Aluminium ion-promoted radical-scavenging reaction of methylated hydroquinone derivatives
Received
11th July 2016
, Accepted 29th July 2016
First published on 29th July 2016
Abstract
The effect of the aluminium ion (Al3+) on the scavenging reaction of a 2,2-diphenyl-1-picrylhydrazyl radical (DPPH˙), as a reactivity model of reactive oxygen species, with hydroquinone (QH2) and its methylated derivatives (MenQH2, n = 1–4) was investigated using stopped-flow and electrochemical techniques in a hydroalcoholic medium. The second-order rate constants (k) for the DPPH˙-scavenging reaction of the hydroquinones increased with the increasing number of methyl substituents. Upon addition of Al3+, the k values significantly increased depending on the concentration of Al3+. Such an accelerating effect of Al3+ on the DPPH˙-scavenging rates of the hydroquinones results from the remarkable positive shift of the one-electron reduction potential (Ered) of DPPH˙ in the presence of Al3+. These results demonstrate that Al3+, a strong Lewis acid, can act as a radical-scavenging promoter by stabilising the one-electron reduced species of the radical, although Al3+ is reported not only to act as a pro-oxidant but also to strongly interact with biomolecules, showing toxicities.
Introduction
Metal ions are known to play important roles in controlling biological redox reactions.1 It is also well known that metal ions accelerate electron-transfer reactions by binding to electron acceptors.2 Furthermore, they are reported to form transient complexes with the superoxide anion (O2˙−), which is one of the reactive oxygen species (ROS).3,4 Among them, a complex of Al3+ with O2˙− contributes to promote the Fenton reaction by reducing Fe3+ to Fe2+ to produce hydroxyl radicals (˙OH), the strongest ROS.5 In addition, Al3+ as a strong Lewis acid forms a variety of stable complexes with proteins and biomolecules, such as citrate, glutamate and α-ketoglutarate.6 Al3+ also interacts with molecules containing phosphate groups, such as AMP, ATP, RNA and DNA.7 These complexes disrupt the cell metabolic processes, leading to neurological diseases.8
Ubiquinol (UQH2), the reduced form of coenzyme Q10, has a hydroquinone (QH2) structure as an active centre, showing an efficient scavenging activity against ROS.9 In human beings, ROS are generated as side products during the energy production by mitochondrial electron-transport systems or the elimination of virus and bacteria in macrophages and neutrophils. However, excessive ROS cause many diseases, such as cancer, diabetes, cardiovascular disease, Alzheimer's and Parkinson's disease by oxidising proteins, lipids and DNA.10,11 Thus, antioxidants are very useful to protect against oxidative stress and may lead to prevention of these diseases. UQH2 is one of the lipid-soluble antioxidants and efficiently scavenges lipid peroxyl radicals.12,13 Furthermore, UQH2 reacts with the α-tocopheroxyl radical to regenerate α-tocopherol inside the biomembrane.14–16 On the other hand, QH2 is a major benzene metabolite and is widely applied in human and industrial activities. QH2 is used as an antioxidant in the rubber and food industries due to its antioxidative activity.17 QH2 also has a long history of use in pigmentary disorders and is applied in the commercially available cosmetic skin lightning formulations.18–20
The radical-scavenging reactions of phenolic antioxidants, such as flavonoids and α-tocopherol, are affected by redox-inactive metal ions, such as Mg2+, Al3+ and Sc3+.21–23 However, little is known about the effects of metal ions on the radical-scavenging reaction of QH2 and its derivatives. Particularly, whether Al3+ promotes the radical-scavenging reactions of antioxidants or acts as a pro-oxidant is yet to be clarified.
We report herein the effects of Al3+ on the scavenging reaction of a 2,2-diphenyl-1-picrylhydrazyl radical (DPPH˙) (Fig. 1) with QH2 and its methylated derivatives, which are methylhydroquinone (MeQH2), 2,6-dimethylhydroquinone (Me2QH2), trimethylhydroquinone (Me3QH2) and tetramethylhydroquinone (Me4QH2) (Fig. 2).24 DPPH˙ is frequently used as a reactivity model of peroxyl radicals, which are relatively less reactive ROS compared to hydroxyl and alkoxyl radicals.25–30 In addition, we investigated the effects of the number of methyl substituents of the hydroquinones on the DPPH˙-scavenging activity. Furthermore, the electron-donating ability of the hydroquinones and the electron-accepting ability of DPPH˙ were also evaluated by cyclic voltammetry (CV) and second-harmonic alternating current voltammetry (SHACV) in the absence and presence of Al3+.31–35 The strong Lewis acidity of Al3+ enhanced the electron-accepting ability of DPPH˙, leading to an acceleration of the DPPH˙-scavenging reaction by the hydroquinones.
