DOI:
10.1039/C5RA21959K
(Paper)
RSC Adv., 2016,
6, 9247-9253
New solution-processable carbazole derivatives as deep blue emitters for organic light-emitting diodes†
Received
20th October 2015
, Accepted 11th January 2016
First published on 14th January 2016
Abstract
Two new compounds based on three carbazole units connected by triple bonds as π-spacers have been developed as deep blue emitters for organic light-emitting diodes (OLEDs). Their optical and electrochemical properties were examined and their charge carrier transport properties were investigated by means of the xerographic time-of-flight (XTOF) technique. The prepared diodes demonstrate the feasibility of the new molecules as effective emitters in the deep blue region yielding devices with low turn-on voltages.
Introduction
Organic light-emitting diodes (OLEDs) have been studied extensively due to their promising applications in flat-panel displays and solid-state lighting. However, further improvement of power efficiency, colour purity and longer operational lifetimes are still required to produce efficient industrial devices. The development of OLEDs that emit in the blue region (defined by a Commission Internationale de l'Eclairage chromaticity y coordinate (CIE y) value less than 0.1) have attracted intensive research, since these materials can be applied in combination with green and red colour emitters in white OLEDs and full-colour displays. Whereas green and red emitters have reached a more successful development, the fabrication of high power-efficiency and bright blue OLEDs is complex due to the intrinsic large band gaps of the organic materials, which make difficult the injection of charges into the emitters, and the lower sensitivity of the human eye in this part of the electromagnetic spectrum, which decreases the efficacy. In addition, blue OLEDs are more prone to degradation showing poor long-term stability and shorter lifetimes.1 Nevertheless, several efficient deep-blue OLEDs have been reported without the use of host–dopant systems.2
Carbazole based compounds have been reported as good candidates for hole transport materials.3 In addition, the relatively weak π-donating ability of the carbazole moiety makes of them suitable candidates for deep-blue emitters.4 Therefore, the design of new carbazole based dyes that could present both blue fluorescent properties and hole transport characteristics is an attractive challenge. Herein we present the synthesis and physical properties of two new dyes, which incorporate three carbazole units in their design (Scheme 1). Whereas the central carbazole unit has been maintained with no substitution in the nitrogen atom to favour electronic delocalization, the two external carbazole units connected to the 3 and 6 positions of the central core have been substituted by two different groups to introduce steric hindrance in order to get higher quantum yields. The carbazole triple based substitution has been selected to promote luminescence in the blue zone of the visible spectrum. Furthermore, carbazole derivatives are soluble in many solvents, overcoming the insolubility of small molecules and opening the door to the construction of OLEDs by solution processing.
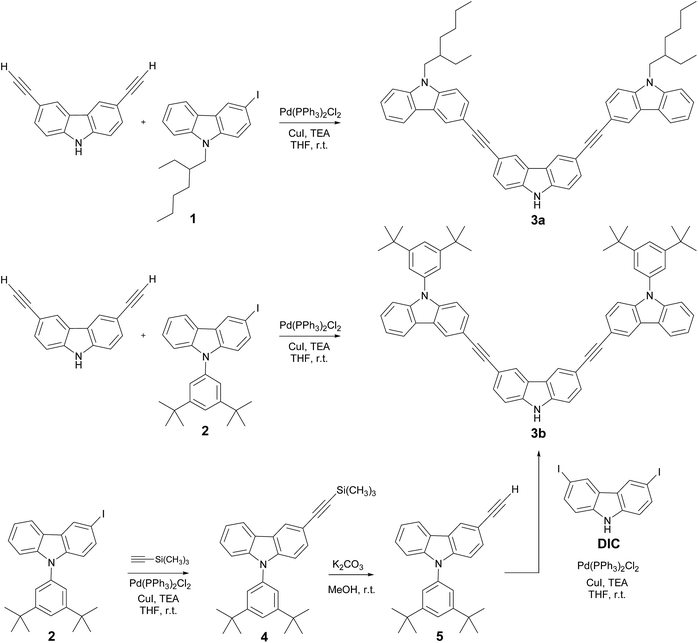 |
| Scheme 1 Synthetic routes towards 3a and 3b. | |
Results and discussion
Synthesis and characterization
Two new blue fluorescent carbazole-based dyes were designed and synthesized, as shown in Scheme 1. Sonogashira coupling reaction5 of 3,6-diethynyl-9H-carbazole6 with 1 and 2 in the presence of triethylamine (TEA) under Pd(PPh3)2Cl2 catalysis gave final derivatives 3,6-bis[2-(9-(2-ethylhexyl)-9H-carbazol-3-yl)ethynyl]-9H-carbazole (3a) and 3,6-bis[2-(9-(3,5-di-tert-butylphenyl)-9H-carbazol-3-yl)ethynyl]-9H-carbazole (3b), respectively. Previously, compounds 1 (ref. 7) and 2 were prepared from 3-iodo-9H-carbazole (MIC)8 with 2-ethylhexyl bromide in the presence of KOH and 1-bromo-3,5-di-tert-butylbenzene, respectively.
