DOI:
10.1039/C5RA21131J
(Paper)
RSC Adv., 2016,
6, 119-131
Synthesis, 64Cu-labeling and PET imaging of 1,4,7-triazacyclononane derived chelators with pendant azaheterocyclic arms†‡
Received
12th October 2015
, Accepted 11th December 2015
First published on 14th December 2015
Abstract
The TACN derived chelators NOTI, NOTI-Me and NOTThia were synthesized in a facile, single reaction step. The new chelators were readily labeled with 64Cu under mild conditions over a wide pH range. The corresponding 64Cu complexes are of high stability in vitro as determined by Cu2+ exchange experiments, acid decomplexation and serum stability studies. Single crystal X-ray analysis showed that the six-coordinate copper complexes exhibited a distorted prismatic CuN3N′3 coordination geometry similar to Cu(NOTA). Biological testing by small animal PET imaging revealed retention of 64Cu complexes in the kidneys and, to a lesser extent, in the liver. Altogether, the results presented support the development of bifunctional derivatives for conjugation to targeting vectors and further testing in vivo.
Introduction
In the field of nuclear medicine, metal complexes in their radioactive form play a pivotal role as an essential part of radiopharmaceuticals for diagnosis and therapy of various diseases.1–10 In recent years, medically relevant radionuclides of copper such as 60Cu, 61Cu, 62Cu, 64Cu and 67Cu have received a great deal of attention.11–15 The interest originates in particular from the positron-emitting radionuclide 64Cu that has favorable decay characteristics for PET (positron emission tomography) because of its low positron energy (Eβ+,max = 653 keV; 18%), which is comparable to that of the gold standard 18F (Eβ+,max = 633 keV). Furthermore, 64Cu has a reasonably long half-life of 12.7 h that allow for delayed imaging and investigation of biological processes at later time points making 64Cu compatible with slow pharmacokinetic molecules such as antibodies.16 The radionuclide 64Cu can be produced on a large scale at high specific activities and is also available from commercial suppliers. The dual decay characteristics of 64Cu with positron and beta (Eβ−,max = 578 keV; 37%) emissions allow diagnostic PET imaging and, in principle, radionuclide therapy with the same radiopharmaceutical.17
The major limitation to the widespread use of radioactive copper isotopes in nuclear medicine is the labile character of the Cu2+ cation, which may result in significant decomplexation and/or transchelation in vivo and subsequent accumulation of 64Cu in non-target organs.18–21 As a consequence, various ligands of different structural types have been proposed for use with 64Cu, many of which are based on cyclic polyamines including cyclam (1,4,8,11-tetraazacyclotetradecane) derivatives such as TETA (1,4,8,11-tetraazacyclotetradecane-1,4,8,11-tetraacetic acid) and cyclen (1,4,7,10-tetraazacyclododecane) type ligands such as DOTA (1,4,7,10-tetraazacyclododecane-1,4,7,10-tetraacetic acid) (Scheme 1). However, despite their ability to form Cu2+ complexes of very high thermodynamic stability both TETA and DOTA suffer from limited kinetic inertness leading to decomplexation in vivo.22 In order to overcome the lack of kinetic inertness, more rigid cage-like sarcophagine type ligands such as diamsar23–29 as well as cross-bridged ligands such as CB-TE2A23,30–35 were subsequently developed (Scheme 1). While increased rigidity of the ligand backbone in e.g. CB-TE2A makes these complexes less prone to dissociation, it also results in slow formation rates, which is hampering their biological application in combination with sensitive biomolecules such as antibodies. In contrast, bispidines label 64Cu under mild conditions and form complexes of high kinetic inertness.36–38 More recently, it has been shown that NOTA and derivatives, such as NODAGA (Scheme 1) also form 64Cu complexes of high stability/inertness in vivo and corresponding radiopharmaceuticals have been used successfully in PET imaging.39–44 In spite of these advances, there is still a high demand for an optimal chelator for 64Cu that provides sufficient in vivo stability, quantitative and fast radiolabeling as well as favorable pharmacokinetics (for detailed reviews see ref. 22 and 45).
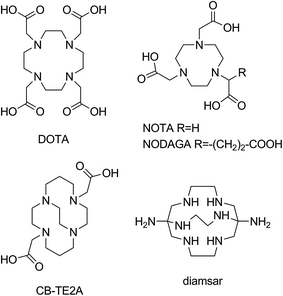 |
| Scheme 1 Common ligands for 64Cu chelation. | |
The macrocycle TACN (1,4,7-triazacyclononane) coordinates facially to Cu2+ with the metal lying out of the plane defined by the three nitrogen atoms forming exclusively five-membered chelate rings.46 The preorganized geometry of the TACN ring leads to favorable conformations of the nitrogen donors maximizing orbital overlap.46 Combination with additional donor atoms to saturate the coordination sphere of the metal cation of interest leads to metal complexes of high thermodynamic stability and kinetic inertness.46 In this respect, TACN appears to be an optimal starting point for the development of copper complexing agents for radiopharmaceutical applications.
While many chelators for copper are based on cyclic polyamines in combination with various substituents providing different O- and N-donor atoms, macrocyclic ligands bearing azaheterocyclic substituents providing aromatic N-donors have received less attention.47–50 Among heterocyclic amine donors, five-membered azaheterocycles such as imidazole form Cu2+ complexes of high thermodynamic stability.51 From the outset, our hypothesis was that a chelating system that combines the advantages of macrocyclic polyamines in terms of kinetic inertness with the excellent donor properties of five-membered heterocyclic amines may facilitate thermodynamically and kinetically stable copper complexation. Herein, we report the synthesis, complex formation and 64Cu-labeling of a novel class of hexadentate macrocyclic polyamines. In addition, we evaluated the stability/inertness of corresponding copper complexes in vitro and by PET imaging studies in vivo.
Experimental
General
Chemicals and solvents were purchased from Sigma-Aldrich and TCI Europe. All chemicals and reagents were of the highest grade commercially available and used without further purification. Samples of 1,4,7-triazacyclononane were kindly provided by Chematech (Dijon, France). 1H and 13C NMR spectra were measured in D2O at room temperature using a Bruker DRX Avance 250 MHz NMR spectrometer. Chemical shifts are given in parts per million (ppm) and are reported relative to trimethylsilyl propionate (TPS). Coupling constants are reported in hertz (Hz). The multiplicity of the NMR signals is described as follows: s = singlet, d = duplet, t = triplet, q = quartet, m = multiplet. Mass spectra were measured using Thermo Scientific Exactive mass spectrometer. Elemental analysis was performed using a Vario EL instrument from Elementaranalysensysteme GmbH. UV/vis spectra were recorded on a Molecular Devices SpectraMax Plus spectrometer (H2O, 25 ± 3 °C). 64CuCl2 was purchased from the Department of Preclinical Imaging and Radiopharmacy of the Eberhard-Karls-University (Tübingen, Germany). 64CuCl2 was produced via the 64Ni(p, n)64Cu route.52 The corresponding specific activity was 300–400 MBq nmol−1 (18 h EOB). Analytical HPLC was performed on a Knauer Smartline 1000 HPLC system with an Agilent 1200 DAD UV detector and Raytest Ramona radioactivity detector in series. A Chromolith performance RP-18e (100 × 4.6 mm) column (Merck) was used for analytical HPLC. The solvent system was A = H2O (0.1% TFA) and B = acetonitrile (0.1% TFA). The gradient was 0–5 min 0% B, 5–8 min 70% B, 8–10 min 70% B, 10–12 min 0% B and 12–15 min 0% B at a flow rate of 1 mL min−1. Semi-preparative HPLC was performed on a Knauer Smartline 1000 HPLC system in combination with a Macherey Nagel VP 250/21 Nucleosil 120-5 C18 column. The gradient was 0–1 min 5% B, 1–10 min 45% B, 10–11 min 50% B, 11–12 min 50% B, 12–14 min 5% B and 14–15 min 5% B at a flow rate of 15 mL min−1.
Caution: copper-64 emits β+ and β− radiation as well as annihilation (511 keV) photons. All work should therefore be performed in a specialized laboratory equipped for handling radioactive materials.
Ligand syntheses
1,4,7-Tris((1H-imidazol-2-yl)methyl)-1,4,7-triazonane (NOTI) (L1) and 1,4-bis((1H-imidazol-2-yl)methyl)-1,4,7-triazonane (NODI) (L2). Imidazole-2-carboxaldehyde (446 mg, 4.644 mmol) was suspended in water (10 mL) and 40% sodium hydroxide was added dropwise (∼10 drops) until a clear solution was obtained. Then, TACN (100 mg, 0.774 mmol) was added and the solution was allowed to stir at r.t. for 16 h, yielding a dark orange solution. Sodium borohydride (202 mg, 5.418 mmol) was then added in small portions (gas evolution) and the solution stirred for additional 5 h, during which the color changed to light orange. The crude reaction mixture was extracted with chloroform (3 × 30 mL), the combined organic layers were dried using anhydrous sodium sulfate, and the solvent was removed under vacuum. The crude product contained a mixture of L1 and L2. Both compounds were isolated by semi-preparative HPLC to give NOTI (48 mg, 0.130 mmol, 17%) and NODI (103 mg, 0.356 mmol, 46%) as light yellow solids. NOTI L1: 1H NMR (D2O): δ = 2.62 (s, 12H), 3.68 (s, 6H), 6.99 ppm (s, 6H); 13C NMR (D2O): δ = 43.4, 46.8, 48.9, 49.4, 119.4, 143.4 ppm. HR-ESI-MS calcd m/z for C18H28N9 (M + H): 370.2462, found: 370.2465. RP-HPLC (semipreparative): tR = 9
:
30 min. NODI L2 1H NMR (D2O): δ = 2.62 (s, 4H), 3.02 (t, J = 5.8, 4H), 3.26 (t, J = 5.8, 4H), 4.17 (s, 4H), 7.46 ppm (s, 4H); 13C NMR (D2O): δ = 43.3, 46.8, 49.0, 49.4, 119.4, 143.4 ppm. HR-ESI-MS calcd. m/z for C14H24N7 (M + H): 290.2088, found: 290.2088. RP-HPLC (semipreparative): NODI: tR = 8
:
30 min.
