DOI:
10.1039/C5RA22176E
(Paper)
RSC Adv., 2016,
6, 3186-3197
In situ growth of Ag/Ag2O nanoparticles on g-C3N4 by a natural carbon nanodot-assisted green method for synergistic photocatalytic activity†
Received
22nd October 2015
, Accepted 22nd December 2015
First published on 23rd December 2015
Abstract
Novel visible-light-driven Ag/Ag2O@g-C3N4 (AAC) hybrid materials were synthesized successfully via a green, facile hydrothermal treatment approach by reducing AgNO3 using carbon nanodots as reducing agent for the first time. Natural leaves were used as precursors to prepare carbon nanodots. By adjusting the feed mass ratio of AgNO3 to carbon nanodots, the average diameter of Ag/Ag2O can be modulated from 8 to 33 nm. The effects of Ag/Ag2O deposition on the optical and photocatalytic performance of AAC in the degradation of rhodamine B (RhB) under visible light irradiation were systematically investigated. It was found that the Ag/Ag2O content greatly influences the photocatalytic activity of AAC nanocomposites. A suitable amount of Ag/Ag2O in the AAC system could obtain the best photocatalytic activity. The experimental results indicated that all the AAC nanocomposites showed higher photocatalytic activities compared with that of pure g-C3N4. The remarkable visible-light photocatalytic activity of AAC heterostructures could be attributed to their absorption in the visible region and low recombination rate of the electron–hole pairs because of the heterojunction formed between Ag2O and g-C3N4. In addition, Ag nanoparticles are believed to play an essential role in affecting the photoreactivity because they are able to trap electrons, further separate the electron–hole pairs, and prolong the life of electron–hole pairs. In other words, it can be concluded that the increased photocatalytic activity of AAC was attributed to the synergic effect between g-C3N4, Ag2O and Ag. It is believed that the present work could render useful information for steering the design and application of noble nanoparticle loaded g-C3N4-based heterojunction composites with high photocatalytic activity.
1. Introduction
The growing concerns about environmental and energy crises have attracted increasing attention for solar energy utilization. Heterogeneous photocatalysis has been recognized as a potential strategy for solar energy conversion and environmental remediation. Anatase TiO2 is considered as one of the best photocatalysts, but the band gap energy of anatase TiO2 (3.0–3.2 eV) requires UV light to be excited, and thus only a small portion of solar light is absorbed in the UV region.1–4 Therefore, it becomes a challenge to find novel photocatalytic catalysts with visible-light response.
Graphitic carbon nitride (g-C3N4) polymer, a polymeric metal-free semiconductor with a band gap of about 2.70 eV, is a potentially viable candidate of the photocatalytic field, due to their peculiar thermal stability, low cost preparation and visible-light responsive property.5 Nevertheless, the fast recombination of photogenerated electron–hole pairs may still limit the enhancement of its photocatalytic activity.6 Transition metal modification and heterostructured composite fabrication are the two methods introduced for lowering the recombination rate.6–8
Metal–semiconductor composite prepared by modifying metals, such as silver, gold and platinum onto a semiconductor can be significantly improve the photocatalytic activity.9–13 Noble nanoparticles (NPs) can reduce the recombination rate of the photogenerated electron–hole pairs by transferring the electrons to themselves.14 Combined Ag and g-C3N4 can enhance the photocatalytic performance due to the substantial reduction in the rate of recombination of photogenerated charge carriers by the enhanced electron transport through Ag NPs and g-C3N4.15 Zhu16 and co-workers reported the enhanced photocatalytic activity for the photodegradation of methylene blue and hydrogen evolution reaction under visible light compared to the pure g-C3N4 sample. Chen et al.17 reported that modifying g-C3N4 with Ag significantly increases the photoelectric conversion performance. Ge et al.18 prepared Ag-modified g-C3N4 composite and found that the modification of Ag on the g-C3N4 surface significantly enhanced the visible-light photocatalytic capacity of g-C3N4.
In addition, coupling g-C3N4 with the other semiconductors to form heterostructures also provides a feasible route to inhibit the recombination of photogenerated electron–hole pairs, such as TiO2@g-C3N4,19,20 ZnO@g-C3N4,21–23 TaON@g-C3N4,24 SrTiO3@g-C3N4 (ref. 25) and Fe3O4@g-C3N4.26 Ag2O is a fascinating p-type semiconductor with a narrow energy bandgap of ∼1.3 eV, which enables it to absorb visible light.27 Under visible light, pure Ag2O can absorb photons to generate electron–hole pairs. However, the fast recombination rate of charge carriers and its unstability limited its application. It has been found that Ag2O can maintain its stability and enhance the photocatalytic activity when Ag2O is coupled with other semiconductors.28–35 There is one probable reason that the heterojunction structure is formed between Ag2O and the other semiconductors (support materials) and thus the photogenerated electron–hole pairs can separate efficiently, leading to the enhanced photocatalytic performance under visible light irradiation. By comparing the energy levels of g-C3N4 with Ag2O, it is fortunate to find that their well-matched overlapping band-structures are quite suitable to construct heterostructures that would bring an effective separation and transfer of photogenerated charges. Furthermore, Ag2O NPs can be well dispersed on the surface of g-C3N4, which can provide more possible reaction sites for the catalytic reaction.
In this study, we have successfully developed a green, low-cost synthetic method to prepare Ag/Ag2O NPs loaded g-C3N4 catalyst. In the preparation of the catalyst, Ag/Ag2O was first synthesized using hydrothermal treatment approach by reducing AgNO3 with carbon nanodots. A series of AAC-X heterostructures were prepared by mixing the as-prepared g-C3N4 with different amount of the precursor of Ag/Ag2O. The synthesis route ensures not only the successful growth of Ag/Ag2O NPs on g-C3N4 nanosheets but also the high dispersion of Ag/Ag2O NPs on g-C3N4 without aggregation, and the size of Ag/Ag2O can be controlled by adjusting the adding amount of AgNO3. Photocatalytic activity of these Ag/Ag2O-deposited g-C3N4 nanosheets was tested by photocatalytic degradation of RhB as a reference model representing pollutant. The formation of well-defined junctions among Ag, Ag2O and g-C3N4 effectively facilitates charge transfer among Ag, Ag2O and g-C3N4 and suppresses the recombination of photogenerated electrons and holes, resulting in extremely high activity and stability. In addition, possible photocatalytic mechanism of AAC-X nanosheets was investigated. The results present here could highlight the importance of designing ternary composite nanostructures using this facile, green, low-cost method for highly efficient photocatalysts.
2. Experimental
2.1. Materials
Absolute ethanol (C2H5OH), sodium borohydride (NaBH4), silver nitrate (AgNO3) and RhB are of analytical grade and obtained from Sinopharm Chemical Reagent Co., LTD. Terephthalic acid (TA) and polyvinylpyrrolidone (PVP) were bought from Aladdin Reagent Co., LTD. The leaves were obtained from Jiangsu University, China and washed by water for further use. All chemicals used in the experiments were used without further purification. Deionized (DI) water was used for all experiments.