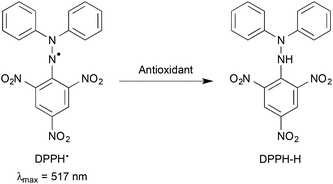 |
| Fig. 1 DPPH˙ as a model of ROS. | |
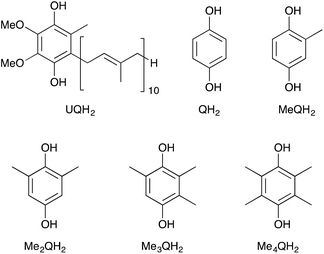 |
| Fig. 2 Structures of UQH2, QH2 and its methylated derivatives. | |
Experimental
Materials
QH2 and its methylated derivatives were purchased from Tokyo Chemical Industry Co., Ltd, Japan. DPPH˙ was purchased from Wako Pure Chemical industries, Ltd. Tetra-n-butylammonium perchlorate (Bu4NClO4), used as a supporting electrolyte for the electrochemical measurements, was purchased from Tokyo Chemical Industry Co., Ltd, Japan. Bu4NClO4 was recrystallised from ethanol (EtOH) and dried under vacuum at 313 K. Aluminium perchlorate nonahydrate [Al(ClO4)3·9H2O] was purchased from Wako Pure Chemical Ind. Ltd, Japan. EtOH (spectral grade) used as a solvent was purchased from Nacalai Tesque, Inc., Japan.
Spectral and kinetic measurements
To avoid the effect of molecular oxygen (O2), the reactions were carried out under strictly deaerated conditions. A continuous flow of argon gas was bubbled through an EtOH–H2O (9
:
1 v/v) solution (3.0 mL) containing DPPH˙ (5.0–8.9 × 10−5 M) or the hydroquinones (1.1–6.6 × 10−3 M) in a reservoir of the spectrophotometer for 7 min. The rates of the DPPH˙-scavenging reactions of the hydroquinones were determined by monitoring the absorbance change at 517 nm due to DPPH˙ using a stopped-flow technique on a UNISOKU RSP-1000-02NM spectrophotometer. The pseudo-first-order rate constants (kobs) were determined by least-squares curve fit using an Apple MacBook Pro personal computer. The first-order plots of ln(A − A∞) vs. time (A and A∞ denoted as the absorbance at the reaction time and the final absorbance, respectively) were linear until three or more half-lives with the correlation coefficient ρ > 0.999. The reaction of hydroquinones with DPPH˙ in the presence of various concentrations of Al(ClO4)3 was carried out in the same manner.
Electrochemical measurements
The cyclic voltammetry (CV) and second-harmonic alternating current voltammetry (SHACV)36 measurements were performed on an ALS-630A electrochemical analyser in deaerated EtOH–H2O (9
:
1 v/v) containing 0.1 M Bu4NClO4 as a supporting electrolyte. The glassy carbon-working electrode (BAS) was polished with BAS polishing alumina suspension and rinsed with methanol before use. The counter electrode was a platinum wire. The measured potentials were recorded with respect to an Ag/AgNO3 (0.01 M) reference electrode. One-electron oxidation potentials (Eox) of hydroquinones and one-electron reduction potentials (Ered) of DPPH˙ (vs. Ag/AgNO3) were converted to those vs. SCE by adding 0.29 V.37 All electrochemical measurements were carried out at room temperature under 1 atm Ar.
pH measurements
The pH values of the solutions of hydroquinones in the presence or absence of the metal ion in EtOH–H2O (9
:
1 v/v) were measured by using a METTLER TOLEDO SevenEasy pH meter.