1,4,7-Tris((1-methyl-1H-imidazol-2-yl)methyl)-1,4,7-triazonane (NOTI-Me) (L3) and 1,4-bis((1H-imidazol-2-yl)methyl)-1,4,7-triazonane (NODI-Me) (L4). TACN (100 mg, 0.774 mmol) was dissolved in anhydrous 1,2-dichloroethane (10 mL) under an inert atmosphere (nitrogen) followed by the addition of 1-methyl-2-imidazole-carboxaldehyde (511 mg, 4.644 mmol). The solution was allowed to stir at r.t. for 30 min, during which a yellowish solution was obtained. Then, sodium triacetoxyborohydride (1.15 g, 5.418 mmol) was added in small portions (slight gas evolution). After complete addition, the suspension was allowed to stir for 16 h at r.t. The solvent was removed under reduced pressure, the residue redissolved in 20 mL methanol and the solvent removed once more. The residue contained L3 and a small amount of L4. The residue was purified by semi-preparative HPLC to give NOTI-Me (153 mg, 0.371 mmol, 48%) and NODI-Me (35 mg, 0.110 mmol, 14%) as light yellow oils. NOTI-Me L3: 1H NMR (D2O): δ = 2.78 (s, 12H), 3.65 (s, 6H), 3.68 (s, 9H), 6.93 (s, 3H), 7.09 ppm (s, 3H); 13C NMR (D2O): δ = 32.8, 52.6, 53.9, 122.8, 125.8, 145.9 ppm. HR-ESI-MS calcd m/z for C21H34N9 (M + H): 412.3921, found: 412.2931. RP-HPLC (semipreparative): tR = 9
:
30 min. NODI-Me L4: 1H NMR (D2O): δ = 2.69 (s, 4H), 3.05 (t, J = 5.7, 4H), 3.29 (t, J = 5.7, 4H), 3.86 (s, 6H), 4.20 (s, 4H), 7.46 ppm (s, 4H); 13C NMR (D2O): δ = 34.4, 43.7, 47.5, 48.3, 50.4, 118.8, 124.2, 143.2 ppm. HR-ESI-MS calcd m/z for C16H28N7 (M + H): 318.2401, found: 318.2401. RP-HPLC (semipreparative): tR = 8
:
30 min.
1,4,7-Tris(thiazol-2-ylmethyl)-1,4,7-triazonane (NOTThia) (L5). 1,4,7-Triazacyclononane (100 mg, 0.774 mmol) was dissolved in anhydrous 1,2-dichloroethane (10 mL) under an inert atmosphere (nitrogen) followed by the addition of thiazole-2-carboxaldehyde (271 mg, 2.399 mmol) and sodium triacetoxyborohydride (984 mg, 4.644 mmol). The orange suspension was allowed to stir at r.t. for 16 h. Excess sodium triacetoxyborohydride was destroyed by dropwise addition of a concentrated sodium bicarbonate solution until no further gas evolution was observed. The product was extracted with chloroform (2 × 10 mL). The combined organic layers were washed with water (2 × 20 mL), dried using anhydrous sodium sulfate, filtered and evaporated to dryness to yield NOTThia L5 (277 mg, 0.658 mmol, 85%) as an orange oil. A small aliquot of the product was purified by semi-preparative HPLC for radiolabeling experiments. 1H NMR (D2O): δ = 3.16 (s, 12H), 4.55 (s, 6H), 7.84 (d, J = 3.5, 3H), 7.97 ppm (d, J = 3.4, 3H); 13C NMR (D2O): δ = 52.1, 56.9, 57.1, 125.8, 142.8, 168.9 ppm. HR-ESI-MS calcd m/z for C18H24N6S3 (M + H): 421.1297, found: 421.1297. RP-HPLC (semipreparative): tR = 9
:
40 min.
Preparation of metal complexes
[Cu(NOTI)H](ClO4)3·1.3H2O (Cu-1). Cu(ClO4)2·6H2O (5.5 mg, 0.01 mmol) and NOTI·6TFA (10.5 mg, 0.01 mmol) were dissolved in water (500 μL). Slow evaporation of the solvent at ambient temperature over a period of several days gave blue crystals suitable for single-crystal X-ray analysis. Elemental analysis calc. C: 26.39%, H: 3.04, N: 11.54, found C: 26.87%, H: 2.97, N: 10.88. HR-ESI-MS calcd m/z for C18H27N9O4CuCl (M + ClO4): 531.1170, found: 531.1165.
[Cu(NOTI-Me)H]2(ClO4)5·2TFA·4H2O (Cu-2). Cu(ClO4)2·6H2O (5.5 mg, 0.01 mmol) and NOTI-Me·6TFA (11.0 mg, 0.01 mmol) were dissolved in water (500 μL). Slow evaporation of the solvent at ambient temperature over a period of several days gave blue crystals suitable for single-crystal X-ray analysis. Elemental analysis calc. C: 33.78%, H: 4.81, N: 15.41, found C: 31.49%, H: 4.23, N: 15.32. HR-ESI-MS calcd m/z for C21H33N9O4CuCl (M + ClO4): 573.1640, found: 573.1635.
[Cu(NOTI-Me)](ClO4)2 (Cu-3). Cu(ClO4)2·6H2O (5.5 mg, 0.01 mmol) and NOTI-Me·6TFA (11.0 mg, 0.01 mmol) were dissolved in water (500 μL). Slow evaporation of the solvent at ambient temperature for several days gave blue crystals suitable for single-crystal X-ray analysis. Elemental analysis calc. C: 33.29%, H: 3.91, N: 13.98, found C: 30.89%, H: 4.31, N: 14.31. HR-ESI-MS calcd m/z for C21H33N9O4CuCl (M + ClO4): 561.2184, found: 561.2180.
[Cu(NOTThia)](ClO4)2 (Cu-4). Cu(ClO4)2·6H2O (14.0 mg, 0.04 mmol) and NOTThia (16.8 mg, 0.04 mmol) were each dissolved in water
:
acetonitrile (100 μL, 50
:
50 v/v). Upon combination of both solutions, green crystals were obtained that were suitable for single-crystal X-ray analysis. Elemental analysis calc. C: 31.65%, H: 3.54, N: 12.30, S: 14.08, found C: 31.42%, H: 3.59, N: 11.76, S: 13.42. HR-ESI-MS calcd m/z for C18H24N6S3O4CuCl (M + ClO4): 582.0005, found: 582.0000.
[Cu(NOTThia)](NO3)2 (Cu-5). Cu(NO3)2·6H2O (7.0 mg, 0.04 mmol) and NOTThia (16.8 mg, 0.04 mmol) were dissolved in water
:
methanol (500 μL, 50
:
50 v/v). Slow evaporation of the solvent at ambient temperature over several days gave green crystals suitable for single-crystal X-ray analysis. Elemental analysis calc. C: 35.55%, H: 3.98, N: 18.42, S: 15.82, found C: 35.67%, H: 4.11, N: 18.14, S: 15.21. HR-ESI-MS calcd m/z for C18H24N7S3O3Cu (M + NO3): 545.0398, found: 545.0391.
Single-crystal X-ray diffraction studies
Datasets were collected on a Bruker Apex-2 Quazar 3-circle diffractometer with Mo-Kα radiation (λ = 0.71073 Å, Incoatec iμS with mirror optics). The samples were cooled during data collection using an Oxford Cryosystems Cryostream 700 crystal thermostat. Structures were solved by direct methods with SHELXS or SHELXT53 and refined by full-matrix least-squares analysis (SHELXL) using the program package OLEX2.54 Data were corrected for absorption effects using the multi-scan method (SADABS).55 Unless otherwise indicated (see ESI for further details‡), all non-hydrogen atoms were refined anisotropically; hydrogen atoms were constrained to ideal geometries and refined with fixed isotropic displacement parameters (in terms of a riding model). CCDC 1043399 (Cu-1), CCDC 1043400 (Cu-2), CCDC 1043398 (Cu-3), CCDC 1043401 (Cu-4) and CCDC 1043403 (Cu-5) contain the supplementary crystallographic data for this paper, including coordinates, experimental details, refinement instructions and structure factors.
UV/vis titration experiments
Prior to titration experiments, electronic absorption spectra of the copper complexes of NOTI L1, NOTI-Me L3, NOTThia L5, NOTA, and DOTA (c = 1 mM) were measured in PBS at pH 7.4 in the range of 300–800 nm. The corresponding absorption maxima λmax and molar absorption coefficients ε for complexes of L1, L3, L5, NOTA, and DOTA are summarized in Table 1. UV/vis spectra are given as ESI.‡ Each ligand was titrated in PBS at pH 7.4 with CuCl2. The absorbance at λmax was then plotted against [CuII]/[ligand].
