DOI:
10.1039/C5RA22006H
(Paper)
RSC Adv., 2016,
6, 1670-1677
Metal ion-immobilized magnetic nanoparticles for global enrichment and identification of phosphopeptides by mass spectrometry†
Received
21st October 2015
, Accepted 11th December 2015
First published on 14th December 2015
Abstract
Phosphopeptide enrichment is critical for the deep analysis of the phosphoproteome, and many strategies have been developed to improve the coverage of identified phosphopeptides. However, the isolation of multiple phosphorylated peptides is still limited due to their distinct affinity capability to various materials. For example, titanium dioxide is preferred to isolate mono-phosphopeptides owing to its very strong binding of multiple phosphorylated peptides. Here, a new set of different metal ion (Ti4+, Zr4+, Fe3+, Tb3+, Tm3+, Ho3+)-immobilized magnetic nanoparticles, Fe3O4@TCPP-DOTA-Ms, were prepared, which possess the advantages of a stable metal complex, a large binding capacity and easy manual operation by introducing TCPP (tetrakis(4-carboxylphenyl) porphyrin), DOTA (1,4,7,10-tetraazacyclododecane N,N′,N′′,N′′′-tetraacetic acid) and various metal ions onto the magnetic nanoparticles. For the model protein α-casein tryptic digest, 15 phosphopeptides were identified with Fe3O4@TCPP-DOTA-Tb or Ti, and 46.67% of the enriched phosphopeptides were multiple phosphorylated peptides, indicating that the metal ion Tb3+ and Ti4+-immobilized materials have excellent enrichment efficiency and stronger adsorption for multiple phosphorylated peptides than other metal ion immobilized magnetic nanoparticles. Even in the tryptic digest of α-casein and BSA (1
:
50), 14–15 phosphopeptides were easily detected with Fe3O4@TCPP-DOTA-Tb/Ti, revealing that the novel materials possess strong selectivity for phosphopeptide enrichment. Additionally, Fe3O4@TCPP-DOTA-Tb/Ti was utilized to capture phosphopeptides in the tryptic digest of an extract of HeLa cells. In total, 13
450 phosphopeptides corresponding to 2965 phosphoproteins were identified in mass spectrometric analyses. The specificity for phosphopeptide enrichment was as high as 94%. More than half of the identified unique phosphopeptides were multiple phosphorylated peptides, which was much higher than that identified by the DHB/TiO2 (13.39%) method, making these materials a good choice for highly selective and global phosphopeptide enrichment in phosphoproteome analysis.
1. Introduction
Reversible protein phosphorylation is a pivotal mediator of intra- and intercellular signaling events in biological systems. It regulates a broad range of complex biological processes, including cell growth, division, apoptosis, transcriptional regulation, and signal transduction.1–5 The characterization of protein phosphorylation status and quantification of dynamic changes have greatly improved the understanding of the molecular mechanisms of signal transduction and disease progression. In the past two decades, mass spectrometry (MS) has proven to be the most powerful technology to characterize and quantify phosphorylated proteins. Considering their low stoichiometric abundance, low ionization efficiency, high dynamic range and the signal suppression by non-phosphorylated peptides, it remains a major challenge to detect phosphorylated peptides, directly by MS. Thus, a dedicated enrichment method prior to MS analysis is necessary.
In recent years, to improve the coverage of the phosphoproteome, various strategies for highly efficient phosphopeptide identification have been developed. Among these strategies, affinity-based methods such as immobilized metal ion affinity chromatography (IMAC)6–8 with different metal ions and metal-oxide affinity chromatography (MOAC)9–11 have been widely utilized for selective enrichment of global phosphopeptides. IMAC is one commonly used method based on pH-dependent chelation between metal cations and the phosphate group. Different metal ions (e.g., Fe3+, Zr4+, Ti4+ and Ga3+) are immobilized onto the various carrier resins through proper linkers.12–15 The nature of metal cations is a vital factor that affects phosphopeptide enrichment efficiency. Owing to the diverse empty orbitals and the positive charges of metal ions, single metal-immobilized affinity chromatography exhibits a bias for the enrichment of phosphopeptides. For example, Ti-IMAC is apt to isolate basophilic phosphorylated peptides, whereas Fe3+-IMAC is better at enriching multiple phosphorylated peptides.16,17 Previous studies have also demonstrated that IMAC shows a strong selectivity for multiple phosphopeptides.18 Thingholm et al. developed a “SIMAC” strategy for sequential elution of monophosphorylated peptides and multiply phosphorylated peptides from IMAC resins,17 resulting in dramatically improved coverage of multiphosphorylated peptides. However, the specificity of conventional IMAC techniques is not ideal due to the unspecific adsorption of acidic peptides. MOAC has also been developed for phosphopeptide enrichment based on the Lewis acid–base interaction. Taking advantage of the high sensitivity and selectivity for phosphopeptide enrichment, TiO2 has gained increasing attention and is regarded as one of most powerful materials for phosphopeptide enrichment. However, due to the extremely strong affinity between TiO2 and multi-phosphopeptides, it has been proven that the TiO2 material shows a preference for isolating mono-phosphorylated peptides.19,20 Thus, it remains a challenge for this strategy to identify multiply phosphorylated peptides.
