DOI:
10.1039/C5RA23041A
(Paper)
RSC Adv., 2016,
6, 5214-5222
Hydrogenation of lignin-derived phenolic compounds over step by step precipitated Ni/SiO2†
Received
2nd November 2015
, Accepted 29th December 2015
First published on 6th January 2016
Abstract
The harsh reaction conditions for the valorization of lignin-derived phenolic compounds considerably limit the efficient utilization of the lignin derivatives. Here, we put forward a high efficient and selective hydrogenation process for phenolic compounds at a mild condition over step by step precipitated Ni/SiO2 catalyst. The properties of the Ni/SiO2 catalysts by different preparation methods were detailedly compared using various characterization measurements. Catalytic activity of the catalysts was tested by the hydrogenation of guaiacol, and the results showed that guaiacol could be completely converted into cyclohexanol with 99.9% selectivity at 120 °C, 2 MPa H2 atmosphere for 2 h. Other typical lignin-derived phenolic compounds also had excellent hydrogenation performance and great energy efficiency. Catalyst characterization results demonstrated that the high catalytic activity of the step by step precipitated Ni/SiO2 was mainly ascribed to its polyporous spherical structure, which led to the large specific surface area and high nickel dispersion. The appropriate acidity of the catalyst also promoted the catalytic performance significantly. Furthermore, the catalyst exhibited an excellent recyclability, where no significant loss of the catalytic activity was showed out after 3 runs.
1. Introduction
With the depletion of petroleum deposition and the increasing environmental problems, lignin, the byproduct of the biomass refinery, is attracting global attention as an important renewable feedstock for the production of fuels, chemicals and energy.1 Due to its 4-propenyl-phenols units interlinked through C–O–C ether covalent bonds,2 lignin can be broken down into phenolic monomers, dimers and oligomers by means of catalytic and thermochemical processes.3–6 These phenolic compounds are potential to be high grade fuels and chemicals. However, owing to the high oxygen content, corrosivity and immiscibility,7 they cannot be directly used as biofuels. The unsaturated aromatic compounds have a poor chemical stability and are flexible to repolymerize, which will result in significant char formation.8,9 Therefore, the researches for lignin depolymerization products conversion to stable and high-value compounds have recently drawn unprecedented attention.
Guaiacol, as one of the most abundant products from the lignin depolymerization processes, is a popular model compound for phenolic monomer valorization study.10,11 It contains three kinds of C–O bonds for CAR–OH, CAR–OCH3 and CARO–CH3, which are typically and frequently found in various lignin derivatives.12 Wherein, the CAR–OCH3 is easy to cleave by hydrogenolysis,13,14 while the removement of the oxygen in CAR–OH is much harder. The hydrodeoxygenation (HDO) method was usually used for the upgrade of guaiacol.15–17 Sulfided CoMo and NiMo are the most common catalysts in the early time.18–20 While, the continuous addition of sulfur is required in the reactant stream to maintain the catalyst activity. Catalyst deactivation also occurs easily for the high coke formation, hydrothermal instability, and catalyst sintering. The noble metals receive widespread attention due to their scarce characteristic of the aromatic ring hydrogenation and anti-carbon deposition. For example, Lin's study21 demonstrated that 100% conversion of guaiacol could be obtained over Rh catalysts at 300 °C. The research group of Kou22–24 also had done lots of interesting work in this issue. They found that guaiacol could be completely converted into cycloalkane with Pd/C and H3PO4 catalysts at 250 °C. However, the high cost for these catalysts is inevitable, and the reaction conditions have yet to be much milder.
The HDO process involves the complete deoxygenation, and hydrocarbons such as benzene and cyclohexane were the desired products. The acid centres are needed to catalyze the deoxygenation and the high temperature is necessary for the high reaction activation energy.24,25 But, the use of a high temperature process is not suitable for the treatment of real lignin derived products because of the simultaneous existence of phenolic monomers and oligomers.9 They contain a large amount of unsaturated structures and can repolymerize at elevated temperature to produce the undesired char. Remarkably, the selective hydrogenation reaction is much easier due to its low reaction activation energy,23 which can realize even over the non-noble metal catalysts.14,26 The platform chemical compounds or high-value biofuel precursors, such as cyclohexanol, are prone to obtain at low temperature likewise. Moreover, the hydrogenation is very fast, in which the rate is several orders of magnitude higher than the deoxygenation reaction.24,25 Therefore, the selective hydrogenation process is potential, energy-saving and easy to realize.
In this work, a mild and high selective process for the hydrogenation of the lignin-derived phenolic compounds was proposed in the presence of different Ni/SiO2 catalysts. Results showed that the step by step precipitated Ni/SiO2 had a great performance on the hydrogenation of guaiacol and other typical phenolic compounds. Detailed characterization demonstrated that the structure of the catalysts influenced the catalytic activity significantly. This novel catalysis process is a quite promising technique for the upgrade of lignin-derived phenolic compounds, producing the platform chemical compounds or high-value biofuel precursors.
2. Experimental
2.1. Materials
Tetraethylorthosilicate (TEOS) and cetylhexadecyl trimethyl ammonium bromide (CTAB) were purchased from Da Mao chemical reagent factory. Ni(NO3)3 was provided by Tianjin Fu Chen chemical reagent factory. Poly-vinylpyrrolidone (PVP) was purchased from Shanghai Yuan Ju biotechnology Co., Ltd. Guaiacol was purchased from Sinopharm Chemical Reagent Co., Ltd. Other reagents were supplied by Jinhuada Chemical Reagent Co., Ltd. All reagents were analytic grade and were used without further purification.