In order to get coupling product 3b in higher yield, a second synthetic route was investigated from 3,6-diiodo-9H-carbazole (DIC).8 For that purpose 9-(3,5-di-tert-butylphenyl)-3-[2-(trimethylsilyl)ethynyl]-9H-carbazole (4) and 9-(3,5-di-tert-butylphenyl)-3-ethynyl-9H-carbazole (5) were prepared from compound 2. The second route afforded a slight increase of the yield from 15 to 24%.
The chemical structure of all synthesized compounds was confirmed by spectroscopy techniques (1H NMR and 13C NMR) and mass spectra. No liquid crystal phases were observed for compounds 3a and 3b, neither by Differential Scanning Microscopy (DSC) nor by Polarized Optical Microscopy (MOP) techniques, where no liquid crystal typical textures were displayed.
Photophysical properties
Molecules 3a and 3b are soluble in common organic solvents such as chlorobenzene, dichloromethane or tetrahydrofuran. The photophysical properties of dyes 3a and 3b in solution and in solid state are summarized in Table 1. Fluorescence measurements in dichloromethane solution, after excitation at 300 nm, show maximum fluorescent peaks centred at 371 and 392, and at 369 and 390 nm, for 3a and 3b, respectively. The emission in solid state samples after photoexcitation renders a single peak in both cases located at 410 and 398 nm, respectively (Fig. 1). The bathochromic shift observed in the solid state emission, compared to the measurements in solution, is more evident on the 3a molecule due to the lower steric hindrance, in comparison to compound 3b with the 3,5-di-tert-butylphenyl group, allowing better van der Waals interactions between neighbouring molecules. The quantum yield of the N-aryl substituted 3b solid state thin-films drops from 0.31 in solution to 0.11 in the solid state, while the N-alkylated analogue 3a maintain substantially unchanged its fluorescence efficiency. Therefore, the introduction of the 2-ethylhexyl substitution on the carbazole units has demonstrated to be more efficient to avoid the quenching of the fluorescence, than the steric hindrance introduced by the 3,5-di-tert-butylphenyl group. It is worth to take into account that the three carbazole units in molecules 3a and 3b are linked by a triple bond. When the triple bond is used as a π-spacer the Stokes shift is reduced in comparison to the double bond analog9 keeping the fluorescence emission wavelength in the blue zone. It is interesting to highlight that both compounds present a CIE y coordinate in the range of the deep blue region emission (CIE y coordinate lower than 0.1).
Table 1 Optical and electrochemical properties for compounds 3a and 3b
Compd |
λabs,maxa (nm) |
λem,maxa (nm) |
Φa |
λem,maxb (nm) |
Φb |
CIEb |
Egapc (eV) |
Eoxonsetd (V) |
IPe (eV) |
EA (eV) |
Measured in CH2Cl2 at 10 μM. Quantum yield measurements (Φ) were done after excitation at 300 nm using POPOP as the standard reference (1,4-bis(5-phenyl-2-oxazolyl)benzene). Measured in thin films on quartz substrates. Optical gap energy. Onset oxidation potential determined from cyclic voltammetry. IP = Eoxonset − E0(Fc/Fc+) + 5.39. |
3a |
305, 325, 349 |
371, 392 |
0.39 |
410 |
0.31 |
(0.17, 0.07) |
3.32 |
0.93 |
5.89 |
2.57 |
3b |
305, 324, 349 |
369, 390 |
0.31 |
398 |
0.11 |
(0.18, 0.11) |
3.33 |
0.99 |
5.95 |
2.62 |
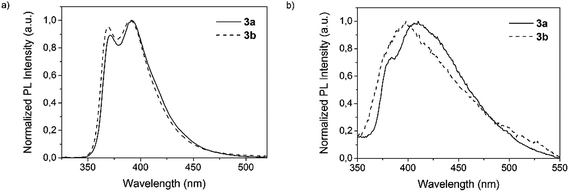 |
| Fig. 1 Photoluminescence spectra of compounds 3a and 3b (a) in dichloromethane at a concentration of 10 μM and (b) in the solid state. | |
In order to increase efficiency in OLED devices it is a good strategy to design luminescent dyes, not only with high quantum yields in the solid state at the selected wavelengths but also with high charge carrier mobilities to favour the whole electroluminescence process.
Electrochemical properties
Electrochemical properties of compounds 3a and 3b were analysed. Cyclic voltammetry (CV) for both compounds were performed in dichloromethane containing 0.1 M TBAP as the supporting electrolyte. Both compounds showed one irreversible oxidation process by cyclic voltammetry (Fig. S17 in the ESI†), being both stable to the reduction process. Table 1 collects the electrochemical characteristics. High ionization potential (IP) values (∼5.9 eV), estimated from oxidation onset potentials by cyclic voltammetry, and high optical gap energy values (∼3.3 eV), estimated in combination with UV-visible spectra, were obtained for compound 3a and 3b, which are indicative of potential hole conduction properties. The substitution of the nitrogen atoms of the carbazole moieties has a slight influence on the ionization potential values, being the ionization potential of the N-aryl derivative 3b slightly higher.