Results and discussion
Kinetics of the DPPH˙-scavenging reaction of the hydroquinones in the presence or absence of Al3+
The rate of the DPPH˙-scavenging reaction of the hydroquinones was measured by monitoring the absorbance change at 517 nm due to DPPH˙ using a stopped-flow technique in deaerated EtOH–H2O (9
:
1 v/v) at 298 K. The decay of the absorbance at 517 nm obeyed pseudo-first-order kinetics, when the concentration of the hydroquinones ([MenQH2]) (n = 0–4) was maintained at a more than 10-fold excess of the DPPH˙ concentration. The pseudo-first-order rate constants (kobs) increase with increasing [MenQH2], exhibiting the first order dependence on [MenQH2] (Fig. 3). From the slope of the linear plots of kobsvs. [MenQH2], the second-order rate constants (k) were determined for the DPPH˙-scavenging reaction (Table 1). The k values of the hydroquinones increased with the increasing number of methyl substituents in the absence of metal ions. Upon addition of Al(ClO4)3 (0.05–0.50 M) to the hydroquinone–DPPH˙ system, the k values for all the hydroquinones significantly increased with the increasing Al(ClO4)3 concentration (Fig. 4, Table 1).
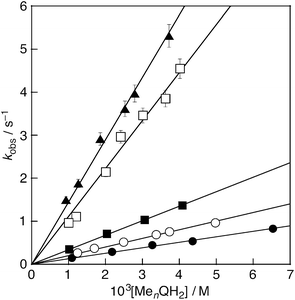 |
| Fig. 3 Plots of kobsvs. [MenQH2] (n = 0–4) for the reaction of QH2 (closed circles), MeQH2 (open circles), Me2QH2 (closed squares), Me3QH2 (open squares) and Me4QH4 (closed triangles) with DPPH˙ in deaerated EtOH–H2O (9 : 1 v/v) at 298 K. | |
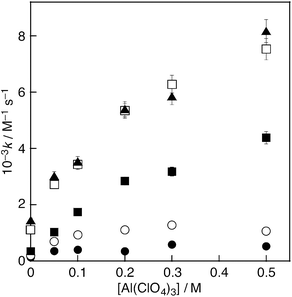 |
| Fig. 4 Plots of k vs. [Al(ClO4)3] for the reaction of QH2 (closed circles), MeQH2 (open circles), Me2QH2 (closed squares), Me3QH2 (open squares) and Me4QH4 (closed triangles) with DPPH˙ in deaerated EtOH–H2O (9 : 1 v/v) at 298 K. | |
Table 1 Second-order rate constants (k) for the DPPH˙-scavenging reaction of the hydroquinones in the presence or absence of Al(ClO4)3 in deaerated EtOH–H2O (9
:
1 v/v) at 298 K
|
k/M−1 s−1 |
[Al(ClO4)3]/M |
QH2 |
MeQH2 |
Me2QH2 |
Me3QH2 |
Me4QH2 |
0 |
1.3 × 102 |
2.0 × 102 |
3.4 × 102 |
1.1 × 103 |
1.4 × 103 |
0.05 |
3.5 × 102 |
7.0 × 102 |
1.0 × 103 |
2.7 × 103 |
3.0 × 103 |
0.1 |
3.9 × 102 |
9.3 × 102 |
1.7 × 103 |
3.4 × 103 |
3.5 × 103 |
0.2 |
3.4 × 102 |
1.1 × 103 |
2.8 × 103 |
5.3 × 103 |
5.4 × 103 |
0.3 |
5.8 × 102 |
1.3 × 103 |
3.2 × 103 |
6.3 × 103 |
5.8 × 103 |
0.5 |
5.2 × 102 |
1.1 × 103 |
4.4 × 103 |
7.5 × 103 |
8.2 × 103 |
Effect of Al3+ on the redox behaviour of the hydroquinones and DPPH˙
The one-electron oxidation potentials (Eox) of the hydroquinones were determined by cyclic voltammetry (CV) and second-harmonic alternating current voltammetry (SHACV)36 measurements in the presence or absence of Al3+ in EtOH–H2O (9
:
1 v/v) to investigate the detailed effects of Al3+ on the DPPH˙-scavenging reaction of the hydroquinones. The Eox values of the hydroquinones were determined from the intersection of SHACV waves (Fig. 5). In the absence of the metal ion, the Eox values of the hydroquinones were shifted to the negative direction with the increasing number of methyl substituents (Table 2). The electron-donating methyl substituents contribute to an increase in the electron density, leading to an enhancement of the electron-donating ability of the hydroquinones.