2.2. Catalyst preparation
The step by step precipitated Ni/SiO2 catalyst was prepared by a modified method.27 In brief, 7.0 g Ni(NO3)3 and 1.0 g poly-vinylpyrrolidone (PVP) was added to a 700 ml ethanol–water (4
:
3) solution. After the solution was stirred for 5 min, 120 ml NH3·H2O (25 wt%) was injected dropwise under strong agitation. Then, 6.0 g hexadecyl trimethyl ammonium bromide (CTAB) was added, and the suspension was stirred for another 10 min. Subsequently, 20 ml tetraethylorthosilicate (TEOS) was injected into the suspension dropwise. Stirring vigorously for 72 h, the precipitation was filtrated, washed three times with distilled water and ethanol, and dried at 60 °C in air for 12 h. Then the catalyst was calcined at 500 °C for 6 h. After reduction in a slow H2 flow at 550 °C for 6 h, the step by step precipitated Ni/SiO2 catalyst sample was obtained. The method for synthesizing the co-precipitated Ni/SiO2 catalyst28 was similar with that for the step by step precipitated Ni/SiO2 catalyst, and only the addition order of reagents was changed, in which NH3·H2O was added after the injection of TEOS. The supported Ni/SiO2 catalyst was prepared by wet impregnation method.29 The SiO2 support was obtained by the hydrolysis of TEOS (20 ml) using 25 wt% NH3·H2O (120 ml) with the same treatment procedure as above. For convenience, the step by step precipitated Ni/SiO2, co-precipitated Ni/SiO2 and supported Ni/SiO2 catalysts were denoted as SS-Ni/SiO2, CP-Ni/SiO2 and SP-Ni/SiO2, respectively.
2.3. Catalyst characterization
The surface area and pore size distribution of the catalysts were carried out over a Quantachrome chemical adsorption instrument. In a typical process, the catalysts were degassed at 280 °C for 10 h prior to measurement, and the surface area was calculated by the Brunauer Emmett Teller (BET) method. Powder X-ray diffraction (XRD) patterns of the catalysts were measured using X-ray diffraction radiation (X Pert Pro MPD with Cu Kα (k = 0.154) radiation, Philip, the scanning angle (2θ) ranged from 10° to 80°). X-ray fluorescence (XRF) was conducted on the PHI-5300/ESCA instrument. Scanning electron microscope (SEM) images of the catalysts were obtained on a Hitach S-4800 instrument (10 kV). Transmission electron microscopy (TEM) and energy dispersive spectrometer (EDS) investigations were measured on a Jeol TEM-100CX instrument at 200 kV accelerating voltage. Hydrogen temperature programmed reduction (H2-TPR) and desorption (H2-TPD), as well as ammonia temperature programmed desorption (NH3-TPD) investigations were conducted in a same U-tube quartz reactor with a thermal conductivity detector (TCD). The Ni metal leaching of the catalyst in the decalin was determined by inductively coupled plasma-atomic emission spectrometry (ICP-AES, OPTIMA 8000). Fourier Translation Infrared Spectroscopy (FT-IR) was obtained on a Nicolet iS50 FT-IR spectrometer using KBr pelleting method.
2.4. Hydrogenation of lignin-derived phenolic compounds
All the hydrogenation reactions of lignin-derived phenolic compounds were carried out in a 50 ml stainless autoclave equipped with an electromagnetic driven stirrer. Typically, 0.1 g catalyst, 0.2 g phenolic compound and 20 ml decalin were added into the autoclave. After purging with hydrogen three times, the reactor was pressurized to 2 MPa at room temperature and heated to 120 °C for 2 h under vigorous stirring of 400 rpm. Repeated activity measurements were made and the error bars were determined and added to the reaction data.
The components of liquid products were identified by gas chromatography/mass spectrometry (GC-MS, Agilent 5890) equipped with a column of HP-INNOWAX, 30 m × 0.25 mm × 0.25 μm. The oven temperature was programmed as 60 °C hold 2 min, and then ramped up to 260 °C with 10 °C min−1 and hold for another 10 min. The injector was kept at 280 °C in split mode (5
:
1) with helium as the carrier gas. Quantitative analysis of the products was determined by a SHIMADZU GC 2014C with a flame ionization detector (FID) and a HP-INNOWAX column. The oven temperature program was the same as the GC-MS analysis. The conversion of phenolic model compounds and the product selectivity were calculated based on the following equations:
Conversion (%) = (molesin − molesout)/molesin × 100% |
Selectivity (%) = moles (product)i/∑moles (product) × 100% |
3. Results and discussion
3.1. Catalyst characterization
N2 adsorption/desorption isotherms of different Ni/SiO2 catalysts and SiO2 support were displayed in Fig. S1.† And their corresponding pore size distributions were also exhibited. Table 1 summarized the textural parameters of these catalysts. The preparation methods had a significant effect on the BET surface area and the pore property. The support had a surface area of 92.08 m2 g−1 and a total pore volume of 0.56 cm3 g−1. However, when it was impregnated with nickel, these values of obtained SP-Ni/SiO2 decreased to 67.63 m2 g−1 and 0.47 cm3 g−1 respectively. Meanwhile, the pore diameter did not change. The pore closure by the active phase precursor30,31 were considered to be responsible for it. As for the CP-Ni/SiO2, a declination of the pore volume was showed with the increase of both the surface area and the pore diameter, compared to the single support. The reason was considered to be that the Ni species were inlaid inside the SiO2 with bad closure of the pore. Moreover, it should be noted that both the surface area and the total pore volume of the SS-Ni/SiO2 were greatly increased to 450.75 m2 g−1 and 1.06 cm3 g−1, while the pore diameter decreased to 3.04 nm. This was due to the high mesoporous nature of the silica phase.32 The large surface area suggested its potential application in catalytic reaction.