The nature of the π-spacer between two carbazole units influences the electron affinity (EA) values.9 Higher EA values were obtained for bicarbazole derivatives bonded by two consecutive triple bonds, instead of by only one double bond or one triple bond. Compounds 3a and 3b, which consist on three carbazole units with a triple bond as a π-spacer intercalated between them, present high EA values which potentially facilitates electron injection into the active layer.
Charge transport properties
Ionization potential (IP), which characterizes the electron releasing work under illumination, was also determined in the solid state for both 3a and 3b compounds by the photo-emission in air method. Photoemission spectra of the amorphous films of both compounds in air are shown in Fig. 2a. IP values for compounds 3a and 3b are 5.33 and 5.44 eV, respectively, close to that of indium tin oxide (4.8 eV). The nature of the N-substituents of the carbazole core has only a slight influence on the ionization potential values. This observation is in agreement with previous reports where it was found that the modification of the substitution patterns of the carbazole moiety practically does not affect the ionization energy values.10 It should be taken into account that from the electrochemical properties, the IP values of compounds 3a and 3b (Table 1) were determined in solution, whereas in the photoemission in air technique the IP is referred to solid thin-films. This slightly difference in IP values obtained from the two different techniques has been previously reported.11
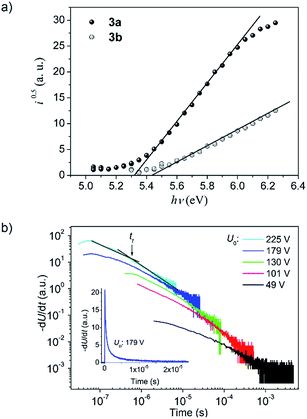 |
| Fig. 2 (a) Photoemission spectra of the amorphous films of compounds 3a and 3b measured in air at 25 °C. (b) XTOF transients for compound 3a measured at 25 °C. Insert shows one transient curve in linear plot. | |
For the estimation of hole transport properties, amorphous layers of the synthesized materials were prepared and subjected to the xerographic time-of-flight (XTOF) measurements. Representative XTOF transients for hole transport of compound 3a are displayed in Fig. 2b, showing a dispersive pattern. The attempts to estimate the hole mobility for compound 3b were unsuccessful, due to the much more dispersive mobility registered for that compound.
The hole-transit times (tt) of 3a were established from intersection points of two asymptotes from the double-logarithmic plots (Fig. 2b). The dependency of hole drift mobilities on the square root of the electric field for compound 3a is represented in Fig. S18 in the ESI.† Compound 3a showed a zero field hole drift mobility (μ0) of 2.6 × 10−7 cm2 V−1 s−1 and a field dependence parameter (α) of ∼0.0087 (cm V−1)1/2. Compound 3a showed a hole charge mobility of 2.8 × 10−4 cm2 V−1 s−1 at a electric field of 6.4 × 105 V cm−1, which is relatively high and useful for practical applications.
Organic light-emitting diodes
The optical and electrochemical properties of compound 3a, the high quantum yield in the solid state, together with the suitable blue CIE coordinates and hole mobility values confer on 3a the sought characteristics for an efficient luminescent dye in the deep blue emission range. On the other hand, film morphology of the emitting layer strongly influences the performance of the organic devices. Tapping mode Atomic Force Microscopy (AFM) was performed in order to characterize the morphology of the thin-film based on compound 3a (Fig. 3). 25 nm thick films were prepared by spin casting of a chlorobenzene solution of compound 3a on a PEDOT:PSS treated ITO substrate. AFM images show homogeneous and continuous thin-films of compound 3a prepared by solution processing with a root-mean-square (rms) roughness of ∼1.46 nm, showing appropriate morphology layer characteristics.
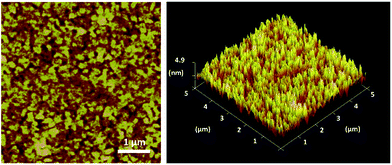 |
| Fig. 3 AFM image of a solution-processed 3a based thin-film from two different points of view (5 × 5 μm2). | |
Consequently, the molecule 3a has been tested as emissive layer in non-doped single-layer light emitting diodes. The here presented OLED configuration consisted on ITO/PEDOT:PSS (25 nm)/3a (25–55 nm)/TPBi (10 nm)/LiF (1 nm)/Al (100 nm) (Fig. 4a). In order to optimize the performance of the devices different thicknesses, from 25 to 55 nm, of the 3a layer were prepared from chlorobenzene solution by spin coating. The performance characteristics of the different devices are summarized in Fig. 4b and c and Table 2. Deposition of the emissive layer of compound 3a was also tested from different solvent solutions, such as dichloromethane and tetrahydrofuran.
