. J. Señoráns, A. Cifuentes and E. Ibáñez, Food Chem., 2005, 93, 417–423 CrossRef CAS.
- H. W. Nichols and H. C. Bold, J. Phycol., 1965, 1, 34–38 CrossRef.
- D.-Y. Kwon, J.-H. Kwon and G.-J. Jo, Desalin. Water Treat., 2013, 52, 1007–1013 CrossRef.
- E. G. Bligh and W. J. Dyer, Can. J. Biochem. Physiol., 1959, 37, 911–917 CrossRef CAS PubMed.
- P. Hidalgo, G. Ciudad and R. Navia, Bioresour. Technol., 2016, 201, 360–364 CrossRef CAS PubMed.
- S. Pieber, S. Schober and M. Mittelbach, Biomass Bioenergy, 2012, 47, 474–482 CrossRef CAS.
- W. Mulbry, S. Kondrad, J. Buyer and D. Luthria, J. Am. Oil Chem. Soc., 2009, 86, 909–915 CrossRef CAS.
- E. Ryckebosch, K. Muylaert and I. Foubert, J. Am. Oil Chem. Soc., 2012, 89, 189–198 CrossRef CAS.
- J. B. Guckert, K. E. Cooksey and L. L. Jackson, J. Microbiol. Methods, 1988, 8, 139–149 CrossRef CAS.
- J. Jones, S. Manning, M. Montoya, K. Keller and M. Poenie, J. Am. Oil Chem. Soc., 2012, 89, 1371–1381 CAS.
- G. Young, F. Nippgen, S. Titterbrandt and M. J. Cooney, Sep. Purif. Technol., 2010, 72, 118–121 CrossRef CAS.
- Y.-H. Kim, Y.-K. Choi, J. Park, S. Lee, Y.-H. Yang, H. J. Kim, T.-J. Park, Y. Hwan Kim and S. H. Lee, Bioresour. Technol., 2012, 109, 312–315 CrossRef CAS PubMed.
- S. Dufreche, R. Hernandez, T. French, D. Sparks, M. Zappi and E. Alley, J. Am. Oil Chem. Soc., 2007, 84, 181–187 CrossRef CAS.
- E. D. Revellame, R. Hernandez, W. French, W. E. Holmes, T. J. Benson, P. J. Pham, A. Forks and R. Callahan Ii, RSC Adv., 2012, 2, 2015–2031 RSC.
- B. D. Wahlen, R. M. Willis and L. C. Seefeldt, Bioresour. Technol., 2011, 102, 2724–2730 CrossRef CAS PubMed.
- B. P. Nobre, F. Villalobos, B. E. Barragán, A. C. Oliveira, A. P. Batista, P. A. S. S. Marques, R. L. Mendes, H. Sovová, A. F. Palavra and L. Gouveia, Bioresour. Technol., 2013, 135, 128–136 CrossRef CAS PubMed.
- A.-M. Aguirre and A. Bassi, Bioresour. Technol., 2014, 164, 136–142 CrossRef CAS PubMed.
- N. Kobayashi, A. Barnes, T. Jensen, E. Noel, G. Andlay, J. N. Rosenberg, M. J. Betenbaugh, M. T. Guarnieri and G. A. Oyler, Bioresour. Technol., 2015, 198, 246–255 CrossRef CAS PubMed.
- M. A. Islam, R. J. Brown, I. O'Hara, M. Kent and K. Heimann, Energy Convers. Manage., 2014, 88, 307–316 CrossRef CAS.
- W. J. Bjornsson, K. M. MacDougall, J. E. Melanson, S. J. B. O'Leary and P. J. McGinn, J. Appl. Phycol., 2012, 24, 547–555 CrossRef CAS.
- B. E. Richter, J. Chromatogr. A, 2000, 874, 217–224 CrossRef CAS PubMed.
Footnote |
† These authors contributed equally to this work. |
|
This journal is © The Royal Society of Chemistry 2016 |
Click here to see how this site uses Cookies. View our privacy policy here.