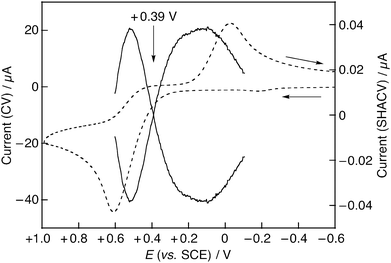 |
| Fig. 5 Cyclic voltammogram (CV) (dashed line) and second-harmonic alternating current voltammogram (SHACV) (solid line) of QH2 (2.4 × 10−3 M) recorded at the scan rate of 0.1 V s−1 and 4 mV s−1, respectively, on a glassy carbon working electrode in EtOH–H2O (9 : 1 v/v) (0.1 M Bu4NClO4). | |
Table 2 The Eox values of hydroquinones and the Ered values of DPPH˙ in EtOH–H2O (9
:
1 v/v) in the presence or absence of Al(ClO4)3
|
E
ox (vs. SCE)a/V |
E
red (vs. SCE)/V |
[Al(ClO4)3]/M |
QH2 |
MeQH2 |
Me2QH2 |
Me3QH2 |
Me4QH2 |
DPPH˙ |
Determined by SHACV at the rate of 4 mV s−1 on a glassy carbon working electrode (0.1 M Bu4NClO4).
|
0 |
+0.39 |
+0.36 |
+0.29 |
+0.23 |
+0.19 |
+0.23 |
0.05 |
+0.48 |
+0.42 |
+0.36 |
+0.29 |
+0.24 |
+0.67a |
0.1 |
+0.54 |
+0.47 |
+0.39 |
+0.33 |
+0.28 |
+0.69a |
0.2 |
+0.55 |
+0.48 |
+0.40 |
+0.34 |
+0.28 |
+0.70a |
0.3 |
+0.56 |
+0.50 |
+0.42 |
+0.36 |
+0.30 |
+0.70a |
0.5 |
+0.56 |
+0.50 |
+0.42 |
+0.36 |
+0.32 |
+0.69a |
Upon addition of Al(ClO4)3 (0.05–0.5 M), the Eox values of hydroquinones were significantly shifted to the positive direction depending on the concentration of Al3+ (Table 2). Thus, the one-electron donating ability of the hydroquinones was decreased in the presence of Al3+. The pKa values of the hydroquinones in H2O and DMSO are listed in Table 3.38,39 The pH values of the hydroquinone solutions in the presence or absence of Al(ClO4)3 in EtOH–H2O (9
:
1 v/v) are shown in Table 4. The pKa values of the hydroquinones used in this study in aqueous solution are in the range of 9.9–11.3 (Table 3). Thus, all the hydroquinones do not undergo deprotonation and exist in their neutral forms. The one-electron reduction potential (Ered) of DPPH˙ was also determined using CV in the presence or absence of Al3+. In the absence of Al3+, two well-defined reversible redox waves were observed as shown in Fig. 6 (dashed line), from which the one-electron reduction potential of DPPH˙ was determined to be +0.23 V vs. SCE. In the presence of 0.1 M Al(ClO4)3, however, the cyclic voltammogram of DPPH˙ was remarkably changed and the Ered value of DPPH˙ was significantly shifted to the positive direction (Fig. 6, Table 2). These results indicated that the DPPH anion (DPPH−), the one-electron reduced species of DPPH˙, strongly interacted with Al3+ to enhance the electron-accepting ability of DPPH˙. The effects of metal ions on the Ered of DPPH˙ differed by the Lewis acidity.40
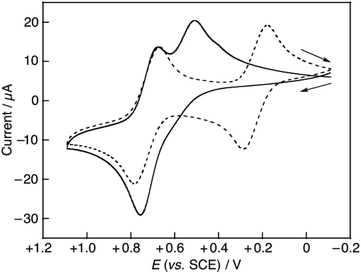 |
| Fig. 6 Cyclic voltammograms of DPPH˙ (2.0 × 10−3 M) in the absence (dashed line) and presence (solid line) of 0.1 M Al(ClO4)3 recorded at the scan rate 0.1 V s−1 on a glassy carbon working electrode in EtOH–H2O (9 : 1 v/v) (0.1 M Bu4NClO4). | |
Table 3 The pKa values of hydroquinones
|
QH2 |
MeQH2 |
Me2QH2 |
Me3QH2 |
Me4QH2 |
The pKa values in aqueous solution.38
The pKa values in DMSO.39
|
pKa |
9.9a |
10.1a |
— |
— |
11.3a |
19.1b |
18.3b |
19.0b |
17.8b |
20.0b |
Table 4 The pH values of hydroquinone solutions in EtOH–H2O (9
:
1 v/v) containing 0.1 M Bu4NClO4 in the presence or absence of Al(ClO4)
[Al(ClO4)3]/M |
QH2 |
MeQH2 |
Me2QH2 |
Me3QH2 |
Me4QH2 |
0 |
5.5 |
4.7 |
4.7 |
4.5 |
4.6 |
0.05 |
2.1 |
2.2 |
2.1 |
2.1 |
2.1 |
0.1 |
1.7 |
1.8 |
1.6 |
1.7 |
1.7 |
0.2 |
1.5 |
1.5 |
2.0 |
1.6 |
1.5 |
0.3 |
1.3 |
1.1 |
1.2 |
1.1 |
1.1 |
0.5 |
0.9 |
0.7 |
0.8 |
0.8 |
0.8 |