Magnetic nanoparticles have received great attention due to their controllable size and magnetism properties. Through being coated with various components, these magnetic materials with different surface properties have been widely applied to many areas, including cell sorting, separation, drug delivery, and enrichment in environmental and biological fields.21,22 In past two decades, many functionalized magnetic materials have been used to digest proteins, adsorb proteins, and enrich phosphopeptides or glycopeptides in proteomics research.23,24 Among these functionalized magnetic materials, magnetic IMAC materials have proven to be highly selective and sensitive for phosphopeptide enrichment due to their physical and chemical properties.7,25 Usually, metal ions Ti4+, Zr4+, Fe3+ and Ga3+ are immobilized on the surface of magnetic materials through proper chelating ligand. Thus, the chelating ligand is an important factor for phosphopeptide enrichment efficiency owing to the various chelating power and hydrophilic–hydrophobic property of different chelating ligand. Iminodiacetic acid (IDA)26 and nitrilotriacetic acid (NTA)27 are commonly used chelating ligands and recently the experimental results indicate that phosphonate group functionalized magnetic nanoparticles have better affinity ability than IDA and NTA.28 Moreover, the hydrophobic groups of magnetic IMAC materials might directly lead to unspecific peptides adsorption.29,30 Furthermore, the magnetic IMAC stability and affinity capacity for phosphate groups depend on metal complexes of the magnetic materials, which directly affect the repeatability and enrichment efficiency of phosphopeptide enrichment. Consequently, design and synthesis of novel magnetic IMAC materials with more stable complexes and high enrichment selectivity for global phosphopeptide capture are still urgently needed.
DOTA (1,4,7,10-tetraazacyclododecane N,N′,N′′,N′′′-tetraacetic acid) is a commonly used macrocyclic chelating ligand that possesses two functional groups for forming stable metal complexes through chelating a wide range of metal ions and covalently linking to another targeting vector. In recent years, due to its high thermodynamic stability and considerable kinetic inertness, DOTA is regarded as the gold standard of chelators for imaging and diagnostic applications.31,32 Liu and colleagues introduced DOTA to the field of proteomics and demonstrated that the DOTA-based metal tagging method was suitable for peptide and protein labelling for quantification research.33,34 Moreover, DOTA-functionalized magnetic IMAC materials can enhance the metal complex stability and the interaction between the materials and phosphopeptides, leading to high phosphopeptide enrichment efficiency.35
Here, a new set of magnetic materials, the Fe3O4@TCPP-DOTA-Ms (Ti4+, Zr4+, Fe3+, Tb3+, Tm3+, Ho3+), were designed and prepared for highly selective and reproducible phosphopeptide enrichment. The application of tetrakis(4-carboxylphenyl) porphyrin (TCPP) can enhance the hydrophilicity of magnetic nanoparticles and increase the amount of DOTA and metal ions immobilized on the surface of the materials, leading to excellent phosphopeptide enrichment performance. Moreover, the high stability of DOTA-based metal complex contributes to good experimental repeatability, and the unique magnetic property enables experimental processes to be rapid and convenient. Finally, through comparing phosphopeptide enrichment performance of different metal ion immobilized materials, Fe3O4@TCPP-DOTA-Ti and Fe3O4@TCPP-DOTA-Tb were selected to isolate phosphopeptides from the extract of HeLa cells. The results demonstrated that our newly developed magnetic nanoparticles displayed satisfactory selectivity and sensitivity for monophosphorylated and multiphosphorylated peptide enrichment in the real biological samples. Thus, the new phosphopeptide enrichment strategy enhances the global phosphopeptide identification, which would be a significant potential for future deep phosphoproteome studies.
2. Experimental section
2.1 Materials
Tetrakis(4-carboxylphenyl) porphyrin (TCPP) was purchased from TCI America (Portland, OR, USA). 1,4,7,10-Tetraazacyclododecane N,N′,N′′,N′′′-tetraacetic acid (DOTA) was obtained from Macrocyclics (Dallas, TX, USA). N-(3-Dimethylaminopropyl)-N-ethylcarbodiimde hydrochloride (EDC), bovine α-casein, terbium (III) chloride, thulium (III) chloride, holmium (III) chloride and 2,5-dihydroxybenzoic acid (DHB) were from Sigma-Aldrich (St. Louis, MO, USA). N-Hydroxysuccinimide (NHS) was obtained from J&K Chemical, Ltd. (Beijing, China). Sequencing-grade porcine trypsin and dithiothreitol (DTT) were purchased from Promega (Madison, WI, USA). Iodoacetamide (IAA) and trifluoroacetic acid (TFA) were obtained from Acros (Morris Plains, NJ, USA). All other chemicals, including iron(III) chloride hexahydrate (FeCl3·6H2O), iron(II) chloride tetrahydrate (FeCl2·4H2O), and ethylenediamine, were analytical grade reagents. Deionized water (with resistance > 18 MΩ cm−1) was prepared using a Millipore purification system (Billerica, MA, USA).