2.2. Catalyst preparation
2.2.1. Preparation of carbon nanodots. In a typical process,3 leaves (2 g) were added into deionized water (10 mL). Next, the above mixture was transferred into a 25 mL Teflon-lined autoclave and heated at 180 °C for 3 h. The as-prepared samples were collected by removing impurity through filtration.
2.2.2. Preparation of g-C3N4 powder. Typically, the g-C3N4 was synthesized by thermal treatment of 40 g of urea in a crucible with a cover under ambient pressure in air. The precursor was heated to 600 °C in 1 h in a tube furnace and maintained at this temperature for 4 h. The resulted final light yellow powder was washed in sequence with water and anhydrous ethanol thoroughly and then collected by filtration and dried at room temperature.
2.2.3. Preparation of Ag/Ag2O@g-C3N4 ternary hybrid materials. Typically, g-C3N4 (0.1 g) nanosheet was dispersed in absolute ethyl alcohol (20 mL) by ultrasonication for 20 min. After that, AgNO3 (0.02 M) and PVP (1 g) were added to the above solution under vigorous stirring. The mixture was stirred for 30 min and impregnated for 12 h. At last, certain amount of carbon nanodots (1.5, 3, 6 and 9 mL) were added to the above mixture and stirred for 5 min. Then, the above mixture was transferred into a Teflon-lined stainless steel autoclave. The autoclave was kept at 180 °C for 3 h in an electric oven. After being cooled to room temperature, the samples were collected by centrifugation, washed with deionized water and absolute ethanol several times, and then dried in a vacuum for 48 h. The schematic illustration for the formation of the Ag/Ag2O@g-C3N4 nanocomposite is shown in Scheme 1. The Ag/Ag2O@g-C3N4 ternary hybrid materials were marked as AAC-X, X label as the added volume of AgNO3 (X = 2.5, 5.0, 10.0 and 15.0 mL). Moreover, the molar ratio between Ag and Ag2O is kept consistent (cal. 3.5
:
1).
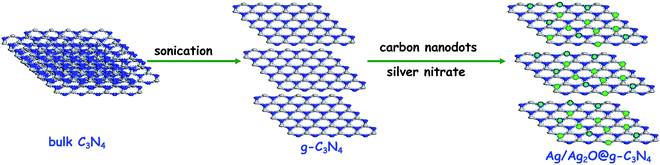 |
| Scheme 1 The schematic illustration for the formation of the AAC-X nanocomposites. | |
2.3. Characterization
The crystal structure and phase purity of the prepared samples were analyzed by X-ray diffraction (XRD) using D8 Advance X-ray diffraction (Bruker axs company, Germany) equipped with Cu-Kα radiation (λ = 1.5406 Å), employing a scanning rate of 0.02° s−1 in the 2θ range from 20 to 80°. Scanning electron microscopy (SEM) and energy-dispersive X-ray spectroscopy (EDS) were performed on a scanning electron microscope (Hitachi S-4800 II, Japan) operated at an acceleration voltage of 10 kV to characterize the morphologies and the compositions of the prepared samples. Furthermore, the morphology and particle size of the products were also examined by transmission electron microscopy (TEM) which was recorded on a JEOL-JEM-2010 (JEOL, Japan) operating at 200 kV. UV-vis diffuse reflection spectroscopy (DRS) was performed on a Shimadzu UV2550 spectrophotometer using BaSO4 as the reference. The N2 physical absorption measurements were carried out at 77 K with a NOVA2000e analytical system made by Quantachrome Corporation (USA). The specific surface area was calculated by Brunauer–Emmett–Teller (BET) method. Pore size distribution and pore volume were calculated by Barrett–Joyner–Halenda (BJH) method. X-ray photo-electron spectroscopy (XPS) analysis was measured on an ESCALAB MK X-ray photoelectron spectrometer. The PL spectra of the samples were obtained with a QuantaMaster & TimeMaster Spectrofluorometer.
2.4. Photocatalytic activity for degradation of pollutant
The photocatalytic activities were evaluated by the decomposition of RhB under visible light irradiation. Typically, 80 mg of photocatalyst was dispersed in 80 mL of 10 mg L−1 RhB aqueous solution in a reactor of double layer condensated by running water to keep the temperature (25 °C) unchanged. The pH value of the RhB solution was determined 6.2. Prior to irradiation, the suspension was magnetically stirred in dark for 30 min to ensure the establishment of an adsorption–desorption equilibrium between the photocatalyst and RhB. Then the suspension was irradiated under continuous stirring by using a 350 W xenon arc lamp with a UV-cutoff filter (420 nm) and was positioned 20 cm away from the reactor. Liquid samples were withdrawn at certain time intervals. The dye solution was separated from the catalyst using a centrifugal and the residual concentration of RhB was determined using a UV-vis spectrophotometer. For comparison, blank experiments without catalyst were also carried out. Additionally, the recycle experiments were performed for three consecutive cycles to test the durability.
2.5. Active species trapping experiments
For detecting the active species during the reaction, tert-butanol (t-BuOH), ammonium oxalate (AO) and 1,4-benzoquinone (BQ) were used as the hydroxyl radical (OH˙) scavenger, hole (h+) scavenger and superoxide radical (O2˙−) scavenger, respectively. The process was parallel to the former photocatalytic activity experiment with the addition of quencher in the presence of RhB.
3. Results and discussion
3.1. TEM analysis
The detailed characterization of the morphologies and crystal structures of as-prepared samples were based on TEM. From the TEM images in Fig. 1, the smooth and flat layers in these series of g-C3N4 based samples could be clearly seen, which was consistent with the reported results.25,36 After the introduction of Ag/Ag2O, numerous dark Ag/Ag2O NPs appear on the lamellar g-C3N4. All the Ag/Ag2O NPs are attached on the surface of g-C3N4 strongly. Fig. 1A–F shows the TEM images of as-synthesized AAC-X prepared with different amount of AgNO3. According to the TEM images, the as-prepared Ag/Ag2O NPs are all well dispersed on the surface of g-C3N4 nanosheets. The TEM image for AAC-2.5 (Fig. 1A) sample shows that only a small amount of NPs with sizes approximately ranging from 4 to 13 nm (Fig. 2A) were deposited on the surface of g-C3N4 nanosheets. The average size of Ag/Ag2O (AAC-2.5) was about 8 nm with an even distribution about this value. A series of experiments were performed by varying the amount of AgNO3, while keeping other parameters constant. As shown in Fig. 1B–D, for AAC-5.0 (Fig. 1B) and AAC-10 (Fig. 1C), Ag/Ag2O NPs with increasing amounts were uniformly distributed on g-C3N4 nanosheets, no apparent aggregation of the Ag–Ag2O NPs was discerned which leads to the formation of interfaces between Ag–Ag2O and g-C3N4 nanosheets. The size distribution of Ag/Ag2O (AAC-5.0) ranged from 4 to 16 nm (Fig. 2B) and the average size was 11 nm. When the amount of AgNO3 increased to 10 mL, the size of Ag/Ag2O NPs only slightly increased (Fig. 2C). The resulting size of Ag/Ag2O NPs (Fig. 1E) increases to 22–48 nm (Fig. 2D) with increasing the amount of AgNO3 (15 mL). The diameter of Ag/Ag2O was about 33 nm according to the size distribution. The NPs are still uniformly loaded on surface of g-C3N4 nanosheets without aggregation, offering high level exposure of the NP surface.