Table 1 Copper exchange, half-lives in 5 M HCl, serum stability, log
D and UV/vis absorption maxima of studied copper complexes
Complex |
Cu exchangea |
t1/2 in 5 M HClb |
Serum stabilityc |
log D |
λmaxf (ε) |
% exchanged after 24 h; 5-fold molar excess of CuCl2 compared to c(L). r.t. % intact complex at 24 h, 37 °C. 95 °C. Decomposition upon addition to 5 M HCl. mol−1 dm−3 cm−1. |
64Cu-1 |
1.78 ± 0.35% |
∼0.5 min |
>99% |
−3.12 ± 0.12 |
580 nm (139) |
64Cu-3 |
0.80 ± 0.23% |
∼0.5 min |
>99% |
−4.02 ± 0.95 |
700 nm (114) |
64Cu-4 |
0.52 ± 0.19% |
55 hd |
>99% |
−3.24 ± 0.22 |
650 nm (150) |
64Cu(NOTA) |
2.04 ± 0.11% |
—e |
>99% |
−3.64 ± 0.44 |
739 nm (44) |
64Cu(DOTA) |
65.8 ± 1.0% |
—e |
>99% |
−4.10 ± 0.03 |
735 nm (94) |
64Cu labeling experiments
For the preparation of 64Cu complexes, stock solutions for each chelator and the controls NOTA and DOTA were prepared in the appropriate labeling buffer with a concentration of 0.025 mM. The buffer solutions used for radiolabeling were 0.1 M NaOAc (pH 4.0), 0.1 M MES (pH 5.5), 0.1 M HEPES (pH 7.4), and 0.1 M NH4OAc (pH 8.0). In a typical labeling experiment, 5 μL of chelator stock were mixed with 119 μL of corresponding buffer. To this solution, 1 μL of 64CuCl2 in 0.1 M HCl (64Cu stock diluted to an activity concentration of ∼1–2 MBq μL−1) was added to give a final volume of 125 μL and a chelator concentration of 1 μM. Samples for analytical HPLC analysis were taken immediately after brief mixing of the labeling solution and after 30 min incubation time. The radiochemical conversion (RCC) were investigated for each chelator in the concentration range of 0.1–5 μM at ambient temperature and 95 °C, respectively. The identity of the 64Cu complexes was confirmed by the UV trace of the corresponding non-radioactive Cu2+ complex. Experiments were performed in triplicate. C18 RP-HPLC: tR(64Cu-1) = 3
:
26 min; tR(64Cu-3) = 8
:
45 min; tR(64Cu-4) = 9
:
06 min; tR([64Cu]Cu-NOTA) = 2
:
50 min; tR([64Cu]Cu-DOTA) = 3
:
26 min.
Serum stability studies
For each experiment, 1–2 MBq (20 μL) of the 64Cu complexes were added to human serum (200 μL, human male AB plasma, Sigma-Aldrich) in an 1.5 mL Eppendorf tube, which was pre-equilibrated at 37 °C in a cell incubator for 1 h. Samples were vortexed and stored at 37 °C in a cell incubator. At different time points, 50 μL aliquots were taken from the serum solution and serum proteins removed by centrifugation using centrifuge tubes (molecular weight cutoff 30 kD) at 4 G for 5 min at 4 °C. The filtrate was kept on ice until HPLC analysis. The percentage of intact 64Cu complex was calculated from the chromatograms. All experiments were performed in triplicate.
Measurement of copper exchange
Experimental conditions were chosen based on a procedure described by Maheshwari et al.56 Copper exchange was measured at pH 7.4 in 0.1 M HEPES buffer. Non-radioactive Cu2+ solution was prepared as a stock solution of CuCl2·2H2O with a concentration of 0.0625 mM. Test solutions were prepared by mixing the chelator solution, the 64Cu solution, and the buffer. After incubation of the test solutions for 15 min at 95 °C, the labeling yield was checked by analytical HPLC. The exchange experiment was then initiated by addition of a 5-fold molar excess of the non-radioactive Cu2+ solution. For example, after incubation of a solution containing 5 μL chelator stock, 1 μL 64Cu (∼1–2 MBq), and 109 μL buffer, 10 μL of non-radioactive Cu2+ solution was added. The final concentrations in this solution were 1 μM chelator and 5 μM non-radioactive Cu2+. The extent of loss of 64Cu from the chelators at ambient temperature was measured by analytical HPLC after 24 h.
Acid-decomplexation studies
Acid-decomplexation studies were performed according to a procedure described by Anderson and co-workers.57,58 The sample concentration of Cu2+ complexes was 1 mM. For each complex, the decrease of absorbance at λmax (Cu-NOTI 580 nm, Cu-NOTI-Me 730 nm, NOTThia 575 nm, NOTA 739 nm, and DOTA 739 nm) in 5 M HCl at 95 °C was recorded at specific time points to monitor the progress of the decomplexation reaction. For complexes with λmax interfering with the λmax of free Cu2+ the absorption was corrected accordingly. Half-lives were calculated from the slopes of the linear ln(absorbance) vs. time plots. The results are summarized in Table 1. A graphical representation showing the UV changes upon incubation in 5 M HCl for the NOTThia complex is given as ESI ‡.
Partition coefficient measurements
For each determination, ∼1–2 MBq of the corresponding 64Cu complex in water (20 μL) was added to a mixture of phosphate buffered saline pH 7.4 (PBS) (480 μL) and octanol (500 μL) in 1.5 mL Eppendorf tubes. Samples were shaken for 1 h at room temperature. For separation, the samples were then centrifuged at 13
200 rpm for 5 min. From each sample, three 100 μL samples of both the water and the organic layer were transferred into plastic tubes, and samples were counted using a Packard Cobra gamma counter. Experiments were performed in triplicate.
Small animal PET imaging studies
Small animal PET studies were carried out in accordance with the German Animal License Regulations and were approved by the animal care committee of the Regierungspräsidium Freiburg. Imaging was performed on a Focus 120 microPET scanner. For PET imaging studies, nude mice (balb/c nude, Janvier) were anesthetized with isoflurane (2–4% in oxygen) and placed on the scanner. Immediately after scan start, animals were injected with 100 μL of each 64Cu complex (3.7–5.6 MBq) in saline via a catheter into the tail vein. Data were acquired for 45 min in list mode. Reconstruction was performed using unweighted OSEM2D. Image analysis was performed using AMIDE. Time-activity curves (TACs) were constructed by manually drawing regions of interest (ROI) in the liver and the kidneys. All ROIs were then copied on each of the frames, and time-activity curves of the ROI mean values were generated. TACS of the liver and kidney uptake are given as ESI.‡
Results and discussion
For radiopharmaceutical applications, the optimal chelator for divalent copper should provide a six-coordinate geometry in order to maximize complex stability and inertness. In the present study, we chose the macrocycle TACN as building block for a series of chelators due to its excellent metal binding properties for first row transition metal cations.46 TACN only provides three nitrogen donor atoms for metal binding and, consequently, additional donor groups need to be introduced into the macrocycle. A comparison of the pKa values and complex formation constants of several azaheterocyclic amine derivatives prompted us to choose imidazole and thiazole residues to provide a total of six nitrogen donors for Cu2+ complexation.51 The Cu2+ cation has a high affinity to heterocyclic nitrogen donors as exemplified by their corresponding complex formation constants for imidazole (log
β1 = 4.21) and 1-methylimidazole (log
β1 = 4.22).51 The ligand NOTA and its derivatives possess excellent complexation properties for 64Cu. However, the affinity of the acetic acid substituents to Cu2+ is lower compared to imidazole derivatives as can be seen from the corresponding complex formation constants (acetic acid, log
β1 = 1.79 vs. imidazole, log
β1 = 4.21 and 1-methylimidazole, log
β1 = 4.22). There is also a difference in acidity of the azaheterocycles compared to acetic acid illustrated by the pKa values of acetic acid, imidazole, 1-methylimidazole and thiazole with 4.56, 7.00, 7.10 and 2.80, respectively.51 In this respect, a metal chelator based on TACN in combination with heterocyclic amine donors providing a six-coordinate geometry should be able to form Cu2+ complexes of high stability/inertness, making the hexadentate ligands NOTI, NOTI-Me and NOTThia potential candidates for stable 64Cu chelation.
Ligand syntheses
Introduction of the five-membered heterocyclic substituents to the macrocycle TACN was accomplished by reductive amination as illustrated in Scheme 2. All chelators were fully characterized by 1H and 13C NMR spectroscopy as well as high resolution mass spectrometry. The corresponding data are available as ESI.‡
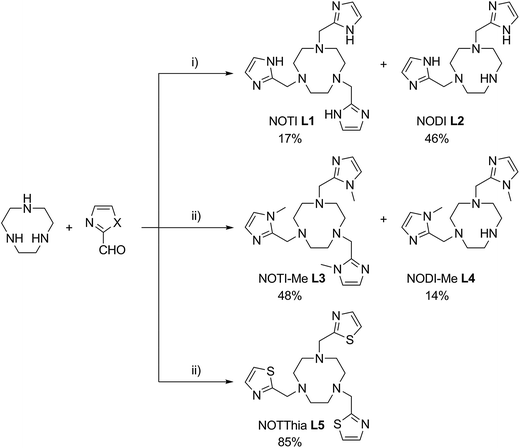 |
| Scheme 2 Synthetic route for chelators presented in this work. (i) NaBH4/H2O; (ii) NaBH(OAc)3/DCE. | |
The imidazole-derivatized chelators, NOTI L1 (1,4,7-tris((1H-imidazol-2-yl)methyl)-1,4,7-triazonane) and NODI L2 (1,4-bis((1H-imidazol-2-yl)methyl)-1,4,7-triazonane) were prepared by reacting 1 equiv. TACN with 6 equiv. imidazole-2-carboxaldehyde in water. After formation of the corresponding imine, indicated by formation of an orange solution, sodium borohydride was added to the reaction mixture. After workup, a product mixture with a L1
:
L2 ratio of 1
:
3 was obtained that could not be separated by regular silica gel column chromatography; however, separation could be accomplished by semi-preparative RP-HPLC, providing ligands L1 and L2 in 17% and 46% yield, respectively. The use of either sodium borohydride/TFE (2,2,2-trifluoroethanol)59 or sodium triacetoxyborohydride/DCE (1,2-dichloroethane)60 as reducing systems gave significantly lower yields due to the low solubility of imidazole-2-carboxaldehyde in these solvents.