Table 1 Surface properties of the different Ni/SiO2 catalysts
Catalystsa |
Surface areab (m2 g−1) |
Total pore volumec (cm3 g−1) |
Pore diameterc (nm) |
SS, step by step precipitated; CP, co-precipitated; SP, supported. MultiPoint Brunauer, Emmett &Teller (BET) method. Barrett, Joyner & Halenda (BJH) method. |
SS-Ni/SiO2 |
450.75 |
1.06 |
3.04 |
CP-Ni/SiO2 |
238.93 |
0.28 |
3.41 |
SP-Ni/SiO2 |
67.63 |
0.47 |
3.82 |
SiO2 |
92.08 |
0.56 |
3.82 |
The XRD patterns of the different Ni/SiO2 catalysts were exhibited in Fig. 1. Wherein, the patterns of CP-Ni/SiO2 and SP-Ni/SiO2 samples were very similar. The diffraction peaks at values of 44.3°, 51.4°, and 76.1° were showed, which were respective related to the crystal planes of (111), (200), and (220) of metallic nickel phase.33 And the peaks of SP-Ni/SiO2 sample were stronger, indicating the greater crystallinity and bigger crystal grain size. However, the SS-Ni/SiO2 sample was quite different. There was only a broad peak at about 22.3° that ascribed to the SiO2 phase, and no diffraction peaks belonging to Ni phase could be detected. Obviously, the SiO2 showed no crystallinity demonstrated that it existed as amorphous phase.32,34 While, based on the preparation method, the disappearance of Ni species was considered to result from that the Ni particles were quite tiny and well dispersed in the interior of the SiO2 phase.
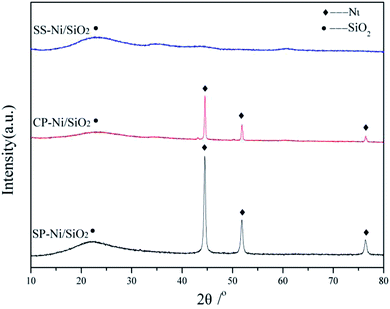 |
| Fig. 1 XRD patterns of the different Ni/SiO2 catalysts. | |
In order to confirm the high dispersion of Ni particles, the SEM and TEM measurements were used to observe the morphology of the different Ni/SiO2 catalysts. As showed in the SEM image of SS-Ni/SiO2 sample (Fig. 2a), the spherical composite was uniformly obtained with particle size of 200–400 nm, which was much larger compared with other reported Ni/SiO2 catalysts.35,36 They were exhibiting a relative homogeneous smooth external surface with porosity. The STEM images (Fig. 2b) exhibited that the small Ni particles with nanoscale were highly dispersed not only on the surface, but also in the interior of catalysts. This special structure was affected by the preparation procedure significantly. The tiny Ni particles were firstly precipitated and suspended in the solution. Afterward, the SiO2 precipitated and encapsulated many Ni particles, then the large composite spheres formed. Some Ni particles also attached to the surface of catalysts spontaneously. The EDS images (Fig. 2b) demonstrated the Ni particles were quite small and the size was nanoscale, which verified the high dispersion of the Ni phase in the SS-Ni/SiO2 sample. These phenomena explained well the large BET surface area (Table 1) and the disappearance of the Ni species diffraction peaks in the XRD pattern (Fig. 1). However, the other two Ni/SiO2 samples were quite different. As for CP-Ni/SiO2 (Fig. 2c), the Ni and SiO2 were coprecipitated, and the interconnected structure was clustered together, where the size was much smaller than SS-Ni/SiO2. Moreover, the SP-Ni/SiO2 sample showed that the Ni species were well supported on the SiO2 surface (Fig. 2d) and their average size was much larger than that of CP-Ni/SiO2. It explained well its stronger diffraction peaks in XRD patterns (Fig. 1). Remarkably, the aggregation of the Ni particles was obvious in the SP-Ni/SiO2 and CP-Ni/SiO2, but not in the SS-Ni/SiO2, which indicated that the Ni dispersion of SS-Ni/SiO2 was much better than the others.
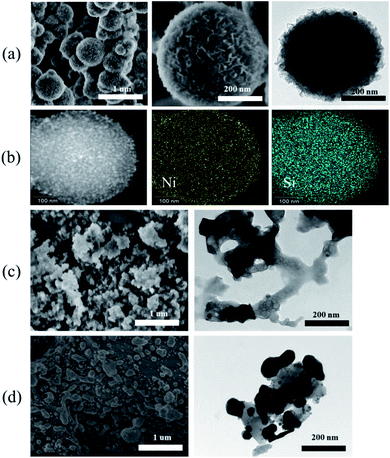 |
| Fig. 2 (a) SEM and TEM images of SS-Ni/SiO2, (b) STEM and EDS images of SS-Ni/SiO2, (c) SEM and TEM images of CP-Ni/SiO2, (d) SEM and TEM images of SP-Ni/SiO2. | |
The H2-TPR profiles of the different Ni/SiO2 catalysts were given in Fig. 3, in which a low-temperature peak centered at 445 °C and a high-temperature peak around 840 °C were observed. The reduction peak at low temperature was attributed to the reduction of NiO less interacted with the support, while the one at high temperature corresponded to the reduction of NiO under strong interaction with the support.37 The SP-Ni/SiO2 only exhibited a stronger low-temperature peak and the CP-Ni/SiO2 showed both the low-temperature and high-temperature peaks. This difference was resulted from the preparation methods, which made the CP-Ni/SiO2 have a strong interaction with the support.38 Particularly, the SS-Ni/SiO2 catalyst only had a broad reduction band between 600 °C and 880 °C. This was probably due to the formation of the nickel silicate species,34 which were strongly interacted with the support and hard to reduce.