2.2 Synthesis of different metal ion-immobilized magnetic nanoparticles
The amine-functionalized magnetic nanoparticles (NH2-MNPs) were prepared according to the previous work in our laboratory, and the precursor was utilized for the following synthetic steps.15 In brief, TCPP and DOTA were linked on the NH2-MNPs through ethylenediamine, and then, different metal cations were immobilized by the chelation reaction of DOTA. The detailed process is as follows: 10 mg of TCPP was dissolved in ethanol and sonicated for 1 h. Then, N-(3-dimethylaminopropyl)-N-ethylcarbodiimde hydrochloride (EDC) and N-hydroxysuccinimide (NHS) were added to the solution at molar ratio of 10
:
3 for 30 min to activate part of the carboxyl groups at room temperature. Next, 4 mL of NH2-MNP was added to form Fe3O4@TCPP followed by rinsing with deionized water and ethanol with the help of a magnet. Then, EDC and NHS with a molar ratio of 2
:
1 were again added to the solution for another 30 min to activate the rest of the carboxyl groups. Next, 6 μL of ethylenediamine was added to covalently link with TCPP on the magnetic nanoparticles, followed by washing three times with deionized water and ethanol. The prepared nanoparticles were further reacted with 20 mg of DOTA in the solution and then washed with water followed by ethanol. The nanoparticles were resuspended in the ethanol solution containing 4 mM of TiCl4, ZrOCl2, FeCl3, TbCl3, TmCl3, HoCl3, respectively, at 70 °C for 6 h to chelate different metal cations. Finally, the magnetic nanoparticles were washed with ethanol and stored at 4 °C for further use.
2.3 Magnetic nanoparticle characterization
Transmission electron microscopy (TEM, FEI, Hillsboro, OR, USA) was performed on an FEI Tecnai G2 20 transmission electron microscope operated at 200 kV. The morphology of the magnetic nanoparticles was obtained by a Hitachi S-4800 cold field emission scanning electron microscope (FESEM, Hitachi, Tokyo, Japan) equipped with an energy-dispersive X-ray spectrometer (EDX) to analyze the elemental composition. Fourier transform infrared spectroscopy (FTIR, Nicolet, Denver, CO, USA) results were recorded on a Nicolet Fourier spectrophotometer using KBr pellets.
2.4 Tryptic digests of standard proteins
One milligram of bovine α-casein was dissolved in 50 mM ammonium bicarbonate buffer and denatured for 5 min at 98 °C. Then, the standard proteins were digested at 37 °C for 16 h with trypsin at a mass ratio of 1
:
50 enzyme to protein. BSA (1 mg) was first treated with DTT for 1 h to reduce the disulfide bonds of proteins. After IAA was added to the solution, the mixture was incubated in the dark at room temperature for 40 min, and the subsequent steps were performed as described above. The tryptic peptide mixtures were stored at −20 °C until subsequent use.
2.5 Tryptic digests of a protein mixture extracted from HeLa cells
HeLa cells were cultured in Dulbecco's modified eagle medium (DMEM) containing 10% fetal bovine serum (FBS), 1% penicillin/streptomycin at 37 °C in a humidified atmosphere with 5% CO2. After reaching 90% confluence, the cell pellets were collected by centrifugation and suspended in cell lysis buffer (8 M urea, 50 mM Tris–HCl (pH = 8.0), 1% DTT, 1 mM NaF, 0.2 mM Na3VO4, and 2 mM Na2H2P2O7). After brief sonication on ice, the sample was centrifuged at 14
000 × g for 30 min at 4 °C, and the supernatant was collected and stored at −80 °C for subsequent use.
For protein identification, the extract was first denatured by boiling for 5 min. Then, the sample was treated according to filter-aided sample preparation (FASP)36 to remove urea prior for further analysis.
2.6 Selective capture of phosphopeptides with Fe3O4@TCPP-DOTA-Ms
In a typical process, 150 μL of Fe3O4@TCPP-DOTA-Ms was first washed with loading buffer (50% ACN containing 1% TFA, 150 μL). The tryptic digests from α-casein, BSA were dissolved in loading buffer and then were incubated with the prepared Fe3O4@TCPP-DOTA-Ms for approximately 15 min at room temperature. For the real biological sample, one milligram of tryptic peptide mixture of an extract of HeLa cells were incubated with 2 mL of the prepared Fe3O4@TCPP-DOTA-Ti4+ and Fe3O4@TCPP-DOTA-Tb3+ for 30 min at room temperature. Subsequently, Fe3O4@TCPP-DOTA-Ms-adsorbed phosphopeptides were separated from the mixture with an external magnet. After removing the supernatant, the magnetic nanoparticles were washed with loading buffer (50% ACN containing 1% TFA) and washing buffer (50% ACN containing 0.1% TFA) three times to remove the non-specific peptides respectively. Finally, the bound phosphopeptides were eluted with 0.1 M NH3·H2O or 15% NH3·H2O depending on the sample complexity. The eluate was analyzed by MALDI-TOF-MS or Q-Exactive mass spectrometer.
2.7 Identification of phosphopeptides by mass spectrometry
The eluate was deposited on the MALDI plate, and another 1 μL of matrix solution was dropped for MALDI-TOF-MS analysis. MALDI-TOF experiments were performed in the positive reflector mode on an Applied Biosystems 4800 Proteomics Analyzer (Applied Biosystems, Framingham, MA, USA), at a repetition rate of 200 Hz and an acceleration voltage of 20 kV. All obtained mass spectra were recorded by summing 1000 laser shots and analyzed by the Data Explorer software (Version 4.5).
ESI-MS/MS was performed using a Q-Exactive mass spectrometer (Thermo Fisher Scientific, Waltham, MA, USA) equipped with a nanoflow HPLC instrument (EASY-nLC 1000 system, Thermo Fisher Scientific, Waltham, MA, USA). The collected peptide mixtures were autosampled directly and loaded onto a C18-reversed trap column (10 cm length, 100 μm internal diameter). Then the sample was separated by an analytical column (15 cm length, 75 μm internal diameter) at a flow rate of 350 nL min−1 within a 75 min linear gradient. The mobile phase A was 0.1% (v/v) formic acid in water and the mobile phase B was 0.1% (v/v) formic acid in ACN. The elution gradient was programmed from 3 to 100% mobile phase B for 90 min. An electrospray voltage of 2.0 kV was used. The scan range was 300–1400 m/z, and the 20 most intense precursor ions were selected for MS/MS analysis.