We previously reported that the radical-scavenging mechanism of a vitamin E model is significantly affected by solvents.22b In a protic solvent, such as methanol, an electron transfer from the vitamin E model to radicals followed by proton transfer is favorable compared to one-step hydrogen-atom transfer. Based on these results, the DPPH˙-scavenging reaction by hydroquinones in the hydroalcoholic medium would proceed via the electron transfer. The free energy change (ΔGet) of the electron transfer from hydroquinones to DPPH˙ is calculated by using eqn (1),
where
F is the Faraday constant, which is negative (Δ
Get < 0) in the presence of Al
3+. Al
3+ enhanced the electron transfer by strongly stabilizing DPPH
− and increased the
k values of the hydroquinones depending on its concentration, in spite of decreasing the one-electron donor ability of hydroquinones.
Fig. 7 shows the free-energy-change dependence of log
k for the radical-scavenging reaction of DPPH˙ with a series of hydroquinones in the absence and presence of Al3+ (0.5 M).
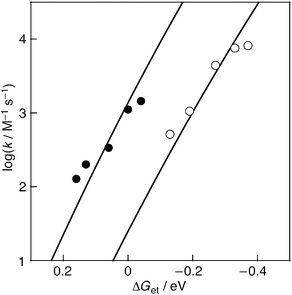 |
| Fig. 7 Plots of log k vs. ΔGet for the DPPH˙-scavenging reaction by the hydroquinone derivatives in the absence of metal ions (closed circles) and the presence of Al3+ (0.5 M) (open circles). The lines represent the curve fits to eqn (2) with λ = 1.78 and 2.19 eV for the reaction without and with Al3+ (0.5 M), respectively. | |
The dependence of outer-sphere electron transfer has been well established by Marcus as given by eqn (2),41
| 1/k = 1/kdiff + 1/{Zexp[–(λ/4)(1 + ΔGet/λ)2/kBT]} | (2) |
in which
kdiff is the diffusion rate constant (10
10 M
−1 s
−1),
42,43λ is the reorganization energy of electron transfer,
kB is the Boltzmann constant and
Z is the collision frequency (1 × 10
11 M
−1 s
−1). The
λ value is determined to be 1.78 eV for the radical-scavenging reaction in the absence of metal ions. In the presence of Al
3+, the rate constants increase with a decrease in the Δ
Get value as shown in
Fig. 7. The
λ value in the presence of Al
3+ is 2.19 eV, which is larger than that in the absence of metal ions, suggesting that trivalent Al
3+ strongly binds to DPPH
− as an electron transfer product in electron transfer from the hydroquinones to DPPH˙.
2,44
Conclusions
The DPPH˙-scavenging activity of the hydroquinones increased with the increasing number of methyl substituents in the dearated hydroalcoholic medium. Upon addition of Al(ClO4)3 to the hydroquinone–DPPH˙ system, the k values of the hydroquinones were significantly increased depending on the concentration of Al3+, in spite of decreasing the electron-donating ability of the hydroquinones. Al3+, one of the strong Lewis acids, strongly stabilized the DPPH− generated by the electron transfer from the hydroquinones to DPPH˙. In addition, the electron transfer occurred voluntarily (ΔGet < 0) in the presence of Al3+ according to the electrochemical data. The strong Lewis acids, such as Al3+, significantly accelerated the electron-transfer reaction and enhanced the radical-scavenging reaction of phenolic antioxidants. The results obtained in this study demonstrate that Al3+, a strong Lewis acid, can act as a radical-scavenging promoter by stabilising the one-electron reduced species of the radical, although Al3+ is reported not only to act as a pro-oxidant but also to strongly interact with biomolecules, showing toxicities.