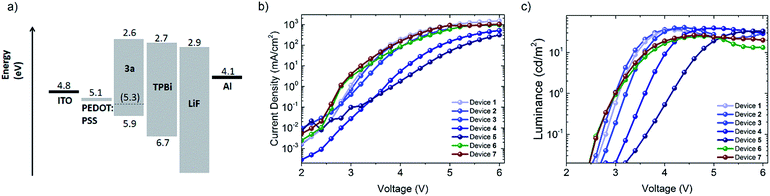 |
| Fig. 4 (a) Energetic scheme of the different components of the device structure used in this study. The dashed line in 3a component indicates the ionization potential obtained from the photo-emission in air technique. This value is displayed in parentheses. (b) Current density–voltage and (c) luminance–voltage characteristics of the devices summarized in Table 2. | |
Table 2 Data of best OLED devices based on compound 3aa
Device |
Solventb |
dc (nm) |
Vtd (V) |
Lmaxe (cd m−2) |
ηcf (cd A−1) |
Average values are reported in Table S1 in the ESI. Solvent used for preparing the 3a based layer by spin-coating (CB: chlorobenzene, DCM: dichloromethane, THF: tetrahydrofuran). Thickness of the 3a based layer measured with a profilometer. Turn-on voltage defined as voltage corresponding to a luminance of 0.1 cd m−2. Maximum luminance. Maximum current efficiency. |
1 |
CB |
25 |
2.80 |
35.59 |
0.08 |
2 |
CB |
30 |
2.68 |
37.52 |
0.09 |
3 |
CB |
40 |
2.88 |
40.98 |
0.09 |
4 |
CB |
50 |
3.23 |
39.46 |
0.17 |
5 |
CB |
55 |
3.62 |
33.49 |
0.05 |
6 |
DCM |
25 |
2.61 |
24.75 |
0.03 |
7 |
THF |
25 |
2.62 |
26.47 |
0.03 |
All the devices present competitive turn-on voltages between 2.6 and 3.6 V indicating small injection barriers from the transporting layers in the device. The luminance increases upon voltage application, reaching the maximum values below 5 V. The maximum luminance values are dependent on the thickness of the active layer, reaching the optimum ratio between luminance and current efficiency at 50 nm. Further increase of the thickness leads to a decrease of the maximum luminance and efficiency together with an increase of the turn-on voltage, pointing to growing unbalance of the charge transport in the device. On the other hand, the devices prepared from DCM and THF solutions show poorer performances suggesting the influence of the solvent on the final morphology of the active layer.
Conclusions
Tricarbazoles 3a and 3b show the sought deep blue emission in the solid state, as a result of linking the three carbazole units by a triple bond as a π-spacer. In addition, alkylation of the nitrogen positions of the lateral carbazole units with 2-ethylhexyl chains transfers the quantum yield determined in solution to that in the solid state without significant reduction. Solution processed non-doped OLED devices from compound 3a demonstrates its potential as a blue deep emitter in non-doped devices, reaching luminances of up to 40 cd m−2 and current efficiency of 0.2 cd A−1.
Experimental
Materials
All chemicals were of commercial grade and used as received. All solvents were dried and degassed by standard methods. Tetrahydrofuran was distilled from sodium/benzophenone. The starting materials 3-iodo-9H-carbazole (MIC) and 3,6-diiodo-9H-carbazole (DIC) were obtained according to the procedures described in the literature.8 3,6-Diethynyl-9H-carbazole was synthesized from 3,6-diiodo-9H-carbazole according to reported procedures.6
Synthesis of 9-(2-ethylhexyl)-3-iodo-9H-carbazole (1)
1.20 g (4.09 mmol) of 3-iodo-9H-carbazole dissolved in 10 mL of DMF, 2.37 g (12.27 mmol, 2.18 mL) of 2-ethylhexyl bromide, 0.92 g (16.36 mmol) of KOH and 0.58 g (4.09 mmol) of anhydrous Na2SO4 were stirred at room temperature for 10 min. After termination of the reaction, the reaction mixture was diluted with water and the product was extracted with ethyl acetate. The organic layer was washed thoroughly with water until the aqueous layer was neutral, dried over anhydrous MgSO4, filtered off and the solvent was distilled off under reduced pressure. The crude was purified by flash column chromatography using hexane as the eluent. The yield was 1.30 g (80%). 1H NMR (400 MHz, d6-acetone) δ (ppm): 8.50 (d, J = 1.7 Hz, 1H), 8.18 (d, J = 7.8 Hz, 1H), 7.72 (dd, J1 = 8.6 Hz, J2 = 1.7 Hz, 1H), 7.56 (d, J = 8.3 Hz, 1H), 7.51–7.47 (m, 1H), 7.43 (d, J = 8.6 Hz, 1H), 7.25–7.21 (m, 1H), 4.30 (d, J = 7.6 Hz, 2H), 2.14–2.07 (m, 1H), 1.46–1.17 (m, 8H), 0.91 (t, J = 7.5 Hz, 3H), 0.81 (t, J = 7.2 Hz, 3H).