The synthesis of the ligand NOTI-Me L3 has been reported previously by Di Varia et al. using 1-methyl-2-chloromethylimidazole hydrochloride for the introduction of the methyl-imidazole residues.61 Here, we report the synthesis of L3 and L4 by reductive amination. Reaction of 1 equiv. TACN with 6 equiv. 4-Methyl-1H-imidazole-2-carboxaldehyde in the presence of sodium triacetoxyborohydride in DCE resulted in a product mixture of the di- and trisubstituted compounds NOTI-Me L3 (1,4,7-tris((1-methyl-1H-imidazol-2-yl)methyl)-1,4,7-triazonane) and NODI-Me L4 (1,4-bis((1H-imidazol-2-yl)methyl)-1,4,7-triazonane). Both compounds NOTI-Me and NODI-Me were separated by semi-preparative RP-HPLC to give L3 and L4 in 48% and 14% yield, respectively.
Reaction of 1 equiv. TACN with 3 equiv. thiazole-2-carboxaldehyde in the presence of NaBH(OAc)3 in DCE provided exclusively the trisubstituted derivative NOTThia L5. After workup, compound L5 was obtained in analytical purity in 85% yield. A small aliquot was additionally purified by semi-preparative RP-HPLC for further experiments.
Structural characterization in solution
Complex formation of L1, L3 and L5 was investigated by electronic absorption (UV/vis) titration experiments in PBS. For all complexes, plots of the absorbance at λmax vs. [Cu2+]/[ligand] gave a sharp inflection point at ∼1.0 for each chelator, confirming exclusive formation of mononuclear complexes at physiological pH. The corresponding titration graphs are given as ESI.‡ Further structural insights could be gained from the absorption spectra of Cu2+ complexes (provided as ESI‡). The corresponding absorption maxima λmax are given in Table 1. The λmax of Cu(NOTI) (Cu-1) of 580 nm at pH 7.4 is comparable to the 585 nm reported for the structurally similar, five-coordinate NOTI-Me complex in acetonitrile by Stoppioni and co-workers.62 Furthermore, the λmax is in accordance with other copper pentaamine complexes in water, confirming formation of a five-coordinate NOTI L1 complex at physiological pH.63 In contrast, all six nitrogen donors of NOTI-Me L3 are bound to Cu2+ at neutral pH. The corresponding λmax of 700 nm is comparable to the 720 nm reported for the six-coordinate Cu(NOTI-Me) (Cu-3) complex62 and to other six-coordinate copper hexaamine complexes.64,65 The ligand NOTThia L5 also coordinates the Cu2+ cation via six nitrogen donors at neutral pH with a λmax = 650 nm. In 5 M HCl, the complex Cu-4 exhibits its maximum at λmax = 575 nm, suggesting formation of a five-coordinate complex under highly acidic conditions.
X-ray crystallography
Further structural information was obtained by X-ray analysis of corresponding copper complexes. Single crystals of complexes were grown by combination of either water or water
:
acetonitrile solutions of the corresponding metal salt and the chelator followed by slow evaporation of the solvents at room temperature. The crystallographic refinement data are given in Table 2. Selected bond distances and angles are summarized in Tables 3 and 4. For all complexes, the Cu–N distances fell into expected ranges and are comparable to the distances reported for Cu(TACN) and Cu(NOTA).66,67 Depending on the formation of a five- or six-coordinate complex, either one or two Cu–N bonds are elongated compared to the remaining Cu–N contacts due to the d9 electronic configuration, resulting in Jahn-Teller distortion (Tables 3 and 4). For all complexes, the corresponding N–Cu–N angles vary considerably and, hence, the coordination spheres around the Cu2+ center are highly distorted.
Table 2 Crystallographic data and structure refinement for copper complexes of L1, L3 and L5
Complex |
Cu-1 |
Cu-2 |
Cu-3 |
Cu-4 |
Cu-5 |
CCDC entry no. |
1043399 |
1043400 |
1043398 |
1043401 |
1043403 |
Empirical formula |
C18H30Cl3CuN9O13.3 |
C46H77Cl5Cu2F6N18O28 |
C21H33B2CuF8N9 |
C18H24Cl2CuN6O8S3 |
C18H24CuN8O6S3 |
Formula weight |
755.27 g mol−1 |
1748.58 g mol−1 |
684.72 g mol−1 |
683.05 g mol−1 |
608.17 g mol−1 |
Temperature |
100.0(2) K |
100.0(2) K |
100.0(2) K |
100.0(2) K |
100.0(2) K |
Wavelength |
0.71073 Å |
0.71073 Å |
0.71073 Å |
0.71073 Å |
0.71073 Å |
Crystal habitus |
Clear light blue plate |
Clear light blue plank |
Clear light blue plate |
Clear light green block |
Clear light green plate |
Crystal dimensions |
0.20 × 0.20 × 0.05 mm |
0.10 × 0.10 × 0.05 mm |
0.21 × 0.11 × 0.07 mm |
0.20 × 0.20 × 0.15 mm |
0.10 × 0.10 × 0.05 mm |
Crystal system |
Orthorhombic |
Triclinic |
Hexagonal |
Monoclinic |
Monoclinic |
Space group |
Pbca |
P![[1 with combining macron]](https://www.rsc.org/images/entities/char_0031_0304.gif) |
P63 |
P21/c |
P21/c |
Unit cell dimensions |
a = 11.8317(3) Å |
a = 10.7732(2) Å |
a = 11.0381(2) Å |
a = 17.6700(12) Å |
a = 8.3376(3) Å |
b = 13.3857(3) Å |
b = 18.8231(4) Å |
b = 11.0381(2) Å |
b = 15.8947(11) Å |
b = 16.3381(6) Å |
c = 36.8172(9) Å |
c = 19.6569(5) Å |
c = 13.1020(3) Å |
c = 23.4201(12) Å |
c = 17.2140(6) Å |
|
α = 62.6910(10)° |
|
β = 129.627(3)° |
β = 95.926(2)° |
|
β = 75.1780(10)° |
|
|
|
γ = 78.2020(10)° |
Volume |
5831.0(2) Å3 |
3406.4(1) Å3 |
1382.5(1) Å3 |
5066.3(6) Å3 |
2332.4(2) Å3 |
Z |
8 |
2 |
2 |
8 |
4 |
Density (calculated) |
1.721 g cm−3 |
1.705 g cm−3 |
1.558 g cm−3 |
1.791 g cm−3 |
1.732 g cm−3 |
Absorption coefficient |
1.104 mm−1 |
0.933 mm−1 |
0.874 mm−1 |
1.379 mm−1 |
1.260 mm−1 |
Reflections collected |
61 849 |
67 729 |
12 448 |
51 490 |
23 641 |
Independent reflections |
7053 [R(int) = 0.0378] |
15 562 [Rint = 0.0362] |
1588 [R(int) = 0.0309] |
11 650 [R(int) = 0.0193] |
5351 [R(int) = 0.0362] |
Data/restraints/parameters |
7053/680/562 |
15 562/1240/1017 |
1588/196/151 |
11 650/139/713 |
5351/0/325 |
Data completeness |
0.996 |
0.990 |
0.990 |
0.995 |
0.998 |
Goodness-of-fit on F2 |
1.139 |
1.035 |
1.085 |
1.025 |
1.034 |
Final R indices [I > 2sigma(I)] |
R1 = 0.0516, wR2 = 0.1145 |
R1 = 0.0535, wR2 = 0.1472 |
R1 = 0.0618, wR2 = 0.1686 |
R1 = 0.0242, wR2 = 0.0653 |
R1 = 0.0291, wR2 = 0.0647 |
R indices (all data) |
R1 = 0.0678, wR2 = 0.1211 |
R1 = 0.0688, wR2 = 0.1599 |
R1 = 0.0651, wR2 = 0.1713 |
R1 = 0.0297, wR2 = 0.0696 |
R1 = 0.0382, wR2 = 0.0684 |
Table 3 Selected bond lengths and metal-to-plane distances of presented metal complexes given in [Å]
Complex |
Cu-1 |
Cu-2 |
Cu(TACN)66 |
Cu–N distances in [Å] |
Cu1–N1 2.030(3) |
Cu1–N1 2.202(3) |
Cu1–N3 2.0458(3) |
Cu1–N2 2.070(3) |
Cu1–N2 2.032(3) |
Cu1–N1 2.0471(3) |
Cu1–N3 2.244(3) |
Cu1–N3 2.061(3) |
Cu1–N2 2.2309(4) |
Cu1–N4 1.973(3) |
Cu1–N4 1.943(3) |
|
Cu1–N6 1.940(3) |
Cu1–N6 1.970(3) |
|
|
Cu2–O27 2.725(1) |
|
|
Cu2–N10 2.265(3) |
|
|
Cu2–N11 2.043(3) |
|
|
Cu2–N12 2.042(3) |
|
|
Cu2–N13 1.951(3) |
|
|
Cu2–N17 1.970(3) |
|
N–Cu–N angles in [°] |
N4–Cu1–N6 104.2(1) |
N4 Cu1–N6 103.0(1) |
N3–Cu1–N1 82.336(5) |
N6–Cu1–N1 167.7(1) |
N4 Cu1–N3 84.1(1) |
N3–Cu1–N2 82.327(4) |
N6–Cu1–N2 84.3(1) |
N4 Cu1–N1 101.9(1) |
N1–Cu1–N2 83.464(4) |
N6–Cu1–N3 101.4(1) |
N6 Cu1–N1 115.7(1) |
|
N4–Cu1–N1 83.2(1) |
N3 Cu1–N1 85.0(1) |
|
