DOI:
10.1039/C5NR06876B
(Paper)
Nanoscale, 2016,
8, 309-317
Chitosan microspheres with an extracellular matrix-mimicking nanofibrous structure as cell-carrier building blocks for bottom-up cartilage tissue engineering†
Received
5th October 2015
, Accepted 10th November 2015
First published on 12th November 2015
Abstract
Scaffolds for tissue engineering (TE) which closely mimic the physicochemical properties of the natural extracellular matrix (ECM) have been proven to advantageously favor cell attachment, proliferation, migration and new tissue formation. Recently, as a valuable alternative, a bottom-up TE approach utilizing cell-loaded micrometer-scale modular components as building blocks to reconstruct a new tissue in vitro or in vivo has been proved to demonstrate a number of desirable advantages compared with the traditional bulk scaffold based top-down TE approach. Nevertheless, micro-components with an ECM-mimicking nanofibrous structure are still very scarce and highly desirable. Chitosan (CS), an accessible natural polymer, has demonstrated appealing intrinsic properties and promising application potential for TE, especially the cartilage tissue regeneration. According to this background, we report here the fabrication of chitosan microspheres with an ECM-mimicking nanofibrous structure for the first time based on a physical gelation process. By combining this physical fabrication procedure with microfluidic technology, uniform CS microspheres (CMS) with controlled nanofibrous microstructure and tunable sizes can be facilely obtained. Especially, no potentially toxic or denaturizing chemical crosslinking agent was introduced into the products. Notably, in vitro chondrocyte culture tests revealed that enhanced cell attachment and proliferation were realized, and a macroscopic 3D geometrically shaped cartilage-like composite can be easily constructed with the nanofibrous CMS (NCMS) and chondrocytes, which demonstrate significant application potential of NCMS as the bottom-up cell-carrier components for cartilage tissue engineering.
Introduction
Biological tissues are composed of spatially organized cells supported by an extracellular matrix (ECM) that provides the most favorably spaced binding sites and geometrical signals to direct cellular behavior and cell fate on the basis of its characteristic 3D nanofibrous microstructure.1–6 The reconstruction of tissue defects, especially of bone and cartilage, is eagerly anticipated in tissue engineering (TE) and regenerative medicine, which proposes stringent requirements for designing serviceable artificial scaffolds.7 The optimal TE artificial scaffolds are those that most closely mimic the physicochemical properties of the natural ECM and favor cell attachment, proliferation, migration and new tissue formation.8,9
One effective approach is by utilizing a 3D biodegradable scaffold with certain critical ECM-mimicking features such as an artificial ECM to seed target cells followed by in vivo implantation to repair tissue defects.10 As expected, scaffolds with an ECM-mimicking nanofibrous structure have been proven to advantageously enhance cell–scaffold interactions compared with those without an ECM-mimicking structure.11–15 However, several hurdles, such as the complicated preparation methodology, inhomogeneous cell distribution, and limited nutrient diffusion, have greatly hampered their clinical applications.16
Recently, as a valuable alternative, a bottom-up TE approach utilizing cell-loaded micrometer-scale modular components as building blocks has been proposed, and it demonstrates a number of desirable advantages for both in vitro and in vivo construction of large geometrically shaped new tissue compared with bulk 3D scaffolds.17–22 It can be anticipated that if the modular components are made with an ECM-mimicking nanofibrous structure, improved cell–material interactions and cell proliferation should be achieved. Ma and co-workers have reported that poly(L-lactic acid) based hollow microspheres with an ECM-mimicking nanofibrous architecture advantageously enhances cell–material interactions and facilitates tissue regeneration.23 Recently, Zhang et al. also revealed that ECM-mimicking nanofibrous chitin microspheres showed a high cell attachment efficiency.24 Despite the achievements, few studies have reported the fabrication of ECM-mimicking nanofibrous micro-components for tissue regeneration to date.25,26 Therefore, more nanofibrous candidates which are biocompatible, biodegradable, size-controllable and cost-effective are highly desirable to provide more options for regenerative medicine.
Chitosan (CS), an abundant natural linear polysaccharide, which is known to possess appealing intrinsic properties such as biocompatibility, bioactivity and biodegradability, has been widely used in TE.11,27,28 Particularly, it is structurally similar to glycosaminoglycan (GAG),8 which is one of the principal components of the natural cartilage-specific ECM. Furthermore, it can enhance chondrogenesis,29 and show promising application potential for cartilage defect repair.8 Nevertheless, it has never been reported that chitosan was fabricated into ECM-mimicking nanofibrous micro-components.