2.8 Database searching analysis
All of the processed raw files from mass spectrometry were searched against SwissProt database (20140916) using Mascot search engine (v 2.2.06). The parameter was set as follows: trypsin; up to two missed cleavage, carbamidomethyl (C) as the fixed modification and oxidation (M), and phosphorylation (S, T, Y) as the variable modification. The mass tolerance of the precursor ion and the fragment ions were 15 ppm and 20 mmu, respectively. The false discovery rates (FDRs) for peptides and proteins were set at 1%.
3. Results and discussion
3.1 Synthesis and characterization of metal ion-immobilized magnetic nanoparticles
The facile fabrication of Fe3O4@TCPP-DOTA-Ms magnetic materials containing two major steps is schematically illustrated in Fig. 1a. First, TCPP and DOTA were subsequently modified on the surface of the reacted precursor, amine-functionalized magnetic nanoparticles (NH2-MNPs). Then, different metal cations, including Ti4+, Zr4+, Fe3+, Tb3+, Tm3+, Ho3+, were chelated with DOTA to be immobilized on the surface of the respective magnetic materials. The synthesized Fe3O4@TCPP-DOTA-Ms possesses a highly stable metal complex for phosphopeptide enrichment due to its strong chelation between DOTA and metal ions. As shown in Fig. 1b, the tryptic digest of a protein and the synthesized magnetic materials were incubated for 15 min at room temperature. Then, the supernatant was removed by separating the materials in the external magnetic field. Finally, the enriched phosphopeptides were eluted by 0.1 M NH3·H2O prior to MS analysis.
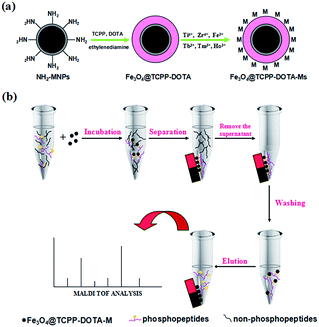 |
| Fig. 1 Schematic approach for preparation of Fe3O4@TCPP-DOTA-Ms nanoparticles (a) and the typical procedure of phosphopeptide enrichment using Fe3O4@TCPP-DOTA-Ms (b). | |
The morphology and the size of the prepared materials were measured by scanning electron microscopy (SEM) and transmission electron microscopy (TEM). As shown in Fig. 2, the representative SEM and TEM images of different metal ion-immobilized magnetic nanoparticles Fe3O4@TCPP-DOTA-Ms revealed that the obtained materials have a rough surface with a mean diameter of approximately 30 nm. The modification layer thickness was estimated to be approximately 10 nm.
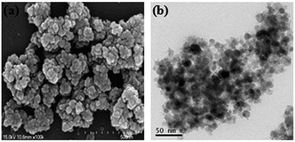 |
| Fig. 2 Representative SEM (a) and TEM (b) images of Fe3O4@TCPP-DOTA-Tb. | |
The functional modification of various groups was analyzed by Fourier transform infrared spectrometry (FTIR). Compared with the spectrum of Fe3O4 magnetic nanoparticles, some new absorption peaks were observed in the spectrum of Fe3O4@TCPP-DOTA-Ms. As shown in Fig. 3a, the new shift at 1080 cm−1 was due to the Si–O adsorption, indicating the successful coating of SiO2 on the surface of the magnetic materials.37 Another new elevated adsorption band at 1629 cm−1 corresponds to the N–H vibration, which further confirmed the linkage of TEOS, TCPP and DOTA by chemical modification.7 The results of energy-dispersive X-ray analysis (EDX) for various metal ion-immobilized materials proved that in addition to the presence of Fe, C, N, and O elements, the peaks of Ti4+, Zr4+, Fe3+, Tb3+, Tm3+, and Ho3+ were also detected, demonstrating the successful immobilization of transition elements and lanthanides on the surface of magnetic nanoparticles and the linkage of the bifunctional reagent DOTA (Fig. 3). Although the same amount of different metal cations was added during the material preparation, the EDX analysis results revealed that the amounts of metal ions immobilized on the surface of the MNPs were different, which may be attributed to the various reaction efficiencies of the metal ions and DOTA. The results above demonstrated the successful preparation of different metal ion-immobilized Fe3O4@TCPP-DOTA-Ms, which can easily be separated in an external magnetic field.