Acknowledgements
This work was partially supported by Grant-in-Aid (No. 26460056 to I. N., 26620154, 266288037 and 16K13964 to K. O.) from the Ministry of Education, Culture, Sports, Science and Technology, Japan, Acenet Inc. Foundation (to K. O.) and SENTAN projects from JST (to S. F.). We thank Mr T. Waki (Chiba University) for his help in the measurements of redox potentials.
Notes and references
-
W. Kaim, B. Schwederski and A. Klein, Bioinorganic Chemistry: Inorganic Elements in the Chemistry of Life, John Wiley & Son, Chichester, 2nd edn, 2013 Search PubMed.
-
(a) S. Fukuzumi and K. Ohkubo, Coord. Chem. Rev., 2010, 254, 372 CrossRef CAS;
(b) S. Fukuzumi, K. Ohkubo and Y. Morimoto, Phys. Chem. Chem. Phys., 2012, 14, 8472 RSC.
-
(a) S. Fukuzumi and K. Ohkubo, Chem. – Eur. J., 2000, 6, 4532 CrossRef CAS PubMed;
(b) K. Ohkubo, S. C. Menon, A. Orita, J. Otera and S. Fukuzumi, J. Org. Chem., 2003, 68, 4720–4726 CrossRef CAS PubMed.
- J. I. Mujika, F. Ruipérez, I. Infante, J. M. Ugalde, C. Exley and X. Lopez, J. Phys. Chem. A, 2011, 115, 6717 CrossRef CAS PubMed.
- F. Ruipérez, J. I. Mujika, J. M. Ugalde, C. Exley and Z. Lopez, J. Inorg. Biochem., 2012, 117, 118 CrossRef PubMed.
-
(a) J. I. Mujika, J. M. Ugalde and X. Lopez, J. Phys. Chem. B, 2014, 118, 6680 CrossRef CAS PubMed;
(b) J. I. Mujika, J. M. Ugalde and X. Lopes, Phys. Chem. Chem. Phys., 2012, 14, 12465 RSC.
- N. B. Luque, J. I. Mujika, E. Rezabal, J. M. Ugalde and X. Lopez, Phys. Chem. Chem. Phys., 2014, 16, 20107 RSC.
- C. A. Shaw, S. Seneff, S. D. Kette, L. Tomljenovic, J. W. Oller Jr. and R. M. Davidson, J. Toxicol., 2014, 491316 Search PubMed.
-
(a) A. Maroz, R. F. Anderson, R. A. Smith and M. P. Murphy, Free Radical Biol. Med., 2009, 465, 105 CrossRef PubMed;
(b) A. M. James, R. A. Smith and M. P. Murphy, Arch. Biochem. Biophys., 2004, 423, 47 CrossRef CAS PubMed;
(c) T. Kawashima, K. Ohkubo and S. Fukuzumi, Phys. Chem. Chem. Phys., 2011, 13, 3344 RSC.
- I. F. Benzie, Comp. Biochem. Physiol., Part A: Mol. Integr. Physiol., 2003, 136, 113 CrossRef.
- K. Rahman, Clin. Interventions Aging, 2007, 2, 219 CAS.
-
(a) P. Forsmark-Andree, G. Dallner and L. Ernster, Free Radicals Biol. Med., 1995, 19, 749 CrossRef CAS;
(b) L. Ernster and G. Dallner, Biochim. Biophys. Acta, 1995, 1271, 195 CrossRef PubMed.
- B. Frei, M. C. Kim and B. N. Ames, Proc. Natl. Acad. ScI. U. S. A., 1990, 87, 4879 CrossRef CAS.
-
(a) K. Mukai, A. Ouchi, S. Nakaya and S. Nagaoka, J. Phys. Chem. B, 2013, 117, 8378 CrossRef CAS PubMed;
(b) A. Ouchi, S. Nagaoka and K. Mukai, J. Phys. Chem. B, 2010, 114, 6601 CrossRef CAS PubMed;
(c) S. Itoh, S. Nakaya and K. Mukai, J. Phys. Chem. A., 2008, 112, 448 CrossRef CAS PubMed.