Synthesis of 9-(3,5-di-tert-butylphenyl)-3-iodo-9H-carbazole (2)
4.50 g (15.35 mmol) of 3-iodo-9H-carbazole, 12.40 g (46.05 mmol) of 1-bromo-3,5-di-tert-butylbenzene, 4.24 g (30.70 mmol) of anhydrous K2CO3 and 0.13 g (1.99 mmol) of Cu powder was refluxed in 35 mL of anhydrous DMF under nitrogen atmosphere for 72 h. After, the reaction mixture was filtered through Celite and diluted with water. The product was extracted with ethyl acetate and the organic layer was washed thoroughly with water until the aqueous layer was neutral. The organic extract was dried over anhydrous MgSO4, filtered off and the solvent was distilled off under reduced pressure. The residue was purified by column chromatography using hexane as the eluent. The yield was 1.51 g (21%). 1H NMR (400 MHz, d6-acetone) δ (ppm): 8.59 (d, J = 1.7 Hz, 1H), 8.27 (d, J = 7.8 Hz, 1H), 7.71 (dd, J = 8.6 Hz, J = 1.7 Hz, 1H), 7.67 (t, J = 1.7 Hz, 1H), 7.50–7.45 (m, 3H), 7.41 (d, J = 8.2 Hz, 1H), 7.33–7.29 (m, 1H), 7.26 (d, J = 8.6 Hz, 1H), 1.42 (s, 18H). 13C NMR (100 MHz, d6-acetone) δ (ppm): 153.8, 141.8, 141.0, 137.3, 135.1, 130.0, 127.8, 126.7, 122.8, 122.6, 121.8, 121.5, 121.2, 113.0, 110.8, 82.6, 35.8, 31.7. HRMS (ESI-MS) (m/z): calcd for C26H29IN (M + H)+ 482.1339, found: 482.1348.
Synthesis of 3,6-bis[2-(9-(2-ethylhexyl)-9H-carbazol-3-yl)ethynyl]-9H-carbazole (3a)
1.19 g (2.94 mmol) of 9-(2-ethylhexyl)-3-iodo-9H-carbazole (1), 0.035 g (0.05 mmol) of Pd(PPh3)2Cl2 and 0.025 g (0.13 mmol) of CuI were dissolved in 10 mL of anhydrous THF under nitrogen atmosphere. Then, 0.35 g (3.43 mmol, 0.48 mL) of triethylamine and a solution of 3,6-diethynyl-9H-carbazole (0.21 g, 0.98 mmol) in THF were added and stirred at room temperature for 10 min. After termination of the reaction, 3a was isolated according to the procedure described for 2, using a mixture of hexane and ethyl acetate (9
:
1 v/v) as the eluent. The yield was 0.23 g (31%). 1H NMR (400 MHz, d6-acetone) δ (ppm): 10.74 (s, 1H, NH), 8.46 (s, 2H), 8.39 (d, J = 0.8 Hz, 2H), 8.25 (d, J = 7.9 Hz, 2H), 7.69–7.58 (m, 10H), 7.53–7.48 (m, 2H), 7.29–7.24 (m, 2H), 4.35 (d, J = 7.6 Hz, 4H), 2.18–2.10 (m, 2H), 1.50–1.19 (m, 16H), 0.94 (t, J = 7.4 Hz, 6H), 0.83 (t, J = 7.2 Hz, 6H). 13C NMR (100 MHz, CDCl3) δ (ppm): 141.4, 140.6, 139.3, 130.0, 129.3, 126.1, 124.1, 124.0, 123.3, 123.0, 122.6, 120.6, 119.4, 115.4, 113.8, 110.9, 109.3, 109.2, 89.3, 88.6, 47.7, 39.5, 31.2, 28.9, 24.6, 23.2, 14.2, 11.1. HRMS (ESI-MS) (m/z): calcd for C56H56N3 (M + H)+ 770.4469, found: 770.4437.
Synthesis of 3,6-bis[2-(9-(3,5-di-tert-butylphenyl)-9H-carbazol-3-yl)ethynyl]-9H-carbazole (3b)
Method A. 3b was prepared and isolated according to the procedure described for 3a, using 0.11 g (0.51 mmol) of 3,6-diethynyl-9H-carbazole, 0.74 g (1.53 mmol) of 2, 0.018 g (0.026 mmol) of Pd(PPh3)2Cl2, 0.013 g (0.07 mmol) of CuI and 0.18 g (1.78 mmol, 0.25 mL) of triethylamine (TEA). The product was purified using a mixture of hexane and tetrahydrofuran (9
:
1 v/v) as the eluent. The yield was 0.07 g (15%).
Method B. 3b was prepared and isolated according to the procedure described for 3a, using 0.73 g (1.92 mmol) of 5, 0.27 g (0.64 mmol) of 3,6-diiodo-9H-carbazole 0.021 g (0.03 mmol) of Pd(PPh3)2Cl2, 0.015 g (0.08 mmol) of CuI and 0.23 g (2.24 mmol, 0.32 mL) of triethylamine. The product was purified by flash column chromatography using a mixture of hexane and ethyl acetate (4
:
1 v/v) as the eluent. The yield was 0.14 g (24%). 1H NMR (400 MHz, d6-acetone) δ (ppm): 10.76 (s, 1H, NH), 8.48–8.47 (m, 4H), 8.33 (d, J = 7.8 Hz, 2H), 7.69–7.65 (m, 6H), 7.61 (d, J = 8.4 Hz, 2H), 7.50 (d, J = 1.7 Hz, 4H), 7.49–7.43 (m, 6H), 7.36–7.32 (m, 2H), 1.44 (s, 36H). 13C NMR (100 MHz, d6-acetone) δ (ppm): 153.8, 142.3, 141.1, 140.9, 137.5, 130.4, 130.3, 127.5, 124.6, 124.4, 124.3, 123.7, 123.7, 122.6, 121.9, 121.5, 121.2, 115.9, 115.4, 112.3, 111.0, 110.9, 89.8, 89.4, 35.8, 31.7. MS (MALDI-TOF) (m/z): calcd for C68H63N3 (M+) 921.5, found: 921.5.