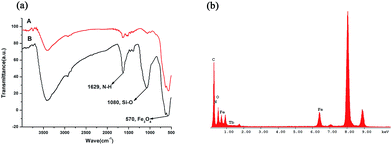 |
| Fig. 3 FT-IR spectra of Fe3O4 and Fe3O4@TCPP-DOTA-Tb (a) and energy dispersive X-ray (EDX) spectrum of Fe3O4@TCPP-DOTA-Tb (b). | |
3.2 Enrichment of phosphopeptides derived from model proteins
To evaluate the capability of different metal ion-immobilized magnetic nanoparticles Fe3O4@TCPP-DOTA-Ms for phosphopeptide enrichment efficiency, the digest of a standard phosphoprotein α-casein was used as a model. Based on the previous study of IMAC materials for phosphopeptide enrichment,38 the improved enrichment efficiency was found to be closely related to the low pH value of the loading buffer, and only the appropriate pH value guaranteed the balance between the protonation of peptides containing acidic amino acids and the deprotonation of phosphopeptides, bringing about high sensitivity and selectivity for phosphopeptide enrichment. However, the metal ions will dissociate from the magnetic materials after the pH of the loading buffer is increased.35 Thus, 1% TFA in 50% ACN was chosen as the loading buffer during the phosphopeptide enrichment process, which can efficiently isolate phosphopeptides from the digest of the model phosphoprotein. In brief, 1 μg digest of α-casein was mixed with the materials in 150 μL loading buffer for 15 min at room temperature. Then, the supernatant was removed in the external magnetic field, and the materials were subsequently washed twice with loading buffer and washing buffer. Finally, phosphopeptides from the model protein α-casein were collected with 0.1 M NH3·H2O. For comparison, α-casein digest was analyzed directly by MALDI-TOF-MS without an enrichment process (ESI Fig. S1†). The results showed that non-phosphopeptide peaks with high intensities dominated the spectrum, and the weak signal of six phosphopeptides was detected. However, after the same enrichment procedure with different metal cation-immobilized affinity materials, the intensities of the phosphopeptide peaks were markedly enhanced and nonphosphorylated peptides were almost undetectable. As shown in Fig. 4, 15 phosphopeptides from the α-casein digest were identified with Fe3O4@TCPP-DOTA-Tb and Fe3O4@TCPP-DOTA-Ti, respectively. However, 14,11,11,13-phosphopeptides were isolated by materials immobilized with metal ions Zr4+, Fe3+, Tm3+, and Ho3+. The sequences of the identified phosphopeptides are listed in Table S1–S6.† After performing the same experiments three times, the average numbers of identified phosphopeptides with Fe3O4@TCPP-DOTA-Ti and Fe3O4@TCPP-DOTA-Tb were 15 and 14, which were also higher than the number of identified phosphopeptides with Fe3O4@TCPP-DOTA-Zr, Fe, Tm, Ho, demonstrating that the phosphopeptide enrichment efficiency of metal ion Tb3+ and Ti4+ immobilized materials was better than that of other metal ion-immobilized materials. More importantly, 46.67% of the phosphopeptides identified by Fe3O4@TCPP-DOTA-Tb and Fe3O4@TCPP-DOTA-Ti were multiphosphorylated peptides, both of which were more than the multiple phosphopeptides captured by Fe3O4@TCPP-DOTA-Zr, Fe, Tm, Ho, suggesting that various metal ions affect the adsorption capacity for multiphosphorylated peptides in enrichment process. The good performance of Fe3O4@TCPP-DOTA-Ti and Fe3O4@TCPP-DOTA-Tb for global phosphopeptide enrichment might be attributed to the balance between the number of empty orbitals and the charge number of the metal cations Ti4+ and Tb3+. The metal ions with more empty orbitals are apt to interact strongly with multiple phosphate groups. However, the positive charge number of the metal ions might affect the adsorption of multiple phosphopeptides by electrostatic interaction and thus affect the specificity of the global phosphopeptide enrichment.
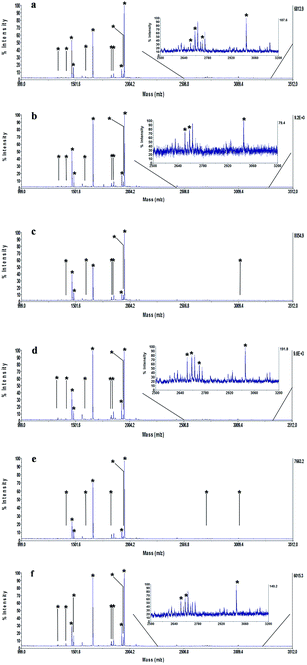 |
| Fig. 4 MALDI-TOF mass spectra of tryptic digest of 1 μg of α-casein after enrichment by Fe3O4@TCPP-DOTA-Ms (Ti4+, Zr4+, Fe3+, Tb3+, Tm3+, Ho3+) (a–f). * indicates phosphopeptides. | |
To assess the difference in the sensitivity of the developed materials Fe3O4@TCPP-DOTA-Ms for phosphopeptide enrichment, α-casein digest solution with a low concentration was employed. As shown in Fig. 5, when the total amount of α-casein was 100 fmol, 5,4,5-phosphopeptides with a clear background were detected by transition metal ion-immobilized materials Fe3O4@TCPP-DOTA-Ti, Zr, Fe. For the lanthanide element-chelated magnetic materials Fe3O4@TCPP-DOTA-Tb, Tm, Ho, 3,3,2-phosphopeptides were easily isolated from the abundant nonspecific peptides with the same concentration of α-casein. The above results showed that the sensitivity of the transition metal ion-immobilized materials for phosphopeptide enrichment was superior to the sensitivity of the lanthanide element-chelated materials, which might be attributed to the transition metal ions that were easy to coordinate with phosphate groups at a low concentration. As a whole, the high sensitivity of the new set of different metal cation-immobilized magnetic nanoparticles was due to the good hydrophilicity, the large amount of immobilized metal ions and the easy separation in magnetic field.