N4–Cu1–N2 158.3(1) |
N13–Cu2–N17 106.8(1) |
|
N4–Cu1–N3 112.9(1) |
N13–Cu2–N10 99.1(1) |
|
N1–Cu1–N2 85.5(1) |
N17–Cu2–N11 83.0(1) |
|
N1–Cu1–N3 84.3(1) |
N17–Cu2–N10 106.5(1) |
|
N2–Cu1–N3 84.2(1) |
N11–Cu2–N10 83.0(1) |
|
Cu-planeTACN distance in [Å] |
1.325(1) |
1.309(1) |
1.359(1) |
1.324(1) |
Table 4 Selected bond lengths and metal-to-plane distances of presented metal complexes given in [Å]
Complex |
Cu-3 |
Cu-4 |
Cu-5 |
Cu(NOTA)67 |
Cu–N distances in [Å] |
Cu1–N3 2.049(8) |
Cu1–N1 2.289(2) |
Cu1–N1 2.122(2) |
Cu1–O1 1.9782(7) |
Cu1–N1B 2.191(8) |
Cu1–N2 2.157(2) |
Cu1–N2 2.343(2) |
Cu1–N3 2.0385(7) |
|
Cu1–N3 2.046(2) |
Cu1–N3 2.086(2) |
Cu1–N1 2.1156(3) |
|
Cu1–N4 2.037(2) |
Cu1–N4 1.995(2) |
Cu1–O3 2.1411(4) |
|
Cu1–N5 2.296(2) |
Cu1–N5 2.227(2) |
Cu1–N2 2.1966(3) |
|
Cu1–N6 2.004(2) |
Cu1–N6 2.131(2) |
Cu1–O2 2.2052(3) |
|
Cu2–N12 2.025(2) |
|
|
|
Cu2–N10 2.034(2) |
|
|
|
Cu2–N7 2.043(2) |
|
|
|
Cu2–N8 2.206(2) |
|
|
|
Cu2–N9 2.274(2) |
|
|
|
Cu2–N11 2.326(2) |
|
|
N–Cu–N angles in [°] |
N1B–Cu1–N3 78.4(1) |
N6–Cu1–N4 93.2(6) |
N4–Cu1–N3 156.746(7) |
O1–Cu1–N3 159.063(15) |
N1B–Cu1–N1B 80.0(1) |
N6–Cu1–N3 82.4(6) |
N4–Cu1–N6 93.799(7) |
O1–Cu1–N1 82.196(11) |
N1B–Cu1–N3 153.5(1) |
N6–Cu1–N2 155.2(6) |
N4–Cu1–N5 89.548(7) |
O1–Cu1–O3 91.047(16) |
N1B–Cu1–N3 110.8(1) |
N6–Cu1–N1 119.8(6) |
N4–Cu1–N2 115.422(7) |
O1–Cu1–N2 111.860(11) |
N3–Cu1–N3 95.3(1) |
N4–Cu1–N3 153.8(6) |
N3–Cu1–N1 84.184(7) |
O1–Cu1–O2 85.330(14) |
|
N4–Cu1–N1 77.2(6) |
N3–Cu1–N6 80.286(7) |
N3–Cu1–N1 85.467(15) |
|
N3–Cu1–N1 82.7(6) |
N3–Cu1–N5 112.756(6) |
N3–Cu1–O3 79.451(11) |
|
N12–Cu2–N10 95.0(6) |
N3–Cu1–N2 78.987(6) |
N3–Cu1–N2 82.824(14) |
|
N12–Cu2–N7 155.3(6) |
N1–Cu1–N6 126.019(7) |
N3–Cu1–O2 113.443(12) |
|
N12–Cu2–N11 89.4(6) |
N1–Cu1–N5 143.167(7) |
N1–Cu1–O3 116.649(11) |
|
N10–Cu2–N7 82.3(6) |
N1–Cu1–N2 78.733(6) |
N1–Cu1–N2 81.703(12) |
|
N10–Cu2–N9 121.4(6) |
N6–Cu1–N5 89.947(6) |
N1–Cu1–O2 148.427(13) |
|
N10–Cu2–N11 93.0(6) |
N6–Cu1–N2 145.510(6) |
O3–Cu1–N2 153.058(13) |
|
N7–Cu2–N11 115.3(6) |
N5–Cu1–N2 73.279(6) |
O3–Cu1–O2 92.389(14) |
Cu-planeTACN distance in [Å] |
1.469(1) |
1.406(1) |
1.439(1) |
1.353(1) |
1.430(1) |
Cu-planeheterocycle distance in [Å] |
1.069(1) |
1.178(1) |
1.194(1) |
1.222(1) |
1.165(1) |
Slow evaporation of an acidic solution (pH < 2) of Cu(ClO4)2·6H2O and NOTI·6TFA L1 provided single crystals of complex Cu-1 that crystallized in the space group Pbca. A representative ORTEP plot is given in Fig. 1. Attempts to prepare single crystals of a six-coordinate NOTI complex at neutral and basic conditions were not successful. The structure of Cu-1 contains the complex cation [Cu(NOTI)H]3+, perchlorate anions, and molecules of water of crystallization. The imidazolium group, two perchlorate anions and one water site are disordered with an 70
:
30 occupancy. The Cu2+ cation is coordinated by three nitrogen atoms of the TACN macrocycle and two nitrogen atoms of the imidazole substituents. One imidazole is protonated and, thus, not coordinated to the metal center. If N3 is considered to be in the apical position of a square pyramid the N–Cu–N angle difference δ between the donor atom pairs N1/N6 and N2/N4 is an indication of the degree of distortion. For an ideal square pyramidal geometry δ would be 0° (cf. 60° for an ideal trigonal bipyramid). With δ = 9.2°, the [Cu[NOTI]H3+] complex exhibits a distorted square pyramidal coordination geometry.
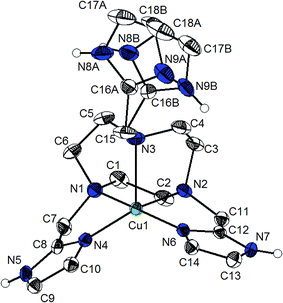 |
| Fig. 1 An ORTEP representation of the cation found in [Cu(NOTI)H](ClO4)3 Cu-1 (50% thermal ellipsoid probability). The uncoordinated imidazole group is disordered, both occupation states are shown in the figure. Carbon bound hydrogen atoms are omitted for clarity. | |
For NOTI-Me L3, two different single crystals were obtained depending on the pH of the crystallization solution. Complex Cu-2 crystallized in the space group P
and was obtained from acidic medium (pH < 2) using the TFA salt of L3. The unit cell contains two [Cu(NOTI-Me)H]3+ complex units, in which one 1-methylimidazole substituent is protonated on the nitrogen atom in each complex, a protonated trifluoroacetic acid molecule, a deprotonated trifluoroacetic acid molecule, five perchlorate counter ions, and water of crystallization. One of the two [Cu(NOTI-Me)H]3+ complex units is five-coordinate (Fig. 2a). The N–Cu–N angle difference between N3/N6 and N2/N4 of δ = 11.8° is indicative for a distorted square pyramidal coordination geometry. The second [Cu(NOTI-Me)H]3+ complex exhibits a 5 + 1 coordination in which the Cu2+ cation is coordinated by five nitrogen atoms of the ligand NOTI-Me and a water molecule (Fig. 2b). Under basic conditions (pH > 12), the ligand NOTI-Me L3 forms a six-coordinate CuN3N′3 coordination geometry in which all nitrogen donor atoms of the ligand are bound to the metal center. An ORTEP plot of complex Cu-3 (space group P63) is shown in Fig. 3. The TACN backbone is disordered about 3-fold axis (60
:
40 ratio). One tetrafluoroborate anion is disordered about the 3-fold axis and another one is disordered about the 63 axis (70
:
30 ratio). Depending on the disorder, the ethylene groups of the TACN macrocycle form either the λλλ or the δδδ isomer (60
:
40 ratio). A twist angle of ϕ = 29.5° for the [Cu(NOTI-Me)]2+ complex indicates a distorted pseudo-prismatic geometry of the CuN3N′3 polyhedron. The coordination sphere with a twist angle of ϕ = 26.6° is comparable to that of the Cu(NOTA) complex.67
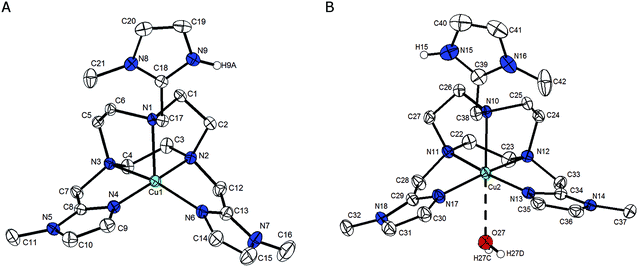 |
| Fig. 2 Both complex units of [Cu(NOTI-Me)H]2(ClO4)5·2TFA·4H2O Cu-2 in the unit cell (50% thermal ellipsoid probability). Carbon bound hydrogen atoms are omitted for clarity. | |
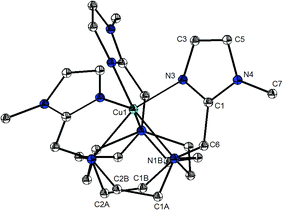 |
| Fig. 3 An ORTEP representation of the cation found in [Cu(NOTI-Me)](BF4)2 Cu-3 (50% thermal ellipsoid probability). The triazacyclononane backbone is disordered about 3-fold axis (60 : 40 ratio). Both occupation states are shown in the figure. One tetrafluoroborate anion is disordered about 3-fold axis (disorder not modeled), the other is disordered about the 63 axis (70 : 30 ratio). Carbon bound hydrogen atoms are omitted for clarity. | |
For NOTThia L5, single crystals of two structurally closely related complexes Cu-4 and Cu-5 were obtained. Both complexes crystallized in the space group P21/c. The unit cell of Cu-4 contains two [Cu(NOTThia)]2+ molecules and four perchlorate counter ions. In both complex units of Cu-4, the metal center possesses a six-coordinate coordination geometry with three nitrogen donors of the TACN macrocycle and three nitrogen donors of the thiazoles bound to the Cu2+ cations. A representative view of one of the complex units is given in Fig. 4. Corresponding data of the closely related structure of [Cu(NOTThia)](NO3)2 Cu-5 are provided as ESI.‡ With a mean twist angle of ϕ = 25.3°, the CuN3N′3 polyhedron of the NOTThia complexes can best be described as a distorted pseudo-prismatic geometry.