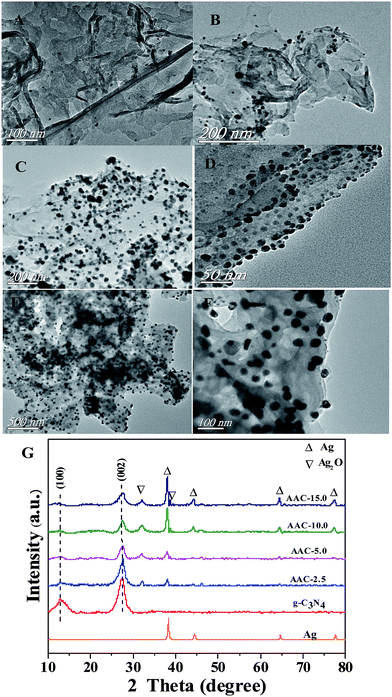 |
| Fig. 1 TEM images of AAC-2.5 (A), AAC-5.0 (B), AAC-10.0 (C and D) and AAC-15.0 (E and F); wide-angle XRD pattern (G) of as-prepared samples. | |
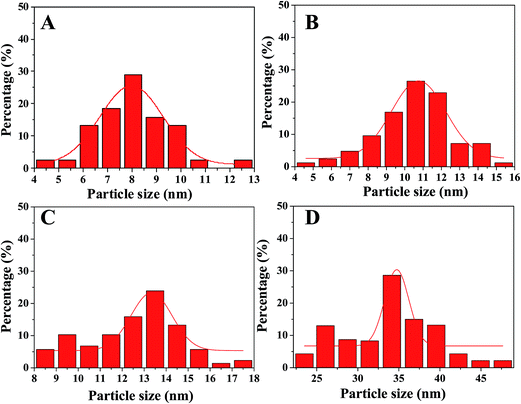 |
| Fig. 2 Particle size distribution of Ag/Ag2O NPs ((A) AAC-2.5, (B) AAC-5.0, (C) AAC-10.0 and (D) AAC-15.0). | |
3.2. XRD analysis
XRD analysis is used to investigate the phase structure of AAC-X photocatalysts. The XRD patterns of as-prepared AAC-X composites, as well as the pure g-C3N4 and Ag were shown in Fig. 1I. Two distinct diffraction peaks at 13.1° and 27.5° in the C3N4 sample, corresponding to the in-plane structural packing motif and inter-layer stacking of aromatic segments, respectively, can be indexed as the (100) and (002) peaks for graphitic materials.37,38 No significant diffraction peaks of any other phases or impurities can be detected in the composite samples, which indicates the introducing of Ag/Ag2O does not affect the crystal structure of C3N4 based photocatalysts. In addition, these two peaks become weaker gradually with increasing Ag/Ag2O contents, suggesting that Ag/Ag2O NPs have been successfully deposited onto the surface of g-C3N4. Additionally, AAC-X catalysts were found to exhibit the diffraction peaks at 38.0, 44.4, 64.4 and 77.3°, which are assigned to the (111), (200), (220) and (311) planes of face centered cubic (FCC) Ag (JCPDS card no. 65-2871).39 The peak intensity of Ag for AAC-X becomes stronger as the Ag contents increased, which may be explained by Ag plasmonic effects and high uniform dispersion in AAC-X samples.16,40 In addition, the diffraction peaks at 33.7 and 38.2° are consistent with JCPDS no. 75-1532 of Ag2O. Moreover, the weak peak of Ag2O at 26.7° may be overlapped by the strong peak of g-C3N4 at 27.5° and cannot be observed in the XRD patterns.
3.3. FT-IR analysis
The composition of AAC-X samples were further confirmed by FT-IR analysis and the results were shown in Fig. 3. The pure g-C3N4 presents three characteristic absorption regions. The broad peak at 3100–3600 cm−1 is ascribed to the stretching vibration of N–H and the stretching vibration of O–H of the physically adsorbed water.41,42 The strong bands in the 1200–1650 cm−1 regions were found in the spectrum, with the peaks at 1240, 1317, 1409, 1573 and 1636 cm−1, which corresponded to the typical stretching vibration modes of C
N and C–N heterocycles.22,43 The peak at 810 cm−1 is related to characteristic breathing mode of triazine units.44 It could also be clearly seen that the main characteristic peaks of g-C3N4 appeared in AAC-X photocatalysts, suggesting that no structural change of g-C3N4 occurs during the hybridization process. Furthermore, in the case of the AAC-X hybrid materials, the characteristic peaks of g-C3N4 did not move (red shift or blue shift) after the introduction of Ag/Ag2O NPs.
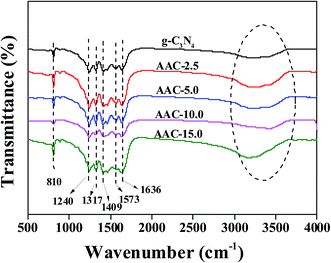 |
| Fig. 3 FT-IR spectra of g-C3N4 and AAC-X materials. | |
3.4. XPS measurements
To investigate the chemical composition and states of surface elements in the AAC-10.0 nanosheets, XPS measurements were carried out. Fig. 4A shows the XPS of ACC-10.0 in the C 1s binding energy regions and two peaks concerning at 288.1 and 284.6 are found. The peak centered at 284.6 eV can be ascribed to the C–C coordination of the surface adventitious carbon, whereas the peak at 288.1 eV corresponds to sp3-bonded C in C–N of g-C3N4.8,44 In the N 1s spectrum (Fig. 4B), several binding energies can be separated. The main signal showed occurrence of C–N–C groups (398.4 eV), tertiary nitrogen N–(C)3 groups (399.2 eV) and N–H groups (400.6 eV). The peak at 404.3 eV could be related to the charging effects.45,46 Accordingly, the asymmetric O 1s peak shown in Fig. 4C can be split by using the XPS peak-fitting program. In the atomic O 1s XPS spectrum, the peak at 532.2 eV is attributed to the external –OH group or the water molecule adsorbed on the surface and the other O 1s peak located at around 531.1 eV was assigned to oxygen atoms in the lattice of Ag2O.47 Fig. 4D presents the XPS of AAC-10.0 in the Ag 3d binding energy regions. Two individual peaks at 367.6 and 373.5 eV, corresponding to Ag 3d5/2 and Ag 3d3/2 binding energies, respectively. These two peaks can be attributed to binding energy values of Ag0.3 In addition, the peaks located at about 368.4 and 374.1 eV can be attributed to Ag+, which are very close to the binding energy values of Ag+ in pure Ag2O.47 The results indicated the existence of both Ag and Ag2O in the as-prepared composites. The binding energy values of Ag 3d and N 1s in the ternary materials are slightly higher than those of pure Ag2O and pure g-C3N4.6,27 The shift could be attributed to the surface coupling effect between Ag/Ag2O and g-C3N4. Furthermore, results from XRD and HRTEM also revealed that the obtained composites contained Ag and Ag2O. Evidently, from the all above the analysis, we can conclude that Ag/Ag2O@g-C3N4 ternary hybrid materials have successfully prepared in this case.