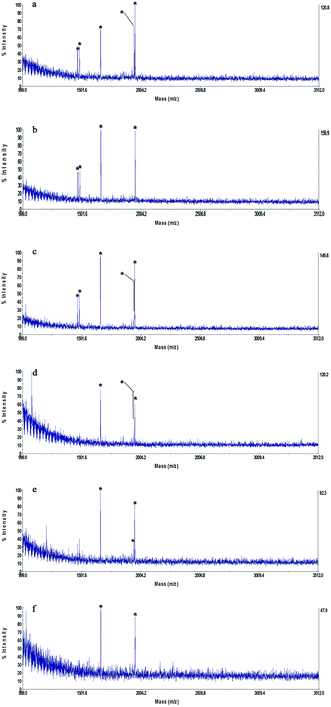 |
| Fig. 5 MALDI-TOF mass spectra of a tryptic digest of 100 fmol of α-casein after enrichment by Fe3O4@TCPP-DOTA-Ms (Ti4+, Zr4+, Fe3+, Tb3+, Tm3+, Ho3+) (a–f). * indicates phosphopeptides. | |
To examine the reproducibility of the magnetic nanoparticles for phosphopeptide enrichment, the materials were recycled after thorough washing with elution buffer. With the same operation, 1 μg digest of α-casein was incubated five times with magnetic materials. Then, 17,15,16-phosphopeptides were still detected by transition metal cation-immobilized materials, and 17,16,17-target peptides were also trapped by lanthanide element-chelated materials, which have similar mass spectra to obtained initially (ESI Fig. S2 and S3†). The results proved that the newly developed materials have good experimental reproducibility for selective phosphopeptide enrichment and a strong chelation reaction between DOTA and metal ions.
To study the specificity of the magnetic nanoparticles Fe3O4@TCPP-DOTA-Ms for phosphopeptide enrichment, a mixture of α-casein and BSA tryptic digest was utilized as the test sample. As shown in Fig. 6, when the molar ratio of α-casein and BSA was 1
:
50, there was no phosphopeptide identified before the enrichment (ESI Fig. S4†). However, after the enrichment with transition metal ion-immobilized materials, 14,12,12-phosphopeptides were observed with remarkably enhanced intensity. Compared to the above results, 15,12,7-phosphopeptide peaks were isolated using the lanthanide element-chelated materials, which dominated the main spectra with a higher S/N ratio (Table S7–S12†). Thus, the specificity of the lanthanide element-chelated materials for phosphopeptide enrichment was higher than the specificity of the transition metal ion-immobilized materials, which was consistent with the detection limit of phosphopeptide enrichment in the above results. Generally, a higher sensitivity of transition metal ion-immobilized magnetic materials might result in more nonphosphorylated peptides to be captured, leading to poorer specificity for phosphopeptide enrichment. For comparison, Ti4+, Zr4+-immobilized commercial IMAC materials were also employed to identify phosphopeptides from a relatively complex sample. With the same enrichment process, 9 and 7 phosphopeptides with lower intensity and more non-specific peptides were observed respectively (ESI Fig. S5†). Moreover, the commercial IMAC materials were collected by centrifugation during the washing and elution steps, which was time-consuming, inconvenient and might bring about the materials loss. Thus, the above results demonstrate that better performance and higher specificity of developed magnetic materials for phosphopeptide enrichment was achieved compared with commercial IMAC materials under the present experimental conditions. The lanthanide element-chelated materials were outstanding for specific enrichment of phosphopeptides. To achieve deeper coverage of phosphopeptides from a real biological sample, a strategy for combination of transition metal ions and lanthanide element-immobilized materials for phosphopeptide isolation might be a better choice.
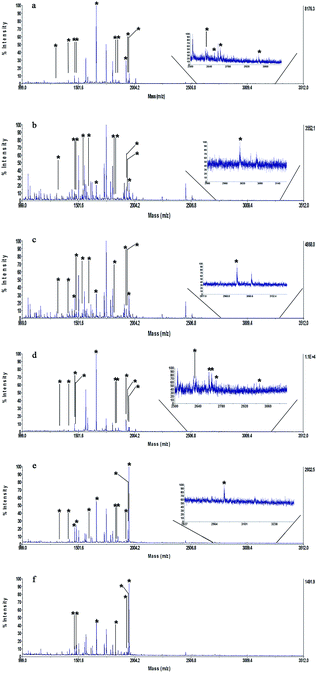 |
| Fig. 6 MALDI-TOF mass spectra of the mixture of α-casein tryptic digest and BSA tryptic digest at a molar ratio of 1 : 50, analyses after enrichment by Fe3O4@TCPP-DOTA-Ms (Ti4+, Zr4+, Fe3+, Tb3+, Tm3+, Ho3+) (a–f). * indicates phosphopeptides. | |
3.3 Application in global enrichment of phosphopeptides derived from a HeLa cell extract
To further investigate the practical application of magnetic materials for phosphopeptide enrichment in a complex biological sample, Fe3O4@TCPP-DOTA-Ti and Fe3O4@TCPP-DOTA-Tb were applied to capture phosphopeptides from the lysate digest of a HeLa cell extract. The protein extraction of HeLa cells was performed in accordance with the previous study in our laboratory.35 After one milligram of proteins of HeLa cells was digested with trypsin, the collected peptides were dried and redissolved in the loading buffer. Then, the peptides were incubated with Fe3O4@TCPP-DOTA-Ti and Fe3O4@TCPP-DOTA-Tb separately for approximately 30 min. After desalting, the dried phosphopeptides were analyzed by Q-Exactive mass spectrometry. After technically running each enriched sample twice, in total, 13
450 phosphopeptides corresponding to 2965 phosphorylated proteins were identified, of which 13
116 were unique phosphopeptides. The specificity of the newly developed Fe3O4@TCPP-DOTA-Tb and Fe3O4@TCPP-DOTA-Ti reached 94%, indicating extremely outstanding affinity performance of our new materials for phosphopeptide enrichment. Previous studies have shown that TiO2 materials had a preference to selectively enrich mono-phosphorylated peptides.19 However, 48.16% mono-, 35.97% di-, 11.36% triphosphopeptides and 4.5% tetraphosphopeptides were identified in our enrichment strategy. The number of multiphosphorylated peptides accounted for more than 50% of the captured unique phosphopeptides, which was much higher than the number identified by the classic DHB/TiO2 method (13.39%),19 suggesting the advantage of the newly developed materials for multi-phosphopeptide enrichment. The percentage of identified multiphosphorylated peptides was found to account for 57.21% from Fe3O4@TCPP-DOTA-Tb and 41.89% from Fe3O4@TCPP-DOTA-Ti, which might be due to the different number of empty orbitals of the metal ions Ti4+ and Tb3+, resulting in various affinities for multi-phosphopeptides with more negative charges. Moreover, among all 11
752 identified phosphorylated sites, 8513 were highly reliable sites. Among these highly reliable sites, 90.45% were located at serine residues, 8.81% at threonine residues and 0.75% at tyrosine residues, indicating no bias in our developed phosphopeptide enrichment procedure. The above results all demonstrated that the combination strategy of Fe3O4@TCPP-DOTA-Ti and Fe3O4@TCPP-DOTA-Tb was capable of globally capturing phosphopeptides from a complicated biological sample and has great potential in the application of this newly developed method in deep phosphoproteome analysis.