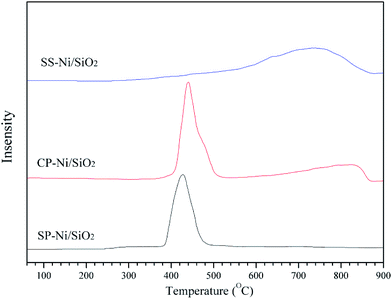 |
| Fig. 3 H2-TPR profiles of the different Ni/SiO2 catalysts. | |
To make an accurate comparison of nickel dispersion, H2-TPD measurements for reduced different Ni/SiO2 catalysts were carried out as represented in Fig. S2.† Generally, there were two hydrogen desorption regions: the low temperature at 250 °C was attributed to hydrogen desorbed from the active metal species, and the high temperature at 550 °C was related to hydrogen desorbed from subsurface layers or to spillover hydrogen.39,40 As observed, the SP-Ni/SiO2 had an obvious low temperature peak, while, the SS-Ni/SiO2 and CP-Ni/SiO2 had a high temperature peak. The peak intensity of SS-Ni/SiO2 was much higher than that of SP-Ni/SiO2 and CP-Ni/SiO2, which indicated the high Ni dispersion of SS-Ni/SiO2. The high peak intensity also demonstrated that the hydrogen desorption from subsurface layers or hydrogen spillover in SS-Ni/SiO2 sample occurred more seriously.39,40 The quantitative analysis of hydrogen uptake by exposed nickel species of the catalysts was conducted based on the H2-TPD peaks area. As showed in Table 2, although the SS-Ni/SiO2 catalyst had the lowest Ni loading (average 14.2%), its H2 uptake was the most, with 360.2 μmol g−1. The nickel dispersion was also calculated out. The SS-Ni/SiO2 catalyst exhibited 29.8% nickel dispersion, which was higher than that of CP-Ni/SiO2 and SP-Ni/SiO2. This was also in well accordance with the XRD patterns (Fig. 1) and STEM, EDS results (Fig. 2).
Table 2 The dispersion of the different reduced Ni/SiO2 catalysts
Catalystsa |
Ni loading (wt%) |
H2 uptakeb (μmol g−1) |
Nickel dispersion (%) |
XRF |
EDS |
SS, step by step precipitated; CP, co-precipitated; SP, supported. Calculated from peak area of H2-TPD profiles. |
20% SS-Ni/SiO2 |
15.85 |
12.56 |
360.2 |
29.8 |
20% CP-Ni/SiO2 |
17.71 |
14.32 |
150.1 |
11.0 |
20% SP-Ni/SiO2 |
18.13 |
15.74 |
137.1 |
9.5 |
The NH3-TPD experiments were also carried out and depicted in Fig. 4. The CP-Ni/SiO2 and SP-Ni/SiO2 exhibited only one weak low-temperature desorption peak, which indicated that they had only weak acid sites. However, two desorption peaks arising from NH3 could be detected in the SS-Ni/SiO2 profile. According to the desorption temperature and the corresponding classification of acid sites,34,41 the SS-Ni/SiO2 catalyst had not only weak, but also strong acid sites with desorption temperature around 175 °C and 400 °C, respectively. It demonstrated that the acid sites of the SS-Ni/SiO2 were much stronger than that of the CP-Ni/SiO2 and SP-Ni/SiO2 catalysts, although these catalysts with SiO2 support were much weaker than those with acid supports, such as Ni/HZSM-5,25 Ni/Al2O3.34 And obviously, the amount of desorbed NH3 represented by the area of desorption peaks also indicated that the SS-Ni/SiO2 catalyst had much more acid sites than the CP-Ni/SiO2 and SP-Ni/SiO2. This was probably resulted from the unreduced nickel silicate, which could form the [Ni(NH3)5]2+ ions.42–44 The explanation correlated well with the strong interaction between nickel and silica in the SS-Ni/SiO2 catalyst, which was testified by the H2-TPR profile in Fig. 3.
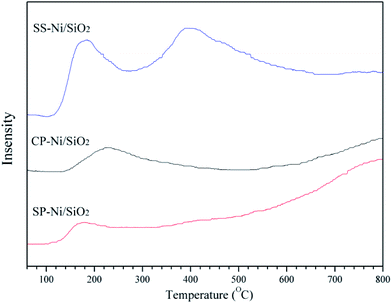 |
| Fig. 4 NH3-TPD profiles of the different Ni/SiO2 catalysts. | |
The FT-IR analysis of the different Ni/SiO2 catalysts was showed in Fig. S3.† The large band in the range 3500–3400 cm−1 corresponded to the molecular water or strongly H-bonded SiOH group. The band 1620 cm−1 was attributed to the vibrations of HOH bond deformation, 1100 cm−1 corresponding to the stretching vibration of Si–O–Si bond, 800 cm−1 corresponding to tetraedric SiO4, 450 cm−1 attributed to the vibrations of Si–O bond.45,46 The FT-IR profiles of different Ni/SiO2 catalysts exhibited the similar peak types, while, the distribution of groups was great different. Wherein, the SS-Ni/SiO2 catalyst had a similar property with the sol–gel catalyst, especially on the existence of a large amount of hydroxyl group.46 And the peak intensity of this group in the SS-Ni/SiO2 catalyst was much higher than that in the CP-Ni/SiO2 and SP-Ni/SiO2 catalysts.