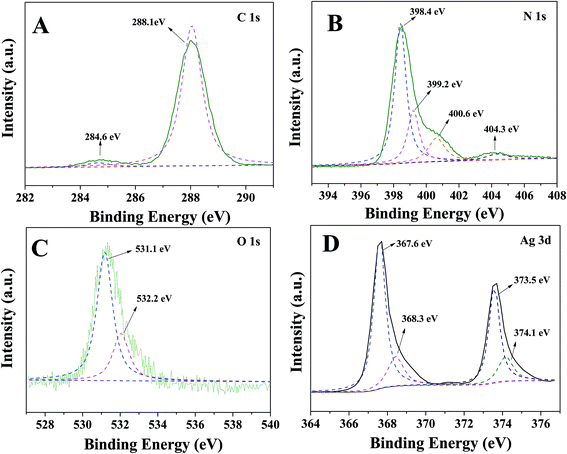 |
| Fig. 4 High-resolution XPS of AAC-10.0 nanocomposites in the (A) C 1s, (B) N 1s, (C) O 1s and (D) Ag 3d binding energy regions. | |
3.5. N2 adsorption–desorption studies
It is well-known that photocatalysts with higher specific surface areas and bigger pore volumes are beneficial for the enhancement of photocatalytic activity due to there being more surface active sites for the adsorption of reactant molecules, ease of transportation of reactant molecules and products through the interconnected porous networks, and enhanced harvesting of light.48 Fig. 5 shows the nitrogen adsorption–desorption isotherms at 77 K for pure g-C3N4 and a representative photocatalyst AAC-15.0. It can be seen that the nitrogen adsorption–desorption isotherms of these two catalysts are similar and both of them are type III with hysteresis loops according to the IUPAC classification.49–51 The BET specific surface area and pore volume of samples are summarized in Table 1. As can been seen from Table 1, the BET specific surface area (SBET) of the pure g-C3N4 is about 41.1 m2 g−1. When the Ag/Ag2O NPs were loaded, the SBET of the samples become smaller, which are slightly lower than pure g-C3N4. However, a further increase of Ag/Ag2O NPs leaded to the severely decreased SBET. It is easy to under stand that once Ag/Ag2O NPs deposit on the surface of g-C3N4 nanosheets or embedded in the pores, they will reduce the specific surface area of the samples (Fig. 5).
Table 1 The BET specific surface area and pore volume of as-prepared samples
Samples |
SBET (cm2 g−1) |
Pore volume (cm3 g−1) |
g-C3N4 |
41.1 |
0.28 |
AAC-2.5 |
39.8 |
0.25 |
AAC-5.0 |
38.3 |
0.19 |
AAC-10.0 |
37.4 |
0.18 |
AAC-15.0 |
31.1 |
0.11 |
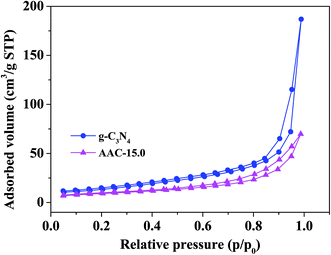 |
| Fig. 5 Typical nitrogen adsorption–desorption isotherm of g-C3N4 and AAC-15.0. | |
3.6. Optical absorption property
The light absorption properties of as-prepared g-C3N4 and typical AAC-10.0 composites are studied by UV-vis diffuse absorption spectra and the result is shown in Fig. 6. The as-prepared g-C3N4 nanosheets show a typical semiconductor absorption in the region of 200–450 nm, originating from charge transfer response of g-C3N4 from the VB populated by N 2p orbitals to the CB formed by C 2p orbitals. In addition, the sample colors shift from yellowish to dark brown with increasing Ag/Ag2O loading. This phenomenon could be attributed to a charge-transfer transition among the Ag, Ag2O species and the g-C3N4 sample.18 The band gap energy of a semiconductor can be determined by fitting the optical transition at the absorption edges using the Tauc/David–Mott model52 described by the equation: |
(αhν)1/n = A(hv − Eg)
| (1) |
where α represents the absorption coefficient, h is Planck's constant, ν is the light frequency, Eg is the band gap and A is a constant. The value of the exponent n denotes the nature of the sample transition and is defined to be 0.5/2 for a directly/indirectly allowed transition. As previous literature reported, g-C3N4 is an indirectly allowed transition. After calculated experimental data,53 Eg of pure g-C3N4 was determined from a plot of (αhν)1/2 versus energy (hν) and the band gap energy of g-C3N4 is approximate 2.69 eV, which is consistent with the existing results.54
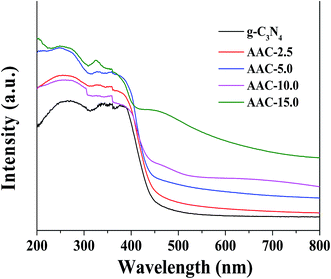 |
| Fig. 6 UV-vis diffuse reflection spectra of g-C3N4 and AAC-X. | |
3.7. Photocatalytic activity and photostability
RhB is widely used in many industrial fields such as textiles, plastics, paper, coatings, and rubber. The discharge of dyes into water has received global concern because of their overall environmental hazards. The removal of RhB from the environment become an important issue.3 To this end, the photocatalytic activities of as-prepared catalysts towards the decomposition of RhB under visible light were investigated in present study. Furthermore, commercially available Degussa P25 TiO2 and pure g-C3N4 were used as references for comparison. According to the adsorption–desorption behavior results of AAC-X without light irradiation, after 30 minutes' adsorption, there is almost no change in the concentration of dye, which indicates that the adsorption–desorption behavior reaches an equilibrium. The photodegradation of RhB using AAC-10.0 was demonstrated by the help of plots between absorption and wavelength as shown in Fig. 7A. After 140 min of visible light exposure, the concentration of RhB was found to be less than 4.5%. Fig. 7B shows the photocatalytic evaluation of the AAC-X and P25 under visible light illumination. As can be seen from Fig. 7B, RhB can only be slightly degraded under visible light irradiation without catalysts, indicating that RhB is a stable molecule and that the photolysis mechanism can be ignored. As a reference material, the P25 sample displayed a low photocatalytic efficiency of 39% after irradiation for 140 min. Fig. 7B also indicates that the as-prepared samples exhibit good photocatalytic activities for the RhB degradation reaction. The catalytic activities of all AAC-X catalysts are better than pure g-C3N4 and Ag2O. In addition, the presence of Ag/Ag2O effectively enhances the photocatalytic activity of g-C3N4. Moreover, photocatalytic activity of Ag2O@g-C3N4 is lower than those of AAC-X catalysts, suggesting the importance of Ag in degradation of RhB. When the optimum content of Ag/Ag2O was obtained (AAC-10.0), the best photocatalytic activity of Ag/Ag2O@g-C3N4 was obtained. With the Ag/Ag2O contents increased, the photocatalytic activity of AAC-X first increased and then decreased. These changes in the photocatalytic activity could be understood through considering the synergetic effects of light absorption and heterojunction structure of AAC-X. When the Ag/Ag2O content increased, the visible light absorption of Ag/Ag2O@g-C3N4 strengthened and generated more electron–hole pairs and more Ag–Ag2O@g-C3N4 heterojunction was formed, contributing to the separation of