Synthesis of 9-(3,5-di-tert-butylphenyl)-3-[2-(trimethylsilyl)ethynyl]-9H-carbazole (4)
1.33 g (2.76 mmol) of 2, 0.10 g (0.14 mmol) of Pd(PPh3)2Cl2, 0.07 g (0.36 mmol) of CuI were dissolved in 10 mL of anhydrous THF under nitrogen atmosphere. Then, 0.98 g (9.68 mmol, 1.35 mL) of triethylamine and 0.54 g (5.50 mmol, 0.78 mL) of ethynyltrimethylsilane dissolved in THF were added and stirred at room temperature for 10 min. 4 was purified according to the procedure described for 2. The yield was 1.23 g (98%). 1H NMR (400 MHz, d6-acetone) δ (ppm): 8.35 (d, J = 1.6 Hz, 1H), 8.30 (d, J = 7.7 Hz, 1H), 7.67 (t, J = 1.8 Hz, 1H), 7.52 (dd, J1 = 8.5 Hz, J2 = 1.6 Hz, 1H), 7.49–7.45 (m, 3H), 7.41 (d, J = 8.1 Hz, 1H), 7.38 (d, J = 8.5 Hz, 1H), 7.34–7.30 (m, 1H), 1.42 (s, 18H), 0.27 (s, 9H). 13C NMR (100 MHz, d6-acetone) δ (ppm): 153.8, 142.3, 141.4, 137.3, 130.6, 127.6, 125.0, 124.1, 123.6, 122.6, 121.8, 121.5, 121.3, 115.1, 110.9, 110.8, 107.4, 92.2, 35.8, 31.7, 0.2. HRMS (ESI-MS) (m/z): calcd for C31H38NSi (M + H)+ 452.2768, found: 452.2759.
Synthesis of 9-(3,5-di-tert-butylphenyl)-3-ethynyl-9H-carbazole (5)
1.2 g (2.66 mmol) of 4 and 1.84 g (13.31 mmol) of anhydrous K2CO3 were dissolved in 180 mL of methanol. The reaction mixture was stirred at room temperature for 24 h. After termination of the reaction, the solvent was distilled off under reduced pressure and the product was purified according to the procedure described for 2. The yield was 0.81 g (80%). 1H NMR (400 MHz, d6-acetone) δ (ppm): 8.38 (d, J = 1.6 Hz, 1H), 8.28 (d, J = 7.8 Hz, 1H), 7.67 (t, J = 1.8 Hz, 1H), 7.55 (dd, J1 = 8.5 Hz, J2 = 1.6 Hz, 1H), 7.49–7.45 (m, 3H), 7.42 (d, J = 8.3 Hz, 1H), 7.39 (d, J = 8.5 Hz, 1H), 7.34–7.30 (m, 1H), 3.57 (s, 1H), 1.42 (s, 18H). 13C NMR (100 MHz, d6-acetone) δ (ppm): 153.3, 141.7, 140.9, 136.8, 130.3, 127.3, 124.9, 123.7, 123.0, 122.2, 121.4, 121.3, 121.0, 113.9, 110.6, 110.5, 85.0, 78.0, 35.4, 31.4. HRMS (ESI-MS) (m/z): calcd for C28H30N (M + H)+ 380.2373, found: 380.2363.