3.2. Adsorption ability of guaiacol
The catalysts adsorption ability of the substrate was tested. The adsorption curve of guaiacol on different Ni/SiO2 catalysts (Fig. 5) showed the SS-Ni/SiO2 catalyst had much stronger adsorption ability than the others. Wherein, guaiacol had been substantially adsorbed to the surface of SS-Ni/SiO2 catalyst in the initial 20 min, and reached to the saturated state with the quantity of about 0.9 mmol g−1. While, as for the CP-Ni/SiO2 and SP-Ni/SiO2, their adsorption ability was much weaker, and the quantity was only about 0.2 mmol g−1. This difference was probably due to the specific surface area of catalysts (Table 1), as well as the amount of hydroxyl groups over the catalysts (Fig. S3†), which had strong absorption to the aromatic hydroxyl groups of guaiacol.47,48
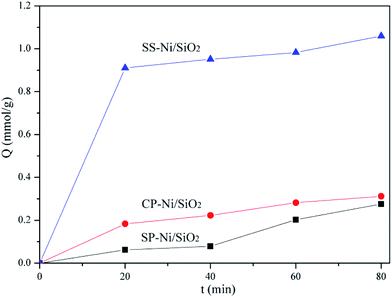 |
| Fig. 5 Adsorption curve of guaiacol on the different Ni/SiO2 catalysts. | |
3.3. Catalytic hydrogenation of guaiacol
The catalytic activity of the different Ni/SiO2 catalysts on the hydrogenation of guaiacol was investigated (Table 3). In order to calculate the turnover frequency (TOF) values, the H2-TPD profiles of different SS-Ni/SiO2 catalysts were extra measured (Fig. S4†) and the Ni dispersions of all the samples were given out (Table S1†). As a blank control, the single support SiO2 had not any effect on the hydrogenation reaction at 120 °C. However, over the SS-Ni/SiO2 catalyst, the guaiacol could be converted into cyclohexanol with 99.4% conversion and 5.26 h−1 TOF value, performing much better than those over the SP-Ni/SiO2 (5.8% of conversion) and the CP-Ni/SiO2 (3.4% of conversion). CH3OH was detected, deriving from the efficient hydrogenolysis of the –OCH3 side chain of guaiacol, which was promoted by the synergistic effect of acid sites (Fig. 4) and Ni particles.25 While, this work focused on the selective hydrogenation products, therefore the CH3OH yield was not presented in the following discussion.
Table 3 The effects of different catalysts on the guaiacol hydrogenationa
Catalystb |
Temperature (°C) |
Conversionc (%) |
TOF (h−1) |
Reaction condition: 20 ml decalin solvent, 0.2 g guaiacol, 0.1 g catalyst, 3 MPa H2, 3 h. CP, co-precipitated; SP, supported; SS, step by step precipitated. The hydrogenation product was cyclohexanol and the selectivity was 99.9%. |
SiO2 |
120 |
0 |
0 |
20% CP-Ni/SiO2 |
120 |
3.4 |
0.49 |
20% SP-Ni/SiO2 |
120 |
5.8 |
0.96 |
20% SS-Ni/SiO2 |
120 |
99.4 |
5.26 |
15% SS-Ni/SiO2 |
120 |
89.2 |
5.98 |
10% SS-Ni/SiO2 |
120 |
68.4 |
6.01 |
5% SS-Ni/SiO2 |
120 |
39.4 |
5.46 |
As for the guaiacol conversion into cyclohexanol, Escalona et al. had reported their study at the reaction temperature of 300 °C, with the cyclohexanol selectivity of 60%.11 Also, Guvenatam et al. had successfully realized the reaction at the temperature of 200 °C over noble metal catalyst, but the conversion of guaiacol only reached 80%, with the cyclohexanol selectivity of 70%.12 Comparatively, the reaction condition in this process was milder and the performance was more excellent. According to the detailed characterization of the catalysts above, the high catalytic activity of SS-Ni/SiO2 was mainly ascribed to its polyporous spherical structure (Fig. 2), which led to the large specific surface area (Table 1) and high nickel dispersion (Table 2). The appropriate acidity of the catalyst (Fig. 4), as well as the strong guaiacol adsorption ability (Fig. 5), also promoted the catalytic performance significantly. Moreover, the silica phase was polyporous with a huge amount of 3.04 nm mesopores (Table 1), which allowed guaiacol molecules (molecular diameter 0.67 nm (ref. 49)) to pass through and interact with active sites for hydrogenolysis and hydrogenation. Table 3 also showed that the Ni content of the catalyst was a crucial factor for guaiacol hydrogenation. The higher Ni content meant more hydrogenation active sites and more acid sites (the unreduced nickel silicate). Therefore, with the increase of Ni content, the guaiacol conversion was gradually increased, and almost 100% conversion could be achieved over 20% SS-Ni/SiO2.
With the 20% SS-Ni/SiO2 catalyst, the influences of reaction temperature, reaction time, hydrogen pressure and catalyst dosage had also been investigated (Fig. 6). Fig. 6a showed that reaction temperature remarkably affected the guaiacol hydrogenation process. The conversion of guaiacol raised up gradually, with the increase of the reaction temperature. For example, 63.7% guaiacol was hydrogenated at 100 °C, however, when it reached to 120 °C, the guaiacol was completely converted into cyclohexanol with 99.9% selectivity. This was a mild temperature condition for the guaiacol hydrogenation process in comparison with the reported literatures.11–14,25 The reaction time also had a pronounced effect on the conversion of guaiacol (Fig. 6b). In the investigated time range of 0.75 to 3.0 h, the conversion of guaiacol gradually increased from 33.1% to 99.4%. Remarkably, the guaiacol conversion suffered a sharply increase from 49.1% to 98.9% when the reaction time was prolonged from 1.5 to 2.0 h. The induction period of the heterogeneous catalyst were considered to be the main reasons for this significant increase.50 This hydrogenation process was H2 consumed, therefore, the increase of H2 pressure favored the guaiacol conversion. When the pushed H2 pressure was more than 2 MPa, the increase of the performance was insignificant because almost complete conversion of the feedstock (100%) was achieved (Fig. 6c). And thus, 2 MPa hydrogen pressure was obviously the most suitable for this hydrogenation process. Fig. 6d showed the influence of catalyst dosages. Obviously, the augment of catalyst dosages meant more catalytic activity centers, resulting in higher guaiacol conversion. For instance, 79.9% conversion was shown up over 0.06 g SS-Ni/SiO2 catalyst, while nearly 100% conversion was obtained when the catalyst dosage increased to 0.1 g.