electron–hole pairs.4 However, when the Ag/Ag2O content was above the optimal value, the high Ag/Ag2O content may create some new e−/h+ pairs recombination centers, which could counteract its positive effect on the restraint of the recombination of e−/h+ pairs.55 Consequently, the separation efficiency of electron–hole pairs decreased and further restrained the photocatalytic activity. In addition, the photocatalytic activity of catalyst is highly dependent on surface area because high surface area can not only provide more surface active sites for the efficient diffusion and transportation of the degradable organic molecules and active species in photochemical reaction, but also effectively promote the separation efficiency of the electron–hole pairs. Comparing to other three as-prepared catalysts, AAC-15.0 shows lower BET surface area as discussed above (Table 1). This reason may also lead to the decreasing of photocatalytic activity of AAC-15.0. Overall the above analysis, we can conclude that the photocatalytic activity of as-prepared catalysts could depend on the content ratio of Ag/Ag2O@g-C3N4 and their surface area. Langmuir–Hinshelwood model has been widely applied for analysis of heterogeneous photocatalytic degradation kinetics of pollutants in the aqueous phase: |
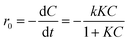 | (2) |
where r0 is the initial reaction rate (mg L−1 min−1), C is the concentration of pollutants (mg L−1), t is the reaction time (min), k is the Langmuir–Hinshelwood reaction rate constant (mg L−1 min−1) and K is the Langmuir adsorption equilibrium constant (L mg−1). At a dilute concentration of pollutants, pseudo-first-order kinetics model could be assumed as shown in eqn (3) and (4): |
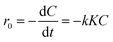 | (3) |
|
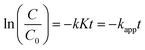 | (4) |
where kapp is the apparent rate constant (min−1), and C0 is the initial concentration of pollutants (mg L−1). The pseudo-first-order rate constants over P25, pure g-C3N4, Ag2O, Ag2O@g-C3N4, ACC-2.5, ACC-5.0, ACC-10.0 and ACC-15.0 are 0.0030, 0.0071, 0.0103, 0.0109, 0.0105, 0.0145, 0.0215 and 0.0167, respectively. These results imply that k values for all AAC-X are higher than those of P25, pure g-C3N4, Ag2O and Ag2O@g-C3N4. The photocatalytic decomposition rate of RhB over the AAC-10.0 catalyst is 3.0 and 7.0 times the activity over g-C3N4 (Ag2O) and P25, respectively. The mineralization behavior of RhB over the AAC-10 photocatalyst was examined. About 82% of total organic carbon (TOC) was decreased with irradiation time of 140 min, suggesting most of the RhB was mineralized to inorganic molecules. All these results indicate that the formation of AAC-X could greatly enhance the photocatalytic efficiency of single g-C3N4.
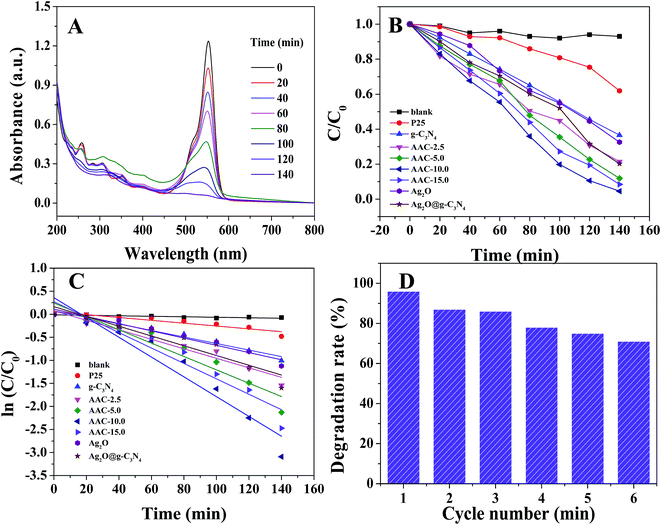 |
| Fig. 7 (A) UV-vis absorption spectra of RhB solutions in the presence of AAC-10.0 under visible light; (B) the relationship between Ct/C0 and reaction time (t) in the photodegradation of RhB; (C) the relationship between ln(Ct/C0) and reaction time (t) in the photodegradation of RhB; (D) the three run test of photocatalytic activity using AAC-10.0. | |
For practical photocatalytic applications in aqueous solution, the recycling of the AAC-X composite is an important but difficult task owing to the leakage of the individual components during the photocatalytic procedures. The photo-stability of AAC-10.0 was carried out by means of a recycling reaction. In recycling, the reacted catalysts were separated by high speed centrifuging (10
000 rpm). The separated catalysts were dried in a vacuum oven at a temperature of 80 °C for 12 h and used again. AAC-10.0 catalyst was repeatedly used for the RhB photodegradation reaction 6 times. The experimental results indicated that the catalytic activity of AAC-10.0 slightly decreased as compared to the fresh catalyst after 2 cycles, (Fig. 7D) which might because a part of Ag2O NPs were reduced to Ag either by the reduction of photogenerated electron or the visible light irradiation. This decrease trend was essentially inhibited in the third cycle, revealing that the Ag/Ag2O@g-C3N4 ternary photocatalyst has the good photostability. However, the catalytic activity further decreased in the fourth, fifth and sixth cycles because during the reusability of the photocatalyst, there would be a loss in the amount of photocatalyst as it is being centrifuged, washed and dried. To confirm the decreasing of photocatalytic activity after the third cycle was attribute to the loss in the amount of catalyst rather than the reduction of Ag2O, the composition of the recyclable composite (after third and sixth cycles) was characterized by XRD. As shown in Fig. S1-A,† after third reaction, the diffraction peaks of Ag species become stronger which could be arised from the partial in situ photoreduction of Ag2O. Previous research results demonstrated that, after the formation of a certain amount of metallic Ag, the obtained Ag2O/Ag exhibits a stable structure.55 In contrast to the three times used photocatalysts, the diffraction peaks of Ag species do not become stronger after sixth reaction. This result further proved that Ag/Ag2O@g-C3N4 ternary photocatalyst has a good photostability. Moreover, the diffraction peak ascribed to the g-C3N4 is the same for the fresh and used photocatalysts, implying the high stability of g-C3N4. No obvious changes could be observed from the high resolution XPS spectra (Fig. S1-B†) of Ag 3d for the fresh and used photocatalysts further indicated that the ternary composites are stable. Moreover, TEM analysis (Fig. S1-C and D†) was also performed to investigate the stability of as-prepared catalyst. As can be observed from TEM image, no obvious agglomeration was found (fresh and six times used catalysts), further reflecting the stability of the ternary composite. As a result, the results demonstrate that the unique, Ag/Ag2O embedded g-C3N4 nanostructures show good catalytic property in photo-stability.