-
(a) Y. Yamamoto, J. Dermatol. Sci., 2001, 27, S1 CrossRef CAS PubMed;
(b) Y. Yamamoto, E. Komuro and E. Niki, J. Nutr. Sci. Vitaminol., 1990, 36, 505 CrossRef CAS PubMed.
- K. U. Ingold, V. W. Bowry, R. Stocker and C. Walling, Proc. Natl. Acad. Sci. U. S. A., 1993, 90, 45 CrossRef CAS.
-
IPCS, Hydroquinone, Environmental Health Criteria 157, 1994 Search PubMed.
- J. Ancerewicz, E. Migliavacca, P. A. Carrupt, B. Testa, F. Brée, R. Zini, J. P. Tillement, S. Labidalle, D. Guyot, A. M. Chauvet-Monges, A. Crevat and A. L. Ridant, Free Radical Biol. Med., 1998, 25, 113 CrossRef CAS PubMed.
- M. Nishizawa, M. Kohono, M. Nishimura, A. Kitagawa and Y. Niwano, Chem. Pharm. Bull., 2005, 53, 714 CrossRef CAS PubMed.
- T. W. Tse, J. Dermatol. Treat., 2010, 21, 272 CrossRef PubMed.
- T. Waki, S. Kobayashi, K. Matsumoto, T. Ozawa, T. Kamada and I. Nakanishi, Chem. Commun., 2013, 49, 9842 RSC.
-
(a) I. Nakanishi, T. Shimada, K. Ohkubo, S. Manda, T. Shimizu, S. Urano, H. Okuda, N. Miyata, T. Ozawa, K. Anzai, S. Fukuzumi, N. Ikota and K. Fukuhara, Chem. Lett., 2007, 36, 1276 CrossRef CAS;
(b) I. Nakanishi, T. Kawashima, K. Ohkubo, H. Kanazawa, K. Inami, M. Mochizuki, K. Fukuhara, H. Okuda, T. Ozawa, S. Itoh, S. Fukuzumi and N. Ikota, Org. Biomol. Chem., 2005, 3, 626 RSC;
(c) I. Nakanishi, K. Ohkubo, K. Miyazaki, W. Hakamata, S. Urano, T. Ozawa, H. Okuda, S. Fukuzumi, N. Ikota and K. Fukuhara, Chem. Res. Toxicol., 2004, 17, 26 CrossRef CAS PubMed;
(d) I. Nakanishi, Y. Uto, K. Ohkubo, K. Miyazaki, H. Yakumaru, S. Urano, H. Okuda, J. Ueda, T. Ozawa, K. Fukuhara, S. Fukuzumi, H. Nagasawa, H. Hori and N. Ikota, Org. Biomol. Chem., 2003, 1, 1452 RSC;
(e) I. Nakanishi, K. Miyazaki, T. Shimada, K. Ohkubo, S. Urano, N. Ikota, T. Ozawa, S. Fukuzumi and K. Fukuhara, J. Phys. Chem. A, 2002, 106, 11123 CrossRef CAS;
(f) I. Nakanishi, K. Fukuhara, T. Shimada, K. Ohkubo, Y. Iizuka, K. Inami, M. Mochizuki, S. Urano, S. Itoh, N. Miyata and S. Fukuzumi, J. Chem. Soc., Perkin Trans. 2, 2002, 1520 RSC.
-
(a) K. Mukai, M. Oi, A. Ouchi and S. Nagaoka, J. Phys. Chem. B, 2012, 116, 2615 CrossRef CAS PubMed;
(b) Y. Kohno, M. Fujii, C. Matsuoka, H. Hashimoto, A. Ouchi, S. Nagaoka and K. Mukai, J. Phys. Chem. B, 2011, 115, 9880 CrossRef CAS PubMed;
(c) A. Ouchi, S. Nagaoka, K. Abe and K. Abe, J. Phys. Chem. B, 2009, 113, 13322 CrossRef CAS PubMed.
- S. B. Kedrare and R. P. Singh, J. Food Sci. Technol., 2011, 48, 412 CrossRef PubMed.
- M. J. Ajitha, S. Mohanlal, C. H. Suresh and A. Jayalekshmy, J. Agric. Food Chem., 2012, 60, 3693 CrossRef CAS PubMed.