4. Conclusions
Fe3O4@TCPP-DOTA-Ms, a set of affinity materials, were successfully synthesized by simple and rapid chemical reactions for the enrichment of phosphopeptides from the digest of model protein and a real biological sample. The prepared materials have a large amount of immobilized metal cations and good hydrophilicity in solution and can form stable metal complexes. Experimental results showed that the Fe3O4@TCPP-DOTA-Ms have high sensitivity and specificity for global enrichment of phosphopeptides. Furthermore, the enrichment process of our magnetic nanoparticles was time-saving and convenient, compared to commercial IMAC materials under the same experimental conditions. This strategy provides a novel way to enrich and identify phosphopeptides in comprehensive phosphoproteome research in the future.
Acknowledgements
This work was supported by the National Key Program for Basic Research of China (Grants 2012CB910603, 2013CB911204), The National Key Program for Scientific Instrument and Equipment Development (Grants 2012YQ12004407, 2011YQ030139, 2011YQ06008408, 2013YQ14040506), The National Program for High Technology Research and Development (Grants 2012AA020200), and The National Natural Science Foundation of China (Grants 21275159 and 21235001).
Notes and references
- J. Ptacek, G. Devgan, G. Michaud, H. Zhu, X. Zhu, J. Fasolo, H. Guo, G. Jona, A. Breitkreutz, R. Sopko, R. R. McCartney, M. C. Schmidt, N. Rachidi, S. J. Lee, A. S. Mah, L. Meng, M. J. Stark, D. F. Stern, C. De Virgilio, M. Tyers, B. Andrews, M. Gerstein, B. Schweitzer, P. F. Predki and M. Snyder, Nature, 2005, 438, 679–684 CrossRef CAS PubMed
. - D. S. O'Connor, D. Grossman, J. Plescia, F. Li, H. Zhang, A. Villa, S. Tognin, P. C. Marchisio and D. C. Altieri, Proc. Natl. Acad. Sci. U. S. A., 2000, 97, 13103–13107 CrossRef PubMed
. - B. Mayr and M. Montminy, Nat. Rev. Mol. Cell Biol., 2001, 2, 599–609 CrossRef CAS PubMed
. - A. W. Truman, K. Kristjansdottir, D. Wolfgeher, N. Hasin, S. Polier, H. Zhang, S. Perrett, C. Prodromou, G. W. Jones and S. J. Kron, Cell, 2012, 151, 1308–1318 CrossRef CAS PubMed
. - J. V. Olsen, B. Blagoev, F. Gnad, B. Macek, C. Kumar, P. Mortensen and M. Mann, Cell, 2006, 127, 635–648 CrossRef CAS PubMed
. - J. Ye, X. Zhang, C. Young, X. Zhao, Q. Hao, L. Cheng and O. N. Jensen, J. Proteome Res., 2010, 9, 3561–3573 CrossRef CAS PubMed
. - Y. Yan, Z. Zheng, C. Deng, X. Zhang and P. Yang, Chem. Commun., 2013, 49, 5055–5057 RSC
. - S. Feng, M. Ye, H. Zhou, X. Jiang, X. Jiang, H. Zou and B. Gong, Mol. Cell. Proteomics, 2007, 6, 1656–1665 CAS
. - M. Wang, C. Deng, Y. Li and X. Zhang, ACS Appl. Mater. Interfaces, 2014, 6, 11775–11782 CAS
. - J. Lu, C. Deng, X. Zhang and P. Yang, ACS Appl. Mater. Interfaces, 2013, 5, 7330–7334 CAS
. - S. T. Wang, M. Y. Wang, X. Su, B. F. Yuan and Y. Q. Feng, Anal. Chem., 2012, 84, 7763–7770 CrossRef CAS PubMed
. - C. F. Tsai, C. C. Hsu, J. N. Hung, Y. T. Wang, W. K. Choong, M. Y. Zeng, P. Y. Lin, R. W. Hong, T. Y. Sung and Y. J. Chen, Anal. Chem., 2014, 86, 685–693 CrossRef CAS PubMed
. - B. Ruprecht, H. Koch, G. Medard, M. Mundt, B. Kuster and S. Lemeer, Mol. Cell. Proteomics, 2015, 14, 205–215 CAS