3.8. PL emission spectra analysis
PL emission measurement has been widely used to investigate the fate of electron–hole pairs in semiconductor particles because PL emission is known to result from the recombination of excited electrons and holes for some semiconductors. The higher the PL emission intensity indicates the higher recombination efficiency of the photogenerated carriers and the lower the photocatalytic activity.56 Fig. 8A shows the PL emission spectra of the pure g-C3N4 and AAC-X composites at room temperature. The excitation light source for pure g-C3N4 and AAC-X is a 360 nm He–Cd laser. The main emission peak for pure g-C3N4 is centered at about 460 nm, which is approximately equal to the band gap energy of pure g-C3N4. Compared with g-C3N4, the addition of Ag/Ag2O does not alter the spectral position of the peaks, but reduces the relative intensity of PL spectral. In addition, the intensity of this emission peak depends on the Ag/Ag2O concentration. AAC-10.0 composites give the weakest PL peak, while the intensity of the PL peak increases for the highest Ag/Ag2O concentration (AAC-15.0). This result is corresponding to the photocatalysis activity. The lifetime of charge carriers in the g-C3N4 and AAC-10 were examined by using time-resolved transient PL spectroscopy, as shown in Fig. 8B. These spectra are both fitted by single-exponential decay models. The lifetime of AAC-10 (τ = 25.2 ns) is longer than the corresponding life time of g-C3N4, while maintaining similar lifetime intensities. This evidence indicates that the formation of Ag–Ag2O/g-C3N4 heterostructures prolonged the lifetime, thereby reduces the recombination of photoexcited electrons and holes. This phenomenon was also investigated by the previous reported. Hou57 and co-workers prepared g-C3N4/Bi2MoO6 heterojunctions, and it found that the lifetime is prolonged after coupling g-C3N4 with Bi2MoO6. The prolonged lifetime originates from the formation of the g-C3N4/Bi2MoO6 heterojunctions, which effectively reduces the recombination of photogenerated electrons and holes.
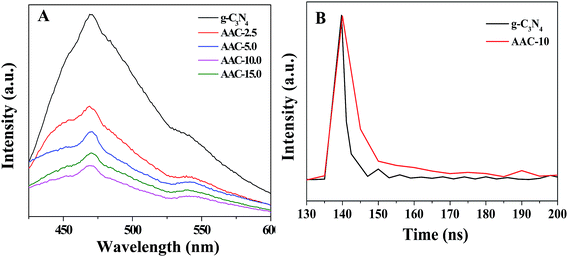 |
| Fig. 8 Room temperature PL spectra (A) of g-C3N4 and AAC-X (X = 2.5, 5.0, 10.0 and 15.0) under the excitation wavelength of 360 nm, time-resolved fluorescence decay spectra (B) of g-C3N4 and AAC-10.0. | |
3.9. Detection of reactive species
A series of experiments were conducted to probe the mechanism responsible for this visible light-induced photocatalysis. Three different quenchers, t-BuOH, AO and BQ were used as the hydroxyl radical (OH˙) scavenger, hole (h+) scavenger and superoxide radical (O2˙−) scavenger, respectively. As shown in Fig. 9, when 1 mmol of BQ as a scavenger for O2˙− radical species is added, no obvious decrease of degradation rate is observed, indicating the absence of O2˙− radical species. The experimental results (Fig. 9) also shown that the addition of 1 mmol t-BuOH or AO caused fast deactivation of the AAC-10.0 photocatalyst, reducing the photocatalytic activity for the degradation rate of RhB from 96% to 13% (t-BuOH) and 11% (AO) within 140 min. This result indicates that the OH˙ and h+ pathways have crucial roles in the process of RhB oxidation. In addition, it is well known that, holes can be adsorbed on the surface of the photocatalyst and can interact with hydroxyl radicals or water molecules to generate OH˙. The above results indicate that OH˙ is the most important oxidizing species during the RhB photocatalytic process, and that O2˙− in the solution is not the main active species. To further confirm hypothesis, we performed the photoactivity tests for OH˙ production. It is accepted that OH˙ radicals react with TA in solution and generate 2-hydroxylterephthalic acid (TAOH), which emits a unique fluorescence signal with a peak at 426 nm. Fluorescence spectra associated with TAOH were generated upon visible light irradiation of AAC-10.0, indicating that the surface structures are active in transferring photo-induced charge carriers to reactants, such as surface adsorbed water and hydroxyl groups. The nearly linear relationship between fluorescence intensity and irradiation time further confirms the stability of the as-prepared g-C3N4-based nanosheets (Fig. 10).
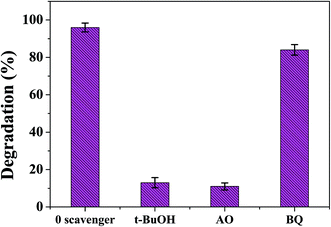 |
| Fig. 9 Trapping experiment of active species during the photocatalytic degradation of RhB over AAC-10.0 under visible light irradiation. | |
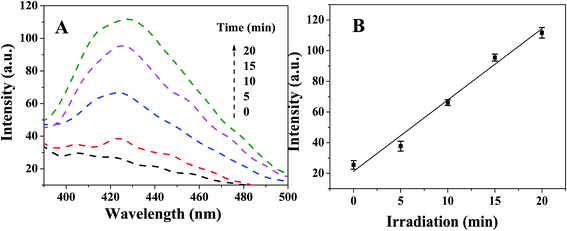 |
| Fig. 10 (A) Fluorescence spectra of AAC-10.0 nanosheets suspensions in 3 mM terephthalic acid irradiated by visible light at different irradiation times; (B) the time dependence of the fluorescence signal intensities at 426 nm on the irradiation time. | |
3.10. Possible photocatalytic mechanism of AAC-X nanosheets
In order to deeply understand the photocatalytic mechanism for the AAC-X nanosheets, the band edge positions of the valence band (VB) and conduction band (CB) of Ag2O and g-C3N4 were investigated. The VB edge potentials of Ag2O and g-C3N4 at the point of zero charge can be calculated by the following empirical equation:where EVB is the VB edge potential, X is the electronegativity of the semiconductor, which is the geometric mean of the electronegativity of the constituent atoms, Ee is the energy of free electrons on the hydrogen scale (approximately 4.5 eV), and Eg is the band gap energy of the semiconductor. The CB edge potential (ECB) can be determined by ECB = EVB − Eg. The band gap of g-C3N4 was estimated as 2.69 eV according to the literatures56 and the DRS analysis (Fig. 6). In the case of Ag2O, the optical band gap was 1.2 eV, according to the reported literature.5 Based on the band gap positions, the CB and VB edge potentials of g-C3N4 were determined at −1.12 eV and +1.57 eV, respectively.7 The CB and VB edge potentials of Ag2O were determined at +0.20 and +1.40 eV, respectively. The CB of g-C3N4 is more negative than that of Ag2O and the VB of g-C3N4 is more positive than that of Ag2O. Considering the inner electric field and energy band structure, it is accepted that transferring electrons between g-C3N4 and Ag2O would be partly restricted, while the transfer of holes can be accelerated.5 However, the electrons can transfer from g-C3N4 and Ag directly. This causes an efficient separation of photogenerated electrons and holes to enhance the photocatalytic activity. On the basis of the above analysis, the process could be described as follows (Fig. 11): under the visible light irradiation, the electrons were promoted from the VB to the CB of the g-C3N4 and Ag2O NPs, leaving the holes behind. When g-C3N4 and Ag2O are in contact, the holes generated in the VB of Ag2O NPs could migrate to the surface of g-C3N4, which promoted the effective separation of photoexcited electron–hole pairs and decreased the probability of electrons and holes recombination. The enriched holes on the VB of Ag2O could not only be consumed by directly decomposing the organic molecules on the surface of as-prepared samples, but also captured by the OH− ionized from water, which produced OH˙ free radicals with very strong oxidation capacities. The Ag2O has been proved to be an effective sacrificial reagent to capture photo-induced electrons.55 After the modification by Ag, the generated electrons on the CB of Ag2O can effectively transfer to the surface of Ag, which can hinder the charge recombination in the electron-transfer processes, thus enhance the photocatalytic performance.58 In addition, part of Ag may directly attach to the surface of g-C3N4, thus, the electrons on the CB of g-C3N4 can also transfer to the surface of Ag. Based on the above discussion, it can be accepted that the enhanced photocatalytic activity of the as-prepared AAC-X could be ascribed to the following reasons: (a) the improved optical absorption property arising from the heterojunction between g-C3N4 and Ag2O, (b) the electrons transfer from g-C3N4 to Ag, noble-metal cocatalyst (Ag) acts as a reduction active site to promote the spatial separation of photogenerated electrons and holes, and the oxygen-reduction reaction on the noble-metal cocatalyst is expected to proceed by one electron transfer route,59 (c) the synergetic effects of the inner electric field among Ag, g-C3N4 and Ag2O, (d) the low recombination rate of the photogenerated electrons which brings from the matched energy band structure.