- M. Hidalgo, C. Sánchez-Moreno and S. Pascual-Teresa, Food Chem., 2010, 121, 691 CrossRef CAS.
- M. Locatelli, R. Gindro, F. Travaglia, J. Coïsson, M. Rinaldi and M. Arlorio, Food Chem., 2009, 114, 889 CrossRef CAS.
- O. P. Shama and T. K. Bhat, Food Chem., 2009, 113, 1202 CrossRef.
- M. C. Foti and C. Daquino, Chem. Commun., 2006, 30, 3252 RSC.
- W. Hakamata, I. Nakanishi, Y. Masuda, T. Shimizu, H. Higuchi, Y. Nakamura, S. Saito, S. Urano, T. Oku, T. Ozawa, N. Ikota, N. Miyata, H. Okuda and K. Fukuhara, J. Am. Chem. Soc., 2006, 128, 6524 CrossRef CAS PubMed.
- A. W. Taylor, S. Puttick and P. Licence, J. Am. Chem. Soc., 2012, 134, 15636 CrossRef CAS PubMed.
- I. Nakanishi, K. Ohkubo, K. Miyazaki, W. Hakamata, S. Urano, T. Ozawa, H. Okuda, S. Fukuzumi, N. Ikota and K. Fukuhara, Chem. Res. Toxicol., 2004, 17, 26 CrossRef CAS PubMed.
-
(a) A. M. Bond and D. E. Smith, Anal. Chem., 1974, 46, 1946 CrossRef CAS;
(b) T. G. McCord and D. E. Smith, Anal. Chem., 1969, 41, 1423 CrossRef CAS.
- E. M. Arnett, K. Amarnath, N. G. Harvey and J. P. Cheng, J. Am. Chem. Soc., 1990, 112, 344 CrossRef CAS.
- M. R. Wasielewski and R. Breslow, J. Am. Chem. Soc., 1976, 98, 4222 CrossRef CAS.
-
A. J. Bard and L. R. Faulkner, Electrochemical Methods, Fundamental and Applications, John Wiley & Sons, New York, 2001, ch. 10, pp. 368–416 Search PubMed.
-
C. K. Mann and K. K. Barnes, Electrochemical Reactions in Non-aqueous Systems, Mercel Dekker, New York, 1970 Search PubMed.
- J. H. Baxendale and H. R. Hardy, Trans. Faraday Soc., 1953, 49, 1140 RSC.
- X. O. Zhu, C. H. Wang and H. Liang, J. Org. Chem., 2010, 75, 7240 CrossRef CAS PubMed.
-
(a) R. G. Pearson, J. Chem. Educ., 1968, 45, 581 CrossRef CAS;
(b) R. G. Peason, J. Chem. Educ., 1968, 45, 643 CrossRef.
-
(a) R. A. Marcus, Annu. Rev. Phys. Chem., 1964, 15, 155 CrossRef CAS;
(b) R. A. Marcus, Angew. Chem., Int. Ed. Engl., 1993, 32, 1111 CrossRef;
(c) R. A. Marcus and N. Sutin, Biochim. Biophys. Acta, 1985, 811, 265 CrossRef CAS.
-
(a) H. Shizuka, M. Nakamura and T. Morirta, J. Phys. Chem., 1980, 84, 989 CrossRef CAS;
(b) H. Shizuka, T. Saito and T. Morirta, Chem. Phys. Lett., 1978, 56, 519 CrossRef CAS.
- The term 1/kdiff is negligible where kdiff is significantly larger than k in this study.
- Large reorganization energies of electron transfer in the presence of a strong Lewis acid have been reported and discussed therein.
(a) K. Ohkubo, R. Garcia, P. J. Sintic, T. Khoury, M. J. Crossley, K. M. Kadish and S. Fukuzumi, Chem.–Eur. J., 2009, 15, 10493 CrossRef CAS PubMed;
(b) K. Okamoto, H. Imahori and S. Fukuzumi, J. Am. Chem. Soc., 2003, 125, 7014 CrossRef CAS PubMed;
(c) K. Okamoto, Y. Mori, H. Yamada, H. Imahori and S. Fukuzumi, Chem. – Eur. J., 2004, 10, 474 CrossRef CAS PubMed.
|
This journal is © The Royal Society of Chemistry 2016 |
Click here to see how this site uses Cookies. View our privacy policy here.