. - H. Zhou, M. Ye, J. Dong, E. Corradini, A. Cristobal, A. J. Heck, H. Zou and S. Mohammed, Nat. Protoc., 2013, 8, 461–480 CrossRef CAS PubMed
. - J. Wei, Y. Zhang, J. Wang, F. Tan, J. Liu, Y. Cai and X. Qian, Rapid Commun. Mass Spectrom., 2008, 22, 1069–1080 CrossRef CAS PubMed
. - H. Zhou, T. Y. Low, M. L. Hennrich, H. van der Toorn, T. Schwend, H. Zou, S. Mohammed and A. J. Heck, Mol. Cell. Proteomics, 2011, 10, M110.006452 Search PubMed
. - T. E. Thingholm, O. N. Jensen, P. J. Robinson and M. R. Larsen, Mol. Cell. Proteomics, 2008, 7, 661–671 CAS
. - S. B. Ficarro, M. L. McCleland, P. T. Stukenberg, D. J. Burke, M. M. Ross, J. Shabanowitz, D. F. Hunt and F. M. White, Nat. Biotechnol., 2002, 20, 301–305 CrossRef CAS PubMed
. - X. Zhao, Q. Wang, S. Wang, X. Zou, M. An, X. Zhang and J. Ji, J. Proteome Res., 2013, 12, 2467–2476 CrossRef CAS PubMed
. - B. Bodenmiller, L. N. Mueller, M. Mueller, B. Domon and R. Aebersold, Nat. Methods, 2007, 4, 231–237 CrossRef CAS PubMed
. - B. Liu, C. Li, P. Ma, Y. Chen, Y. Zhang, Z. Hou, S. Huang and J. Lin, Nanoscale, 2015, 7, 1839–1848 RSC
. - Y. Hao, R. Gao, L. Shi, D. Liu, Y. Tang and Z. Guo, J. Chromatogr., A, 2015, 1396, 7–16 CrossRef CAS PubMed
. - M. Zhao, C. Deng and X. Zhang, Chem. Commun., 2014, 50, 6228–6231 RSC
. - W. Qin, Z. Song, C. Fan, W. Zhang, Y. Cai, Y. Zhang and X. Qian, Anal. Chem., 2012, 84, 3138–3144 CrossRef CAS PubMed
. - C. Y. Lo, W. Y. Chen, C. T. Chen and Y. C. Chen, J. Proteome Res., 2007, 6, 887–893 CrossRef CAS PubMed
. - Y. Li, D. Qi, C. Deng, P. Yang and X. Zhang, J. Proteome Res., 2008, 7, 1767–1777 CrossRef CAS PubMed
. - Y. C. Li, Y. S. Lin, P. J. Tsai, C. T. Chen, W. Y. Chen and Y. C. Chen, Anal. Chem., 2007, 79, 7519–7525 CrossRef CAS PubMed
. - J. Lu, Y. Li and C. Deng, Nanoscale, 2011, 3, 1225–1233 RSC
. - X. S. Li, J. H. Wu, Y. Zhao, W. P. Zhang, Q. Gao, L. Guo, B. F. Yuan and Y. Q. Feng, J. Proteome Res., 2011, 1218, 3845–3853 CAS
. - Y. Li, X. Zhang and C. Deng, Chem. Soc. Rev., 2013, 42, 8517–8539 RSC
. - S. Pandya, J. Yu and D. Parker, Dalton Trans., 2006, 23, 2757–2766 RSC
. - M. Meckel, M. Fellner, N. Thieme, R. Bergmann, V. Kubicek and F. Rösch, Nucl. Med. Biol., 2013, 40, 823–830 CrossRef CAS PubMed
. - H. Liu, Y. Zhang, J. Wang, D. Wang, C. Zhou, Y. Cai and X. Qian, Anal. Chem., 2006, 78, 6614–6621 CrossRef CAS PubMed
. - X. Wang, X. Wang, W. Qin, H. Lin, J. Wang, J. Wei, Y. Zhang and X. Qian, Analyst, 2013, 138, 5309–5317 RSC
. - R. Zhai, F. Jiao, D. Feng, F. Hao, J. Li, N. Li, H. Yan, H. Wang, Z. Jin, Y. Zhang and X. Qian, Electrophoresis, 2014, 35, 3470–3478 CrossRef CAS PubMed
. - J. R. Wiśniewski, A. Zougman, N. Nagaraj and M. Mann, Nat. Methods, 2009, 6, 359–362 CrossRef PubMed
. - G. Cheng, Y. L. Liu, J. L. Zhang, D. H. Sun and J. Z. Ni, Anal. Bioanal. Chem., 2012, 404, 763–770 CrossRef CAS PubMed
. - M. R. Mirza, M. Rainer, C. B. Messner, Y. Güzel, D. Schemeth, T. Stasyk, M. I. Choudhary, L. A. Huber, B. M. Rode and G. K. Bonn, Analyst, 2013, 138, 2995–3004 RSC
.
Footnote |
† Electronic supplementary information (ESI) available. See DOI: 10.1039/c5ra22006h |
|
This journal is © The Royal Society of Chemistry 2016 |