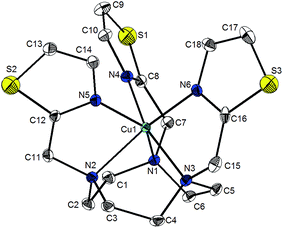 |
| Fig. 4 An ORTEP representation of the cation found in [Cu(NOTThia)](ClO4)2 Cu-4 (50% thermal ellipsoid probability). Carbon bound hydrogen atoms are omitted for clarity. | |
The excellent complexation properties of the presented ligands (vide infra) most likely result from the high affinity of the Cu2+ center to the azaheterocyclic amine donors. An indicator for this affinity is the corresponding metal-to-plane distance (Tables 3 and 4). In six-coordinate complexes Cu-3, Cu-4 and Cu-5, the Cu-planeTACN distances are significantly longer than those for Cu(NOTA) and Cu(TACN). In return, the Cu-planeazaheterocycle distances are significantly shorter than the Cu-planeacetate distance for Cu(NOTA). In addition, small differences could be noted between the five- and six-coordinate complexes that also point towards the high affinity of Cu2+ to azaheterocyclic nitrogen donors. If all residues coordinate to Cu2+ the Cu-planeazaheterocycle distances are significantly shorter than the corresponding Cu-planeTACN distances and, consequently, the metal center is associated more closely with the azaheterocyclic substituents.
64Cu labeling experiments
One important aspect for a chelating agent in view of radiopharmaceutical applications is the conditions under which quantitative labeling can be achieved. In this respect, the chelator concentration, temperature, pH and incubation time are the most important parameters influencing the RCC. Initial experiments were performed in ammonium acetate at pH 8.0 to determine the lowest concentration necessary for quantitative RCC for the ligands presented. Ammonium acetate was chosen for initial tests because 64Cu-complexation rates are known to be fast in acetate containing solutions.47,68
Over the investigated pH range, the chelators NOTI L1, NOTI-Me L3 and NOTThia L5 radiolabeled efficiently at ambient temperature in less than one minute using a chelator concentration of 1 μM (Fig. 5). 64Cu complexes of L1, L3 and L5 (64Cu-1, 64Cu-3, 64Cu-4) were obtained with RCC >95% with specific activities of up to 120–180 MBq nmol−1. RCC generally decreased by 20–30% at lower chelator concentrations (0.1 μM). On the contrary, both reference compounds NOTA and DOTA gave significantly lower RCC under identical conditions (Fig. 5). Altogether, the results show that the labeling kinetics of novel ligands are faster than those of the controls NOTA and DOTA, which is most likely a consequence of the high affinity of Cu2+ for azaheterocyclic nitrogen donors. Moreover, novel chelators exhibited fast labeling characteristics at physiological pH under mild conditions, making this class of chelators a promising candidate for radiolabeling of sensitive biomolecules such as antibodies.
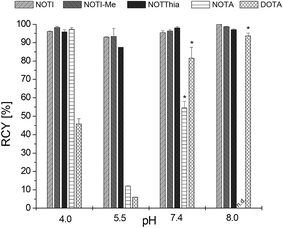 |
| Fig. 5 RCC of NOTI L1, NOTI-Me L3, NOTThia L5, NOTA and DOTA in 64Cu labeling experiments; c(L) = 1 μM, r.t., 1 min; all buffers c = 0.1 M; * represents c(L) = 5 μM, 95 °C, 30 min. | |
Stability measurements
The most important aspect of a chelating agent as part of a radiopharmaceutical is the kinetic inertness of the corresponding metal complex to avoid premature loss of the label and thereby reduce accumulation of activity in non-target tissues. In recent years, different approaches were used to assess the kinetic inertness of 64Cu complexes in order to predict stability in vivo. Methods include ligand challenge and copper exchange experiments as well as acid decomplexation and serum stability studies.57,58 In initial experiments, stability of the 64Cu complexes was measured in human serum (Table 1). However, no significant differences in stability were noted; all 64Cu complexes including the controls remained intact over the 24 h time period of the experiment (Table 1). The corresponding protein associated activity was low for all complexes with 11.6 ± 0.5%, 8.3 ± 0.3%, 9.1 ± 0.2%, 5.4 ± 0.5%, 5.0 ± 0.3% for 64Cu(NOTI), 64Cu(NOTI-Me), 64Cu(NOTThia), 64Cu(DOTA) and 64Cu(NOTA), respectively. A graphical representation is given as ESI.‡ Kinetic inertness of corresponding 64Cu complexes was assessed by using methods described by Maheshwari et al. The 64Cu complexes were incubated with an 5-fold molar excess of non-radioactive Cu2+.56,64 Cu complexes of TACN derived ligands NOTI L1, NOTI-Me L3, NOTThia L5 (64Cu-1, 64Cu-3 and 64Cu-4) and NOTA showed only minimal exchange (<2%) after 24 h incubation time, demonstrating that this type of chelators is kinetically inert towards metal exchange under these experimental conditions (Table 1). This is consistent with the results of Maheshwari et al. who found that NOTA is kinetically more inert than TETA and DOTA.56 From the results, it can further be seen that the macrocyclic ring size plays an important role in terms of the kinetic inertness. While all TACN based ligands were essentially kinetically inert, the corresponding DOTA complex showed substantial loss of 64Cu.
Since no significant differences were noted between the TACN based chelators in the copper exchange experiments, more aggressive acid decomplexation studies were performed to assess kinetic inertness.57,58 Corresponding results are summarized in Table 1. The Cu(DOTA) complex dissociated rapidly as did the NOTA complex – both decomposed upon addition to 5 M hydrochloric acid. Complexes of NOTI and NOTI-Me (64Cu-1 and 64Cu-3) were slightly more inert with half-lives of ∼0.5 min at room temperature. Interestingly, the NOTThia complex 64Cu-4 showed high kinetic inertness with a half-life of ∼55 h at 95 °C. One possible explanation for the kinetic inertness of 64Cu-4 might be the higher acidity of the thiazole residues compared to the imidazole and acetic acid substituents for NOTI/NOTI-Me and NOTA, respectively. Another factor that might contribute is the overall charge of the complex. While the NOTA complex has a negative overall charge, complexes Cu-1, Cu-3 and Cu-4 are positively charged, making them potentially less prone to proton-assisted demetallation. The influence of the mechanism of decomplexation on the kinetic inertness is exemplified by the Cu(DOTA) complex, which has also a negative overall charge, but the mechanism of decomplexation seems to be different to NOTA as the loss of 64Cu is inversely dependent on the pH.56
Small animal PET imaging
The very fast labeling kinetics over a wide pH range as well as the high kinetic inertness/stability of corresponding copper complexes in vitro prompted us to assess the biodistribution of the corresponding 64Cu complexes by small animal PET imaging in mice. Coronal PET maximum intensity projections of the corresponding 64Cu complexes and 64CuCl2 at 1, 5, 15, 30 and 45 min post-injection (p.i.) are given in Fig. 6. Corresponding time activity curves for the liver and the kidney uptake are provided as ESI.‡ All 64Cu complexes including NOTA and DOTA exhibited fast blood clearance with blood levels of <5% injected activity per gram (% IA per g) after 5 min p.i. and <2% IA per g after 15 min p.i. All complexes were excreted via the renal pathway in contrast to uncomplexed 64Cu2+ (64CuCl2 in saline) that showed high uptake and retention in the liver. The positively charged, uncomplexed 64Cu2+ is known to accumulate and to be retained in the liver and the kidneys as can been seen in Fig. 6.69 The biodistribution of 64Cu-1, 64Cu-3 and 64Cu-4 differs significantly from 64Cu2+ as well as the corresponding NOTA and DOTA complexes. The DOTA and NOTA complexes showed the typical clearance of an unretained compound with an initial high uptake in the kidneys and moderate uptake in the liver that both gradually decreased during the course of the experiment. To the contrary, uptake of 64Cu-1, 64Cu-3 and 64Cu-4 in the kidneys reached a plateau after 5 min p.i. and the activity remained essentially constant over the entire imaging period (NOTI ∼40% IA per g, NOTI-Me ∼20% IA per g and ∼20% IA per g at 45 min p.i.), indicating retention of the complexes. A similar finding was obtained for the liver uptake. While both NOTA and DOTA were cleared gradually from the liver, 64Cu-1, 64Cu-3 and 64Cu-4 showed an increase in uptake within the initial 5 min and persistent uptake until the end of the experiment (64Cu-1 ∼11% IA per g, 64Cu-3 ∼11% IA per g and 64Cu-4 ∼17% IA per g at 45 min p.i.). No correlation between the uptake in the kidneys and the liver, and the lipophilicity of corresponding 64Cu complexes (see log
D values in Table 1) was noted. An increase in 64Cu uptake in the liver and kidneys is often used as evidence for instability of a 64Cu chelate caused by superoxide dismutase (SOD1) dependent transchelation.70 SOD1 is a homodimeric enzyme that is mostly abundant in cells of the liver and the kidneys as well as erythrocytes.70 Several stability studies of 64Cu-labeled BFC biomolecule conjugates have pointed to the dissociation of 64Cu in the presence of SOD1.30,71 The differences in kidney and liver uptake between 64Cu(NOTA) and 64Cu(DOTA), and 64Cu-1, 64Cu-3 and 64Cu-4 may also be attributed to different physical properties of the complexes, namely the different overall charge. The DOTA and NOTA complex are negatively charged, whereas the complexes of NOTI L1, NOTI-Me L3, and NOTThia L5 have a positive overall charge. Cationic species are known to be retained in the kidneys and the liver.72–74 The results of the PET study do not allow to distinguish whether the uptake of 64Cu-1, 64Cu-3 and 64Cu-4 in both organs occurs due to transchelation in vivo or due to the positive overall charge. Further studies of this novel type of chelators in combination with biologically active targeting vectors in which the biodistribution profile will then be dominated by the properties of the attached biovector (e.g. antibody, peptide, etc.) are necessary in order to elucidate the reason for the high uptake and retention of 64Cu-1, 64Cu-3 and 64Cu-4 in the kidneys and the liver.