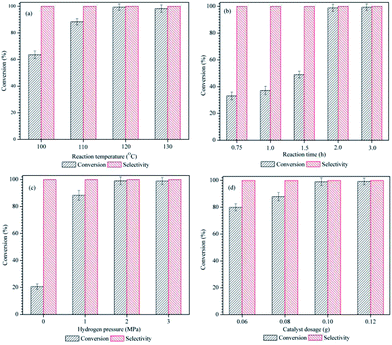 |
| Fig. 6 Effects of different (a) reaction temperature, (b) reaction time, (c) hydrogen pressure and (d) catalyst dosage on guaiacol hydrogenation.a aReaction condition: (a) 0.2 g guaiacol, 0.1 g 20% SS-Ni/SiO2, 20 ml decalin, 3 MPa H2, 3 h; (b) 0.2 g guaiacol, 0.1 g 20% SS-Ni/SiO2, 20 ml decalin, 3 MPa H2, 120 °C; (c) 0.2 g guaiacol, 0.1 g 20% SS-Ni/SiO2, 20 ml decalin, 120 °C, 2 h; (d) 0.2 g guaiacol, 20 ml decalin, 2 MPa H2, 120 °C, 2 h. | |
3.4. Hydrogenation of other typical phenolic compounds
In an extension to guaiacol, a series of other typical lignin-derived phenolic compounds with various structures were investigated under the optimized condition (120 °C, 2 MPa H2 for 2 h) over the SS-Ni/SiO2 catalyst. Table 4 listed the hydrogenation results of seven kinds of lignin-derived phenolic compounds in detail. As the representative phenolic monomers, phenol, p-cresol and benzyl alcohol were all completely converted into the corresponding saturated alcohol with the selectivity up to 99.9% through the aromatic ring hydrogenation (Table 4, entry 1–3). The catalyst also performed well on the hydrogenation of 2,4-dimethyl-phenol, with 2,4-dimethylcyclohexanol as the major product and a small amount of saturated alkane obtained (Table 4, entry 4). Unexpectedly, 5-eugenol and vanillin could not achieve the hydrogenation of the aromatic ring, and that of the carbon double bond and aldehyde group occurred instead. 2-Methoxy-4-propyl-phenol and 2-methoxy-4-methyl-phenol were their major products, respectively (Table 4, entry 5 and 6). It indicated that the species and position of the substituents in aromatic ring affected the chemical property greatly. And the carbon double bond and aldehyde group were more active than the aromatic ring during this process, which accorded well with the results of our previous study.51 Moreover, the diphenyl ether, as the example of dimeric phenolic compounds, was mostly converted into hydrogenation product rather than cyclohexane, which was much different from the previous study of Zhang52 (Table 4, entry 7). The much weaker acidity of the SS-Ni/SiO2 compared with Ru/HZSM-5, was considered to be the main reason for this difference.
Table 4 Hydrogenation of other lignin-derived phenolic compounds over the SS-Ni/SiO2 catalysta
Entry |
Substrates |
Conversion (%) |
Selectivity (%) |
Condition: 0.2 g phenolic compounds, 0.1 g 20% SS-Ni/SiO2 catalyst, 20 ml decalin, 2 MPa H2, 120 °C, 2 h. |
1 |
 |
99.9 |
 |
|
|
2 |
 |
97.8 |
 |
|
|
3 |
 |
98.5 |
 |
|
|
4 |
 |
96.4 |
 |
 |
 |
5 |
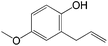 |
99.9 |
 |
 |
|
6 |
 |
99.8 |
 |
 |
 |
7 |
 |
95.8 |
 |
 |
 |
In summary, the typical lignin-derived phenolic compounds could be effectively converted into saturated alcohol with high yields, which suggested that the catalytic hydrogenation reaction routine of the phenolic compounds was highly atom economic and energy efficient, and the SS-Ni/SiO2 catalyst was potential to apply widely for the upgrade of diverse phenolic compounds.
3.5. Catalyst recycle
The recyclability of the SS-Ni/SiO2 catalyst was also investigated carefully. As shown in Fig. S5,† the catalyst exhibited an excellent recyclability for the first 3 runs, where no significant decrease of the activity loss was detected (more than 99.4%). However, in the fourth run, the conversion of guaiacol was slight decreased to 92.1%. The SEM images of the fresh and recovered SS-Ni/SiO2 (Fig. S6†) demonstrated that there was no significant change on the particle size and morphology. However, a slight oily substance and char-liked material were observed coating on the recovered catalyst surface. The corresponding EDS results showed the carbon increased remarkably and the Ni loading amount nearly did not change. This was verified by the ICP-AES analysis result, which showed no obvious Ni leaching in the solvent. The strong interaction between Ni species and SiO2, which was confirmed by the H2-TPR profiles (Fig. 2), was considered to be responsible for it. Moreover, the TEM, STEM and EDS images of the catalysts before and after reaction (Fig. S7†) were measured, where no significant change on the Ni particle size and morphology was exhibited after treatment as well. The FT-IR spectra analysis of the fresh and recovered SS-Ni/SiO2 catalyst were also compared. The characteristic infrared absorption peaks of repolymerized products could be found in the recovered catalyst. For example, the stretching of –OCH3 and –CH2 bonds (2952 and 2845 cm−1), and the vibration of aromatic ring (1606, 1514, 1462 and 843 cm−1)53,54 could be observed clearly (Fig. S8†). Therefore, it was considered that the formation of char and tar, which were caused by the repolymerization of feedstocks,54,55 was the main reason for the slight activity decrease. The FT-IR spectra also exhibited that the peaks at 3400, 1630 and 675 cm−1 were enhanced, indicating the H2O formation. And the interaction between the catalyst and the formed water was also responsible for the activity declination. To prove this conclusion, we calcined the recovered catalyst after 4 runs at 500 °C under air atmosphere and then reduced at 550 °C for 6 h at the flow of hydrogen. Remarkably, the conversion had gone up back to 100% at the next run. It indicated that the catalysts could be refreshed through simple methods, which was a prominent advantage of the catalyst for the further industrial application. Overall, the SS-Ni/SiO2 catalyst verified its excellent recyclability clearly, further suggesting the good stability of catalyst structure.