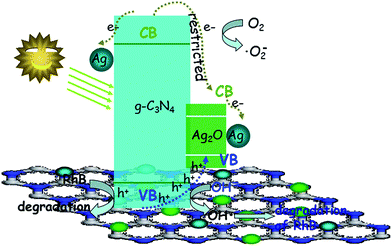 |
| Fig. 11 Schematic diagrams for the possible photocatalytic mechanism of the AAC-X composites under visible light irradiation. | |
4. Conclusion
In summary, AAC-X nanocomposites have been prepared via a green and versatile method by directly reducing AgNO3 using carbon nanodots for the first time. The average size of Ag/Ag2O can be controlled from 8 to 33 nm by adjusting the adding amount of AgNO3. The as-obtained AAC-X nanocomposites exhibited enhanced visible-light-driven photocatalytic activity and stability in the degradation of RhB in aqueous solution. The optimum photocatalytic activity of the AAC-10.0 nanocomposites for the degradation of RhB was almost 3.0 and 7.0 times higher than g-C3N4 and P25, respectively. The high activity and stability could contribute to the formation of well-defined junctions among Ag, Ag2O and g-C3N4, which effectively facilitates charge transfer and suppresses the recombination of photogenerated electrons and holes. In addition, the possible radical species involved in the degradation of RhB were analyzed by means of PL. The results indicate that the photodegradation of RhB molecules is mainly attributed to holes and hydroxyl radicals. It is expected that our present work could provide useful information for the fabrication of other metal loaded g-C3N4-based heterojunction composites.
Acknowledgements
This work was partly supported by National Natural Science Foundation (21003065, 21306067), China Scholarship Council (201508320218), Industry High Technology Foundation of Jiangsu Province (BE2013090), Innovation Project for Graduate Student Research of Jiangsu Province (KYLX_1065/1291310024) and Science & Technology Foundation of Zhenjiang (SH2012011 and GY2012048), China.
References
- G. Liu, H. G. Yang, X. Wang, L. Cheng, J. Pan, G. Q. Lu and H. M. Cheng, J. Am. Chem. Soc., 2009, 131, 12868–12869 CrossRef CAS PubMed.
- H. G. Yang, C. H. Sun, S. Z. Qiao, J. Zou, G. Liu, S. C. Smith, H. M. Cheng and G. Q. Lu, Nature, 2008, 453, 638–641 CrossRef CAS PubMed.
- Z. F. Jiang, X. M. Lv, D. L. Jiang, J. M. Xie and D. J. Mao, J. Mater. Chem. A, 2013, 1, 14963–14972 CAS.
- Z. F. Jiang, J. J. Zhu, D. Liu, W. Wei, J. M. Xie and M. Chen, CrystEngComm, 2014, 16, 2384–2394 RSC.
- X. Wang, K. Maeda, A. Thomas, K. Takanabe, G. Xin, J. M. Carlsson, K. Domen and M. Antonietti, Nat. Mater., 2009, 8, 76–80 CrossRef CAS PubMed.
- X. C. Wang, X. F. Chen, A. Thomas, X. Z. Fu and M. Antonietti, Adv. Mater., 2009, 21, 1609–1612 CrossRef CAS.
- S. Ye, L. G. Qiu, Y. P. Yuan, Y. J. Zhu, J. Xia and J. F. Zhu, J. Mater. Chem. A, 2013, 1, 3008–3015 CAS.
- Z. X Li, Y. Shen, Y. H. Guan, Y. H. Hu, Y. H. Lin and C. W. Nan, J. Mater. Chem. A, 2014, 2, 1967–1973 Search PubMed.
- H. Yan, J. Yang, G. Ma, G. Wu, X. Zong, Z. Lei, J. Shi and C. Li, J. Catal., 2009, 266, 165–168 CrossRef CAS.
- Y. Zheng, L. Zheng, Y. Zhan, X. Lin, Q. Zheng and K. Wei, Inorg. Chem., 2007, 46, 6980–6986 CrossRef CAS.
- Y. Di, X. Wang, A. Thomas and M. Antonietti, ChemCatChem, 2010, 2, 834–838 CrossRef CAS.
- M. Yoshida, A. Yamakata, K. Takanabe, J. Kubota, M. Osawa and K. Domen, J. Am. Chem. Soc., 2009, 131, 13218–13219 CrossRef CAS PubMed.
- J. Dong, M. Wang, X. Li, L. Chen, Y. He and L. Sun, ChemSusChem, 2012, 5, 2133–2138 CrossRef CAS PubMed.
- P. D. Cozzoil, R. Comparelli, E. Fanizza, M. L. Curri, A. Agostiano and D. Laub, J. Am. Chem. Soc., 2004, 126, 3868–3879 CrossRef PubMed.
- Y. L. Meng, J. Shen, D. Chen and G. Xin, Rare Met. Mater. Eng., 2011, 30, 276–279 CrossRef CAS.
- X. Bai, R. Zong, C. Li, D. Liu, Y. Liu and Y. Zhu, Appl. Catal., B, 2014, 147, 82–91 CrossRef CAS.
- Y. Bu, Z. Chen and W. Li, Appl. Catal., B, 2014, 144, 622–630 CrossRef CAS.
- L. Ge, C. Han, J. Liu and Y. Li, Appl. Catal., A, 2011, 215, 409–410 Search PubMed.
- Z. F. Jiang, D. Liu, D. L. Jiang, W. Wei, K. Qian, M. Chen and J. M. Xie, Dalton Trans., 2014, 43, 13792–13802 RSC.
- H. Yan and H. Yang, J. Alloys Compd., 2011, 509, L26–L29 CrossRef CAS.