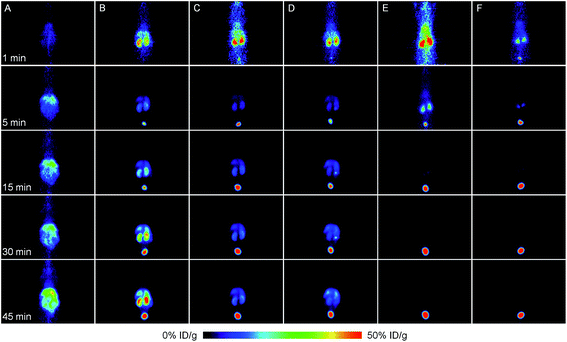 |
| Fig. 6 PET images in balb/c mice of (A) 64CuCl2 and 64Cu complexes of (B) NOTI L1, (C) NOTI-Me L3, (D) NOTThia L5, (E) NOTA and (F) DOTA at 1, 5, 15, 30 and 45 min post-injection. | |
Conclusion
A series of novel hexadentate chelators with pendant heterocyclic substituents derived from the macrocycle TACN were successfully prepared in a single reaction step in good yields. The set of novel chelators exhibited excellent labeling properties with 64Cu under mild conditions over a wide pH range, yielding high specific activities. These ligands label readily at physiological pH, a feature that is of particular interest for radiolabeling pH sensitive biomolecules such as antibodies. Corresponding 64Cu complexes proved to be of high kinetic inertness in vitro. Results for NOTI, which only forms five-coordinate complexes, suggest that two azaheterocyclic substituents might be sufficient for radiopharmaceutical applications of this type of chelators. Initial biological testing by small animal PET imaging revealed retention of corresponding 64Cu complexes in the kidneys and, to a lesser extent, in the liver. The biodistribution of 64Cu complexes of presented ligands was significantly different to 64CuCl2 and 64Cu(DOTA), which is known to be prone to transchelation, suggesting retention due to their positive overall charge. However, further testing in vivo with biologically active targeting vectors is necessary to confirm sufficient stability in vivo. While thiazole-type ligands are promising candidates for the development of traditional bifunctional chelators due to their pronounced inertness, the NOTI/NOTI-Me system demonstrates the possibility for chemical modification at the nitrogen of the imidazole residues without perturbation of the complexation properties. In this respect, this system has the potential to be developed as a platform for multivalent/-meric radiopharmaceuticals. Altogether, the results of the present study, outlining the excellent labeling properties and the high kinetic inertness in vitro, warrant for the development of bifunctional derivatives of this class of ligands for evaluation in combination with biologically relevant targeting vectors in vivo.
Acknowledgements
The authors would like to thank the Chemistry Department of the Albert-Ludwigs-University Freiburg for its support, in particular Manfred Keller for NMR measurements, Christoph Warth for MS measurements and Nils Trapp, Boumahdi Benkmil, and Daniel Kratzert for X-ray analysis. Furthermore, the authors like to thank Alan Packard (Harvard Medical School/Children's Hospital Boston) for critically reviewing this manuscript and Chematech (Dijon, France) for kindly providing 1,4,7-triazacyclononane.
References
- E. W. Price and C. Orvig, Chem. Soc. Rev., 2014, 43, 260–290 RSC.
- I. Velikyan, Recent Results Cancer Res., 2013, 194, 101–131 CAS.
- J. D. Correia, A. Paulo, P. D. Raposinho and I. Santos, Dalton Trans., 2011, 40, 6144–6167 RSC.
- M. Bartholoma, J. Valliant, K. P. Maresca, J. Babich and J. Zubieta, Chem. Commun., 2009, 7, 493–512 RSC.
- D. Zwanziger and A. G. Beck-Sickinger, Curr. Pharm. Des., 2008, 14, 2385–2400 CrossRef CAS.
- J. S. Lewis and C. J. Anderson, Methods Mol. Biol., 2007, 386, 227–240 CAS.
- H. R. Maecke, M. Hofmann and U. Haberkorn, J. Nucl. Med., 2005, 46(Suppl 1), 172S–178S CAS.
- C. F. Ramogida and C. Orvig, Chem. Commun., 2013, 49, 4720–4739 RSC.
- C. Decristoforo, R. D. Pickett and A. Verbruggen, Eur. J. Nucl. Med. Mol. Imaging, 2012, 39(Suppl 1), S31–S40 CrossRef.
- C. Bolzati, D. Carta, N. Salvarese and F. Refosco, Anti-Cancer Agents Med. Chem., 2012, 12, 428–461 CrossRef CAS.
- G. Hao, A. N. Singh, O. K. Oz and X. Sun, Curr. Radiopharm., 2011, 4, 109–121 CrossRef CAS.
- M. Shokeen and T. J. Wadas, Med. Chem., 2011, 7, 413–429 CrossRef CAS.
- M. T. Ma and P. S. Donnelly, Curr. Top. Med. Chem., 2011, 11, 500–520 CrossRef CAS.
- T. J. Wadas, E. H. Wong, G. R. Weisman and C. J. Anderson, Curr. Pharm. Des., 2007, 13, 3–16 CrossRef CAS.
- P. J. Blower, J. S. Lewis and J. Zweit, Nucl. Med. Biol., 1996, 23, 957–980 CrossRef CAS.
- B. M. Zeglis and J. S. Lewis, Dalton Trans., 2011, 40, 6168–6195 RSC.
- Y. Yoshii, T. Furukawa, Y. Kiyono, R. Watanabe, T. Mori, H. Yoshii, T. Asai, H. Okazawa, M. J. Welch and Y. Fujibayashi, Nucl. Med. Biol., 2011, 38, 151–157 CrossRef CAS.
- D. Ma, F. Lu, T. Overstreet, D. E. Milenic and M. W. Brechbiel, Nucl. Med. Biol., 2002, 29, 91–105 CrossRef CAS.
- A. K. Boal and A. C. Rosenzweig, Chem. Rev., 2009, 109, 4760–4779 CrossRef CAS PubMed.
- T. J. Wadas, E. H. Wong, G. R. Weisman and C. J. Anderson, Chem. Rev., 2010, 110, 2858–2902 CrossRef CAS.
- M. Eigen, Pure Appl. Chem., 1963, 6, 97 CrossRef CAS.
- M. D. Bartholomä, Inorg. Chim. Acta, 2012, 389, 36–51 CrossRef.
- L. Wei, Y. Ye, T. J. Wadas, J. S. Lewis, M. J. Welch, S. Achilefu and C. J. Anderson, Nucl. Med. Biol., 2009, 36, 277–285 CrossRef CAS.
- H. Cai, J. Fissekis and P. S. Conti, Dalton Trans., 2009, 5395–5400 RSC.
- H. Cai, Z. Li, C. W. Huang, R. Park, A. H. Shahinian and P. S. Conti, Nucl. Med. Biol., 2010, 37, 57–65 CrossRef CAS PubMed.
- H. Cai, Z. Li, C. W. Huang, A. H. Shahinian, H. Wang, R. Park and P. S. Conti, Bioconjugate Chem., 2010, 21, 1417–1424 CrossRef CAS PubMed.
- N. Di Bartolo, A. M. Sargeson and S. V. Smith, Org. Biomol. Chem., 2006, 4, 3350–3357 CAS.
- N. M. Di Bartolo, A. M. Sargeson, T. M. Donlevy and S. V. Smith, J. Chem. Soc., Dalton Trans., 2001, 2303–2309 RSC.
- S. Liu, Z. Li, L. P. Yap, C. W. Huang, R. Park and P. S. Conti, Chemistry, 2011, 17, 10222–10225 CrossRef CAS.
- C. A. Boswell, X. Sun, W. Niu, G. R. Weisman, E. H. Wong, A. L. Rheingold and C. J. Anderson, J. Med. Chem., 2004, 47, 1465–1474 CrossRef CAS.
- J. C. Garrison, T. L. Rold, G. L. Sieckman, S. D. Figueroa, W. A. Volkert, S. S. Jurisson and T. J. Hoffman, J. Nucl. Med., 2007, 48, 1327–1337 CrossRef CAS PubMed.