4. Conclusion
An efficient and promising strategy for lignin-derived phenolic compounds conversion to the platform chemical compounds and the low-cost high-grade biofuel was proposed via the high selective catalytic hydrogenation process. The results showed that guaiacol could be completely converted to cyclohexanol with 99.9% selectivity over the step by step precipitated Ni/SiO2 catalyst at a mild condition of 120 °C, 2 MPa H2 atmosphere for 2 h. Detailed investigation on the catalyst indicated that the polyporous spherical structure were mainly responsible for the high catalytic activity, which led to the large specific surface area and high nickel dispersion. Also, the appropriate acidity of the catalyst, as well as the strong guaiacol adsorption ability, promoted the catalytic performance significantly. Furthermore, this step by step precipitated catalyst was efficient for other typical lignin-derived phenolic compounds at this mild condition. Moreover, the catalyst showed an excellent recyclability, where no significant loss of the catalytic activity was exhibited after 3 runs. And thus, this novel process is a beneficial reference for future utilization of the lignin-derived phenolic compounds and the renewable lignin.
Acknowledgements
The authors gratefully acknowledge the financial support of the National Key Technology R&D Program, (No. 2014BAD02B01) and the Natural Science Foundation of China (No. 51476178).
References
- H. X. Ben and A. J. Ragauskas, RSC Adv., 2012, 2, 12892–12898 RSC.
- K. Barta, T. D. Matson, M. L. Fettig, S. L. Scott, A. V. Iretskii and P. C. Ford, Green Chem., 2010, 12, 1640–1647 RSC.
- Q. Song, F. Wang, J. J. Zhang, W. Q. Yu and J. Xu, Energy Environ. Sci., 2013, 6, 994–1007 Search PubMed.
- Q. W. Tian, N. X. Li, J. H. Liu, M. Wang, J. Q. Deng, J. C. Zhou and Q. H. Ma, Energy Fuels, 2015, 29, 255–261 CrossRef.
- A. K. Deepa and P. L. Dhepe, RSC Adv., 2014, 4, 12625–12629 RSC.
- A. Kloekhorst and H. J. Heeres, ACS Sustainable Chem. Eng., 2015, 3, 1905–1914 CrossRef.
- Y. Yang, A. Gilbert and C. B. Xu, Appl. Catal., A, 2009, 360, 242–249 CrossRef CAS.
- C. F. Palma, Appl. Energy, 2013, 111, 129–141 CrossRef.
- J. X. Long, W. Y. Lou, L. F. Wang, B. L. Yin and X. H. Li, Chem. Eng. Sci., 2015, 122, 24–33 CrossRef CAS.
- Y. Nakagawa, M. Ishikawa, M. Tamura and K. Tomishige, Green Chem., 2014, 16, 2197–2203 RSC.
- N. Escalona, W. Aranzaez, K. Leiva, N. Martinez and G. Pecchi, Appl. Catal., A, 2014, 481, 1–10 CrossRef CAS.
- B. Guvenatam, O. Kursun, E. H. J. Heeres, E. A. Pidko and E. J. M. Hensen, Catal. Today, 2014, 233, 83–91 CrossRef.
- Y. X. Yu, Y. Xu, T. J. Wang, L. L. Ma, Q. Zhang, X. H. Zhang and X. Zhang, J. Fuel Chem. Technol., 2013, 41, 443–447 CrossRef CAS.
- J. X. Long, R. Y. Shu, Q. Y. Wu, Z. Q. Yuan, T. J. Wang, Y. Xu, X. H. Zhang, Q. Zhang and L. L. Ma, Energy Convers. Manage., 2015, 105, 570–577 CrossRef CAS.
- N. Yan, Y. Yuan, R. Dykeman, Y. Kou and P. J. Dyson, Angew. Chem., Int. Ed., 2010, 49, 5549–5553 CrossRef CAS PubMed.
- D. D. Laskar, M. P. Tucker, X. W. Chen, G. Helms and B. Yang, Green Chem., 2014, 16, 897–910 RSC.
- W. Y. Wang, Z. Q. Qiao, K. Zhang, P. L. Liu, Y. Q. Yang and K. Wu, RSC Adv., 2014, 4, 37288–37295 RSC.
- J. B. Bredenberg, M. Huuska and P. Toropainen, J. Catal., 1989, 120, 401–408 CrossRef CAS.
- E. Laurent and B. Delmon, Appl. Catal., A, 1994, 109, 77–96 CrossRef CAS.
- E. Laurent and B. Delmon, Appl. Catal., A, 1994, 109, 97–115 CrossRef CAS.
- Y. C. Lin, C. L. Li, H. P. Wan, H. T. Lee and C. F. Liu, Energy Fuels, 2011, 25, 890–896 CrossRef CAS.
- C. Zhao, H. Z. Wang, N. Yan, C. X. Xiao, X. D. Mu, P. J. Dyson and Y. Kou, J. Catal., 2007, 250, 33–40 CrossRef CAS.
- C. Zhao, Y. Kou, A. A. Lemonidou, X. B. Li and J. A. Lercher, Angew. Chem., Int. Ed., 2009, 48, 3987–3990 CrossRef CAS PubMed.
- C. Zhao, J. Y. He, A. A. Lemonidou, X. B. Li and J. A. Lercher, J. Catal., 2011, 280, 8–16 CrossRef CAS.