Instrumentation and methods
Silica gel (grade 62, 60–200 mesh, 150 Å, Aldrich) was used for flash column chromatography. 1H NMR (400 MHz) and 13C NMR (100 MHz) were collected on a Varian Mercury spectrometer. MALDI-TOF was performed on an Applied Biosystems MDS SCIEX 4800 equipment. High-resolution mass spectroscopy (HR-MS) was performed on a LC/MSD-TOF Agilent Technologies apparatus by means of the electrospray (ESI-MS) technique. UV-vis spectra were registered in a Varian Cary UV-Vis-NIR 500E spectrophotometer. Emission spectra in solution were recorded in a PTI fluorimeter equipped with a 220B lamp power supply, a 815 photomultiplier detection system and a Felix 32 software after excitation at 300 nm and at a concentration of 10 μM in dichloromethane. Photophysical measurements in the solid state were done on films deposited from a 1 mM dichloromethane solution by spin coating at 1500 rpm for 20 s and at 3500 rpm for 10 s followed by 5 min of thermal annealing at 50 °C. Quantum yield measurements in solution and in the solid state were determined after excitation at 300 nm using optically-matched solutions of 1,4-bis(5-phenyl-2-oxazolyl)benzene (POPOP) as the standard (ϕ = 0.93 in cyclohexane) following the reported methods.12 Colour rendering indexes were calculated after excitation at 310 nm. Cyclic voltammograms were carried out in a microcomputer-controlled potentiostat/galvanostat Autolab with PGSTAT30 equipment and GPES software. A cylindrical three-electrode cell was used. The reference electrode was a Metrohm Ag/AgCl/KCl (3 M) mounted in a Luggin capillary containing a 0.1 M solution of tetrabutylammonium perchlorate (TBAP) in dichloromethane. The counter and working electrodes were a platinum spiral and a platinum wire, respectively. All voltammetric curves were recorded under quiescent conditions, at a scan rate of 100 mV s−1 and under argon atmosphere. All solutions were prepared in dichloromethane (1 mM). TBAP (Aldrich, electrochemical grade) was used as the supporting electrolyte. The ionization potential (IP) values were estimated from the onset of the first oxidation peak as IP = Eoxonset − E0(Fc/Fc+) + 5.39, where E0(Fc/Fc+) = +0.43 V vs. Ag/AgCl/KCl (3 M) and where 5.39 eV corresponds to the formal potential of the Fc/Fc+ redox couple in the Fermi scale.13 The electron affinity values (EA) were calculated as EA = IP − Egap. The optical gap energy (Egap) was estimated from λonset of the absorption spectra. Ionization potential was measured by the photo-emission in air method.14 The samples for the hole mobility measurements were prepared by spin-coating the solutions of the synthesized compounds on the polyester films with conductive Al layer. The layer thickness was in the range 1.8–6 μm. The hole drift mobility was measured by the XTOF technique.15 Positive corona charging created electric field inside the TM layer. Charge carriers were generated at the layer surface by illumination with pulses of N2 laser (pulse duration was 2 ns, wavelength 337 nm). The layer surface potential decrease as a result of pulse illumination was up to 1–5% of the initial potential before illumination. The capacitance probe that was connected to the wide frequency band electrometer measured the rate of the surface potential decrease, dU/dt. The transit time tt was determined by the kink on the curve of the dU/dt transient in the log–log scale. The drift mobility was calculated by the formula μ = d2/U0tt, where d is the layer thickness and U0 is the surface potential at the moment of illumination. AFM experiments were conducted using an AFM Multimode 8 system attached to a Nanoscope V electronic unit (Bruker).
OLED device fabrication and measurements
The substrates were cleaned by ultrasonic treatment in acetone and isopropyl alcohol and subsequently dried by a nitrogen blow. After that, their surfaces were processed with ozone treatment for 30 minutes. Subsequently, PEDOT:PSS (Clevios PV P AI4083 from H.C. Starck, filtered at 0.45 μm) was spin-coated at 4500 rpm (thickness around 25 nm) onto the ITO surface and lately baked at 120 °C for 20 min. After that, starting from a solution of 10 mg mL−1, 3a was spin casted from different solvents on the PEDOT:PSS layer and successively heated at 50 °C for 5 min. Samples were transferred into a nitrogen filled glovebox where 10 nm of TPBi, 1 nm of LiF and 100 nm of Al were deposited by thermal evaporation on the active layer under high vacuum. The devices were put in a sample holder that protect them from oxygen and moisture and allow contacting them for measuring. The effective area of each cell was ∼9 mm2. The current density–voltage and luminance–voltage data were simultaneously acquired by a Keithley 2400 unit (current) and a Konica-Minolta LS-100 (luminance).
Acknowledgements
Financial support from the Ministerio de Economía y Competitividad (CTQ2012-36074) is gratefully acknowledged. MR is grateful for the grant ADR from Universitat de Barcelona (UB). EP thanks MINECO project CTQ2013-47183 as well as the Severo Ochoa Excellence Accreditation 2014–2018 (SEV-2013-0319). EP is also grateful to ICIQ and ICREA for economical support. EMF acknowledges MINECO for the project MAT2012-31570 and Ramon y Cajal fellowship (RyC-2010-06787). V. Gaidelis, Department of the Solid State Electronic, Vilnius University, is thanked for the measurements of ionization potentials.
Notes and references
- X. Yang, X. Xu and G. Zhou, J. Mater. Chem. C, 2015, 3, 913 RSC.
-
(a) L. Wang, Y. Jiang, J. Luo, Y. Zhou, J. Zhou, J. Wang, J. Pei and Y. Cao, Adv. Mater., 2009, 21, 4854 CrossRef CAS PubMed;
(b) J.-H. Jou, J.-R. Tseng, K.-Y. Tseng, W.-B. Wang, Y.-C. Jou, S.-M. Shen, Y.-L. Chen, W.-Y. Hung, S.-Z. Chen, T.-Y. Ding and H.-C. Wang, Org. Electron., 2012, 13, 2893 CrossRef CAS;
(c) D. Chercka, S.-J. Yoo, M. Baumgarten, J.-J. Kim and K. Müllen, J. Mater. Chem. C, 2014, 2, 9083 RSC;
(d) M. Zhu and C. Yang, Chem. Soc. Rev., 2013, 42, 4963 RSC;
(e) X. Tang, Q. Bai, Q. Peng, Y. Gao, J. Li, Y. Liu, L. Yao, P. Lu, B. Yang and Y. Ma, Chem. Mater., 2015, 27, 7050 CrossRef CAS.