- Y. Wang, R. Shi, J. Lin and Y. Zhu, Energy Environ. Sci., 2011, 4, 2922–2929 CAS.
- D. Chen, K. Wang, D. Xiang, R. Zong, W. Yao and Y. Zhu, Appl. Catal., B, 2014, 147, 554–561 CrossRef CAS.
- J. Sun, Y. Yuan, L. Qiu, X. Jiang, A. Xie, Y. Shen and J. Zhu, Dalton Trans., 2012, 41, 6756–6763 RSC.
- S. C. Yan, S. B. Lv, Z. S. Li and Z. G. Zou, Dalton Trans., 2010, 39, 1488–1491 RSC.
- X. Xu, G. Liu, C. Randorn and J. T. S. Irvine, Int. J. Hydrogen Energy, 2011, 36, 13501–13507 CrossRef CAS.
- J. W. Lee, H. J. Jeon, H. J. Shin and J. K. Kang, Chem. Commun., 2012, 48, 422–424 RSC.
- X. Wang, S. Li, H. Yu, J. Yu and S. Liu, Chem.–Eur. J., 2011, 17, 7777–7780 CrossRef CAS PubMed.
- Y. Li, H. Zhang, Z. Guo, J. Han, X. Zhao, Q. Zhao and S. J. Kim, Langmuir, 2008, 24, 8351–8357 CrossRef CAS PubMed.
- C. Hu, X. Hu, L. Wang, J. Qu and A. Wa, Environ. Sci. Technol., 2006, 40, 7903–7907 CrossRef CAS PubMed.
- Y. Bi, S. Ouyang, J. Cao and J. Ye, Phys. Chem. Chem. Phys., 2011, 13, 10071–10075 RSC.
- J. F. Guo, B. W. Ma, A. Y. Yin, K. N. Fan and W. L. Dai, Appl. Catal., B, 2011, 101, 580–586 CrossRef CAS.
- J. Cao, B. Luo, H. Lin and S. Chen, J. Mol. Catal. A: Chem., 2011, 344, 138–144 CrossRef CAS.
- S. Rodrigues, S. Uma, I. N. Martyanov and K. J. Klabunde, J. Catal., 2005, 233, 405–410 CrossRef CAS.
- A. Pourahmad, S. Sohrabnezhad and E. Kashefian, Spectrochim. Acta, Part A, 2010, 77, 1108–1114 CrossRef CAS PubMed.
- Y. Zang, R. Farnood and J. Currie, Chem. Eng. Sci., 2009, 64, 2881–2886 CrossRef CAS.
- S. C. Yan, Z. S. Li and Z. G. Zou, Langmuir, 2009, 25, 10397–10401 CrossRef CAS PubMed.
- S. C. Yan, Z. S. Li and Z. G. Zou, Langmuir, 2010, 26, 3894–3910 CrossRef CAS PubMed.
- J. Fu, B. Chang, Y. Tian, F. Xi and X. Dong, J. Mater. Chem. A, 2013, 1, 3083–3090 CAS.
- Y. Wen, H. Ding and Y. Shan, Nanoscale, 2011, 3, 4411–4417 RSC.
- H. Sakai, T. Kanda, H. Shibata, T. Ohkubo and M. Abe, J. Am. Chem. Soc., 2006, 128, 4944–4945 CrossRef CAS PubMed.
- J. Fu, Y. L. Tian, B. B. Chang, F. N. Xi and X. P. Dong, J. Mater. Chem., 2012, 22, 21159–21166 RSC.
- M. J. Bojdys, J. O. Muller, M. Antonietti and A. Thomas, Chem.–Eur. J., 2008, 14, 8177–8182 CrossRef CAS PubMed.
- Q. Xiang, J. Yu and M. Jaroniec, J. Phys. Chem. C, 2011, 115, 7355–7363 CAS.
- H. Xu, J. Yan, Y. G. Xu, Y. H. Song, H. M. Li, J. X. Xia, C. J. Huang and H. L. Wan, Appl. Catal., B, 2013, 129, 182–193 CrossRef CAS.
- Y. S. Xu and W. D. Zhang, ChemCatChem, 2013, 5, 2343–2351 CrossRef CAS.
- Y. Q. Sun, C. Li, Y. X. Xu, H. Bai, Z. Y. Yao and G. Q. Shi, Chem. Commun., 2010, 46, 4740–4742 RSC.
- L. Huang, H. Xu, Y. Li, H. Li, X. Cheng, J. Xia, Y. Xu and G. Cai, Dalton Trans., 2013, 42, 8606–8616 RSC.
- D. Chandra, K. Saito, T. Yui and M. Yagi, Angew. Chem., Int. Ed., 2013, 52, 12606–12609 CrossRef CAS PubMed.
- Z. F. Jiang, J. M. Xie, D. L. Jiang, X. J. Wei and M. Chen, CrystEngComm, 2013, 15, 560–569 RSC.
- Z. F. Jiang, J. M. Xie, D. L. Jiang, J. J. Jing and H. R. Qin, CrystEngComm, 2012, 14, 4601–4611 RSC.
- Z. F. Jiang, J. M. Xie, D. L. Jiang, Z. X. Yan, J. J. Jing and D. Liu, Appl. Surf. Sci., 2014, 292, 301–310 CrossRef CAS.
- X. M. Li, H. W. Zhu, J. Q. Wei, K. L. Wang, E. Y. Xu, Z. Li and D. H. Wu, Appl. Phys. A: Mater. Sci. Process., 2009, 97, 341–344 CrossRef CAS.
- Z. D. Meng, T. Ghosh, L. Zhu, J. G. Choi, C. Y. Park and W. C. Oh, J. Mater. Chem., 2012, 22, 16127–16135 RSC.
- J. S. Zhang, X. F. Chen, K. Takanabe, K. Maeda, K. Domen, J. D. Epping, X. Z. Fu, M. Antonietti and X. C. Wang, Angew. Chem., Int. Ed., 2010, 49, 441–444 CrossRef CAS PubMed.
- W. Yu, X. Liu, H. Chu, G. Zhu, J. Li, J. Liu, L. Niu, Z. Sun and L. Pan, J. Mol. Catal. A: Chem., 2015, 407, 25–31 CrossRef CAS.
- L. L. Chen, W. X. Zhang, C. Feng, Z. H. Yang and Y. M. Yang, Ind. Eng. Chem. Res., 2012, 51, 4208–4214 CrossRef CAS.
- H. Li, J. Liu, W. Hou, N. Du, R. Zhang and X. Tao, Appl. Catal., B, 2014, 160–161, 89–97 CrossRef CAS.
- X. Liu, J. Liu, H. Chu, J. Li, W. Yu, G. Zhu, L. Niu, Z. Sun, L. Pan and C. Q. Sun, Appl. Surf. Sci., 2015, 347, 269–274 CrossRef CAS.
- H. Yu, R. Liu, X. Wang, P. Wang and J. Yu, Appl. Catal., B, 2012, 111–112, 326–333 CrossRef CAS.
Footnote |
† Electronic supplementary information (ESI) available. See DOI: 10.1039/c5ra22176e |
|
This journal is © The Royal Society of Chemistry 2016 |