- W. J. Song, Y. S. Liu, E. Baráth, C. Zhao and J. A. Lercher, Green Chem., 2015, 17, 1204–1208 RSC.
- C. Zhao, S. Kasakov, J. Y. He and J. A. Lercher, J. Catal., 2012, 296, 12–23 CrossRef.
- C. F. Wu and P. T. Williams, Appl. Catal., B, 2011, 102, 251–259 CrossRef CAS.
- C. F. Wu, Z. C. Wang, J. Huang and P. T. Williams, Fuel, 2013, 106, 697–706 CrossRef.
- A. D. Consuegra, P. M. Patterson and M. A. Keane, Appl. Catal., B, 2006, 65, 227–239 CrossRef CAS.
- P. H. Blanco, C. F. Wu, J. A. Onwudili and P. T. Williams, Appl. Catal., B, 2013, 134, 238–250 CrossRef.
- A. Saadi, R. Merabiti, Z. Rassoul and M. M. Bettahar, J. Mol. Catal. A: Chem., 2006, 253, 79–85 CrossRef CAS.
- Y. B. Hu, K. Tao, C. Z. Wu, C. Zhou, H. F. Yin and S. H. Zhou, J. Phys. Chem. C, 2013, 117, 8974–8982 CAS.
- F. Ding, Y. J. Zhang, G. J. Yuan, K. J. Wang, I. Dragutan, V. Dragutan, Y. F. Cui and J. Wu, J. Nanomater., 2015, 2015, 1–6 Search PubMed.
- S. R. Kirumakki, B. G. Shpeizer, G. V. Sagar, K. V. R. Chary and A. Clearfield, J. Catal., 2006, 242, 319–331 CrossRef.
- L. Li, S. C. He, Y. Y. Song, J. Zhao, W. J. Ji and C. T. Au, J. Catal., 2012, 288, 54–64 CrossRef CAS.
- J. S. Zhang and F. X. Li, Appl. Catal., B, 2015, 176–177, 513–521 CrossRef CAS.
- D. P. Upare, S. Yoon and C. W. Lee, J. Porous Mater., 2013, 20, 1129–1136 CrossRef CAS.
- M. V. Bykova, D. Y. Ermakov, V. V. Kaichev, O. A. Bulavchenko, A. A. Saraev, M. Y. Lebedev and V. A. Yakovlev, Appl. Catal., B, 2012, 113–114, 296–307 CrossRef CAS.
- S. Velu and S. Gangwal, Solid State Ionics, 2006, 177, 803–811 CrossRef CAS.
- Y. J. Bang, S. J. Han, J. Yoo, J. H. Choi, J. K. Lee, J. H. Song, J. Lee and I. K. Song, Appl. Catal., B, 2014, 148–149, 269–280 CrossRef CAS.
- F. Arena, R. Dario and A. Parmaliana, Appl. Catal., A, 1998, 170, 127–137 CrossRef CAS.
- K. Hadjiivanov, M. Mihaylov, D. Klissurski, P. Stefanov, N. Abadjieva, E. Vassileva and L. Mintchev, J. Catal., 1999, 185, 314–323 CrossRef CAS.
- J. H. Huang and J. X. Chen, Chin. J. Catal., 2012, 33, 790–796 CrossRef CAS.
- D. W. Fuerstenau and K. Osseoasare, J. Colloid Interface Sci., 1987, 118, 524–542 CrossRef CAS.
- R. F. S. Lenza and W. L. Vasconcelos, J. Non-Cryst. Solids, 2003, 330, 216–225 CrossRef CAS.
- M. Stefanescu, S. Sorescu, D. Susan-Resiga, O. Stefanescu and G. Vlase, J. Therm. Anal. Calorim., 2015, 121, 135–144 CrossRef CAS.
- A. Popov, E. Kondratieva, J. M. Goupil, L. Mariey, P. Bazin, J. P. Gilson, A. Travert and F. Mauge, J. Phys. Chem. C, 2010, 114, 15661–15670 CAS.
- D. D. S. Perez, R. Ruggiero, L. Morais, A. E. H. Machado and K. Mazeau, Langmuir, 2004, 20, 3151–3158 CrossRef.
- D. Frenkel and B. Smit, Understanding molecular simulation from algorithms to applications, Academic Press, San Diego, 2002 Search PubMed.
- R. Imbihl and G. Ertl, Chem. Rev., 1995, 95, 697–733 CrossRef CAS.
- J. X. Long, R. Y. Shu, Z. Q. Yuan, T. J. Wang, Y. Xu, X. H. Zhang, Q. Zhang and L. L. Ma, Appl. Energy, 2015, 157, 540–545 CrossRef CAS.
- W. Zhang, J. Z. Chen, R. L. Liu, S. P. Wang, L. M. Chen and K. G. Li, ACS Sustainable Chem. Eng., 2014, 2, 683–691 CrossRef CAS.
- J. X. Long, Y. Xu, T. J. Wang, Z. Q. Yuan, R. Y. Shu, Q. Zhang and L. L. Ma, Appl. Energy, 2015, 141, 70–79 CrossRef CAS.
- R. Y. Shu, J. X. Long, Z. Q. Yuan, Q. Zhang, T. J. Wang, C. G. Wang and L. L. Ma, Bioresour. Technol., 2015, 179, 84–90 CrossRef CAS PubMed.
- R. Y. Shu, J. X. Long, Y. Xu, L. L. Ma, Q. Zhang, T. J. Wang, C. G. Wang, Z. Q. Yuan and Q. Y. Wu, Bioresour. Technol., 2016, 200, 14–22 CrossRef CAS PubMed.
Footnote |
† Electronic supplementary information (ESI) available. See DOI: 10.1039/c5ra23041a |
|
This journal is © The Royal Society of Chemistry 2016 |