-
(a) M. Reig, J. Puigdollers and D. Velasco, J. Mater. Chem. C, 2015, 3, 506 RSC;
(b) S. Chen, B. Sun, W. Hong, Z. Yan, H. Aziz, Y. Meng, J. Hollinger, D. S. Seferos and Y. Li, J. Mater. Chem. C, 2014, 2, 1683 RSC;
(c) P.-L. T. Boudreault, S. Wakim, M. L. Tang, Y. Tao, Z. Bao and M. Leclerc, J. Mater. Chem., 2009, 19, 2921 RSC;
(d) N. Drolet, J.-F. Morin, N. Leclerc, S. Wakim, Y. Tao and M. Leclerc, Adv. Funct. Mater., 2005, 15, 1671 CrossRef CAS;
(e) C. Beginn, J. V. Grazulevicius and P. Strohriegl, Macromol. Chem. Phys., 1994, 195, 2353 CrossRef CAS.
-
(a) M. Yu, S. Wang, S. Shao, J. Ding, L. Wang, X. Jing and F. Wang, J. Mater. Chem. C, 2015, 3, 861 RSC;
(b) F. Niu, H. Niu, Y. Liu, J. Lian and P. Zeng, RSC Adv., 2011, 1, 415 RSC;
(c) S. H. Kim, I. Cho, M. K. Sim, S. Park and S. Y. Park, J. Mater. Chem., 2011, 21, 9139 RSC;
(d) S.-L. Lin, L.-H. Chan, R.-H. Lee, M.-Y. Yen, W.-J. Kuo, C.-T. Chen and R.-J. Jeng, Adv. Mater., 2008, 20, 3947 CrossRef CAS.
- R. Chinchilla and C. Nájera, Chem. Rev., 2007, 107, 874 CrossRef CAS PubMed.
- S. Shanmugaraju, A. K. Bar, K.-W. Chi and P. S. Mukherjee, Organometallics, 2010, 29, 2971 CrossRef CAS.
-
(a) V. Vaitkeviciene, S. Grigalevicius, J. V. Grazulevicius, V. Jankauskas and V. G. Syromyatnikov, Eur. Polym. J., 2006, 42, 2254 CrossRef CAS;
(b) A. Tomkeviciene, T. Bartiuk, A. Bucinskas, J. V. Grazulevicius and V. Jankauskas, React. Funct. Polym., 2011, 71, 796 CrossRef CAS.
- S. H. Tucker, J. Chem. Soc., 1926, 129, 546, 10.1039/JR9262900546.
- S.-I. Kato, H. Noguchi, A. Kobayashi, T. Yoshihara, S. Tobita and Y. Nakamura, J. Org. Chem., 2012, 77, 9120 CrossRef CAS PubMed.
-
(a) A. Bucinskas, G. Bagdziunas, A. Tomkeviciene, D. Volynyuk, N. Kostiv, D. Gudeika, V. Jankauskas, M. Rutkis and J. V. Grazulevicius, RSC Adv., 2015, 5, 49577 RSC;
(b) A. Tomkeviciene, J. V. Grazulevicius, K. Kazlauskas, A. Gruodis, S. Jursenas, T.-H. Ke and C.-C. Wu, J. Phys. Chem. C, 2011, 115, 4887 CrossRef CAS.
-
(a) N. A. Kukhta, D. Volyniuk, L. Peciulyte, J. Ostrauskaite, G. Juska and J. V. Grazulevicius, Dyes Pigm., 2015, 117, 122 CrossRef CAS;
(b) R. R. Reghu, H. K. Bisoyi, J. V. Grazulevicius, P. Anjukandi, V. Gaidelis and V. Jankauskas, J. Mater. Chem., 2011, 21, 7811 RSC.
-
(a) G. A. Crosby and J. N. Demas, J. Phys. Chem., 1971, 75, 991 CrossRef CAS;
(b) J. Stampfl, S. Tasch, G. Leising and U. Scherf, Synth. Methods, 1995, 71, 2125 CrossRef CAS.
-
(a) C. M. Cardona, W. Li, A. E. Kaifer, D. Stockdale and G. C. Bazan, Adv. Mater., 2011, 23, 2367 CrossRef CAS PubMed;
(b) A. J. Bard and L. R. Faulkner, Electrochemical Methods: Fundamentals and Applications, WILEY-VCH, New York, 2001 Search PubMed.
-
(a) E. Miyamoto, Y. Yamaguchi and M. Yokoyama, Electrophotography, 1989, 28, 364 CAS;
(b) M. Daskeviciene, V. Getautis, J. V. Grazulevicius, A. Stanisauskaite, J. Antulis, V. Gaidelis, V. Jankauskas and J. Sidaravicius, J. Imaging Sci. Technol., 2002, 46, 467 CAS.
- E. Montrimas, V. Gaidelis and A. Pazera, Lith. J. Phys., 1966, 6, 569 CAS.
Footnote |
† Electronic supplementary information (ESI) available: 1H NMR, 13C NMR and mass spectra, cyclic voltammograms, XTOF characteristics, average values of OLED devices and digital picture of an OLED device. See DOI: 10.1039/c5ra21959k |
|
This journal is © The Royal Society of Chemistry 2016 |