- S. H. Hausner, D. L. Kukis, M. K. Gagnon, C. E. Stanecki, R. Ferdani, J. F. Marshall, C. J. Anderson and J. L. Sutcliffe, Mol. Imaging, 2009, 8, 111–121 CAS.
- J. E. Sprague, Y. Peng, A. L. Fiamengo, K. S. Woodin, E. A. Southwick, G. R. Weisman, E. H. Wong, J. A. Golen, A. L. Rheingold and C. J. Anderson, J. Med. Chem., 2007, 50, 2527–2535 CrossRef CAS.
- X. Sun, M. Wuest, G. R. Weisman, E. H. Wong, D. P. Reed, C. A. Boswell, R. Motekaitis, A. E. Martell, M. J. Welch and C. J. Anderson, J. Med. Chem., 2002, 45, 469–477 CrossRef CAS.
- T. J. Wadas and C. J. Anderson, Nat. Protoc., 2006, 1, 3062–3068 CrossRef CAS PubMed.
- S. Juran, M. Walther, H. Stephan, R. Bergmann, J. Steinbach, W. Kraus, F. Emmerling and P. Comba, Bioconjugate Chem., 2009, 20, 347–359 CrossRef CAS.
- H. Stephan, M. Walther, S. Fahnemann, P. Ceroni, J. K. Molloy, G. Bergamini, F. Heisig, C. E. Muller, W. Kraus and P. Comba, Chemistry, 2014, 20, 17011–17018 CrossRef CAS PubMed.
- P. Comba, S. Hunoldt, M. Morgen, J. Pietzsch, H. Stephan and H. Wadepohl, Inorg. Chem., 2013, 52, 8131–8143 CrossRef CAS.
- S. Ait-Mohand, P. Fournier, V. Dumulon-Perreault, G. E. Kiefer, P. Jurek, C. L. Ferreira, F. Benard and B. Guerin, Bioconjugate Chem., 2011, 22, 1729–1735 CrossRef CAS.
- R. A. Dumont, F. Deininger, R. Haubner, H. R. Maecke, W. A. Weber and M. Fani, J. Nucl. Med., 2011, 52, 1276–1284 CrossRef CAS.
- D. Liu, D. Overbey, L. D. Watkinson, C. J. Smith, S. Daibes-Figueroa, T. J. Hoffman, L. R. Forte, W. A. Volkert and M. F. Giblin, Bioconjugate Chem., 2010, 21, 1171–1176 CrossRef CAS.
- M. Fani, L. Del Pozzo, K. Abiraj, R. Mansi, M. L. Tamma, R. Cescato, B. Waser, W. A. Weber, J. C. Reubi and H. R. Maecke, J. Nucl. Med., 2011, 52, 1110–1118 CrossRef CAS.
- S. R. Lane, P. Nanda, T. L. Rold, G. L. Sieckman, S. D. Figueroa, T. J. Hoffman, S. S. Jurisson and C. J. Smith, Nucl. Med. Biol., 2010, 37, 751–761 CrossRef CAS.
- G. Wieser, R. Mansi, A. L. Grosu, W. Schultze-Seemann, R. A. Dumont-Walter, P. T. Meyer, H. R. Maecke, J. C. Reubi and W. A. Weber, Theranostics, 2014, 4, 412–419 CrossRef CAS.
- Z. Cai and C. J. Anderson, J. Labelled Compd. Radiopharm., 2014, 57, 224–230 CrossRef CAS.
- P. Chaudhuri and K. Wieghardt, Prog. Inorg. Chem., 1987, 35, 329–436 CrossRef CAS.
- G. Gasser, L. Tjioe, B. Graham, M. J. Belousoff, S. Juran, M. Walther, J. U. Kunstler, R. Bergmann, H. Stephan and L. Spiccia, Bioconjugate Chem., 2008, 19, 719–730 CrossRef CAS.
- M. Roger, L. M. Lima, M. Frindel, C. Platas-Iglesias, J. F. Gestin, R. Delgado, V. Patinec and R. Tripier, Inorg. Chem., 2013, 52, 5246–5259 CrossRef CAS.
- R. Bergmann, A. Ruffani, B. Graham, L. Spiccia, J. Steinbach, J. Pietzsch and H. Stephan, Eur. J. Med. Chem., 2013, 70, 434–446 CrossRef CAS PubMed.
- Q.-X. Li, W. Zhang, Q.-H. Luo, Y.-Z. Li and Z.-l. Wang, Transition Met. Chem., 2003, 28, 682–686 CrossRef CAS.
- A. E. Martell, R. M. Smith and R. J. Motekaitis, NIST database, 46, vol. 8 Search PubMed.
- D. W. McCarthy, R. E. Shefer, R. E. Klinkowstein, L. A. Bass, W. H. Margeneau, C. S. Cutler, C. J. Anderson and M. J. Welch, Nucl. Med. Biol., 1997, 24, 35–43 CrossRef CAS PubMed.
- G. Sheldrick, Acta Crystallogr., Sect. A: Found. Crystallogr., 2008, 64, 112–122 CrossRef CAS PubMed.
- O. V. Dolomanov, L. J. Bourhis, R. J. Gildea, J. A. K. Howard and H. Puschmann, J. Appl. Crystallogr., 2009, 42, 339–341 CrossRef CAS.
- G. Sheldrick, SADABS - A Program for Empirical Absorption Correction of Area Detector Data, 1996 Search PubMed.
- V. Maheshwari, J. L. J. Dearling, S. T. Treves and A. B. Packard, Inorg. Chim. Acta, 2012, 393, 318–323 CrossRef CAS.
- C. J. Anderson, T. J. Wadas, E. H. Wong and G. R. Weisman, Eur. J. Nucl. Med. Mol. Imaging, 2008, 52, 185–192 CAS.
- K. S. Woodin, K. J. Heroux, C. A. Boswell, E. H. Wong, G. R. Weisman, W. Niu, S. A. Tomellini, C. J. Anderson, L. N. Zakharov and A. L. Rheingold, Eur. J. Inorg. Chem., 2005, 2005, 4829–4833 CrossRef.
- M. Tajbakhsh, R. Hosseinzadeh, H. Alinezhad, S. Ghahari, A. Heydari and S. Khaksar, Synthesis, 2011, 2011, 490–496 CrossRef.
- A. F. Abdel-Magid, K. G. Carson, B. D. Harris, C. A. Maryanoff and R. D. Shah, J. Org. Chem., 1996, 61, 3849–3862 CrossRef CAS PubMed.
- M. Di Vaira, F. Mani and P. Stoppioni, J. Chem. Soc., Chem. Commun., 1989, 126–127 RSC.
- G. de Martino Norante, M. Di Vaira, F. Mani, S. Mazzi and P. Stoppioni, J. Chem. Soc., Dalton Trans., 1992, 361–365 RSC.
- M. Kodama and E. Kimura, J. Chem. Soc., Dalton Trans., 1978, 104–110 RSC.
- P. V. Bernhardt and E. J. Hayes, J. Chem. Soc., Dalton Trans., 1998, 1037–1042 RSC.
- S. L. Grassino and D. N. Hume, J. Inorg. Nucl. Chem., 1970, 32, 3112–3113 CrossRef CAS.
- R. D. Bereman, M. R. Churchill, P. M. Schaber and M. E. Winkler, Inorg. Chem., 1979, 18, 3122–3125 CrossRef CAS.
- K. Wieghardt, U. Bossek, P. Chaudhuri, W. Herrmann, B. C. Menke and J. Weiss, Inorg. Chem., 1982, 21, 4308–4314 CrossRef CAS.
- J. R. Morphy, D. Parker, R. Kataky, M. A. W. Eaton, A. T. Millican, R. Alexander, A. Harrison and C. Walker, J. Chem. Soc., Perkin Trans. 2, 1990, 573–585 RSC.
- M. Shokeen and C. J. Anderson, Acc. Chem. Res., 2009, 42, 832–841 CrossRef CAS PubMed.
- K. Zarschler, M. Kubeil and H. Stephan, RSC Adv., 2014, 4, 10157–10164 RSC.
- L. A. Bass, M. Wang, M. J. Welch and C. J. Anderson, Bioconjugate Chem., 2000, 11, 527–532 CrossRef CAS PubMed.
- J. E. Sprague, Y. Peng, A. L. Fiamengo, K. S. Woodin, E. A. Southwick, G. R. Weisman, E. H. Wong, J. A. Golen, A. L. Rheingold and C. J. Anderson, J. Med. Chem., 2007, 50, 2527–2535 CrossRef CAS PubMed.
- J. L. Dearling, B. Paterson, P. Dunning, E. Snay, S. T. Treves and S. Voss, Nucl. Med., 2013, 54, 1090 Search PubMed.
- J. L. Dearling, B. M. Paterson, V. Akurathi, S. Betanzos-Lara, S. T. Treves, S. D. Voss, J. M. White, J. S. Huston, S. V. Smith, P. S. Donnelly and A. B. Packard, Bioconjugate Chem., 2015, 26, 707–717 CrossRef CAS PubMed.
Footnotes |
† Dedicated to Professor Kaspar Hegetschweiler on the occasion of his 60th birthday. |
‡ Electronic supplementary information (ESI) available: NMR and MS data of chelators, MS and HPLC data of metal complexes, electronic spectra of Cu nat. complexes, UV/vis titration data, crystallographic data, auxiliary information on X-ray structures. CCDC 1043398–1043401 and 1043403. For ESI and crystallographic data in CIF or other electronic format see DOI: 10.1039/c5ra21131j |
|
This journal is © The Royal Society of Chemistry 2016 |