We report here the preparation of chitosan microspheres (CMS) with an ECM-mimicking nanofibrous structure for the first time based on physical hydrogels of CS through the direct alkaline induced gelation of chitosan microsphere emulsions (Scheme 1). In contrast to the previously reported chemical crosslinking method for solidifying CS microspheres,30–33 this physical gelation process not only makes chitosan chains to be assembled into a nanofiber network with controlled morphology,11,24,34,35 but also avoids introducing any potentially toxic or denaturizing chemical crosslinking agent. Furthermore, by utilizing microfluidic technology, a powerful manufacturing technique for the preparation of hierarchical micro-particles,36–43 uniform CMS with tunable sizes (165–425 μm) were obtained to facilitate the actual requirements of tissue regeneration. Notably, the supercritical carbon dioxide (scCO2) drying technique was applied here to avoid the structural collapse of the NCMS, and allowed us to observe the initial nanofibrous structure of the obtained products.44–46In vitro chondrocyte culture revealed that an enhanced cell–material interaction was realized, and a macroscopic 3D geometrically shaped cartilage-like composite was constructed with the NCMS and chondrocytes can be easily obtained. These perfect results demonstrate significant application potential of NCMS as the bottom-up cell-carrier components for repairing cartilage defects.
 |
| Scheme 1 Schematic representation of the NCMS-based bottom-up TE approach for in vitro cartilage-like composite. | |
Experimental section
Materials
All chemicals are of analytical grade and were supplied by Sinopharm Chemical Reagent Co., Ltd., China. Chitosan (with a viscosity of 50–800 mPa s and the degree of N-deacetylation (DD) of 80–95%) solution with a concentration of 1–2% (w/v) was prepared by dissolving 1–2 g chitosan powder in 1–2% (v/v) acetic acid solution and then the solution was centrifuged to remove the air bubbles and the insoluble impurities. The oil phase was prepared by adding 3% (v/v) span-80 to the cyclohexane. The collection NaOH solution was prepared by dissolving 3–12 g NaOH powder in 500 ml ethanol to form 0.15–0.6 M NaOH ethanol solution.
Preparation of microfluidics
The coaxial glass microcapillary emulsion device is assembled by using round and square glass capillaries on glass slides. The round glass capillary tubes were tapered to the desired orifice using a capillary puller (Sutter Instrument, P-97) and a microforge (Narishige, MF-830). The collection channel was evaporated with a thin layer of PDMS (235 °C for 2 h). Epoxy resin glue was used to seal the tubes where required.
Fabrication of nanofibrous CMS and solid CMS with smooth surface
For the preparation of NCMS, chitosan solution is the inner phase and cyclohexane with 3% (v/v) span 80 is the outer oil phase. The diameters of typical orifices of the inner channel and the collection channel were 60–90 μm and 300–400 μm, respectively. The flow rates for the outer and inner phases were set as 0.008–0.05 and 0.2–1.0 ml min−1, respectively. The generated CS emulsions were collected in NaOH ethanol solution and then washed with water, ethanol and acetone. After that, the solidified CMS were soaked in acetone for 24 h and dried by scCO2 (Speed SFE-helix, Applied Separations, USA). For the preparation of solid CMS, the solidified CMS were cross-linked by genipin (10% w/v) for 12 h, and then dried in a 60 °C oven.
Material characterization
Scanning electron microscopy (SEM) was performed on a Zeiss Supra 40 scanning electron microscope at an acceleration voltage of 5 kV. N2 sorption analysis was conducted using an ASAP 2020 accelerated surface area and porosimetry instrument (Micromeritics), equipped with automated surface area, at 77 K using Barrett–Emmett–Teller (BET) calculations for the surface area. The pore size distribution plot was recorded from the adsorption branch of the isotherm based on the Barrett–Joyner–Halenda (BJH) model. The average diameter of the microspheres and the nanofibers in the microspheres was determined by calculating them from SEM and the micrographs, where at least 20 measurements of the microspheres and 100 measurements of the nanofibers were taken. Their averages and standard deviations were reported.
Isolation and culture of chondrocytes
Primary cartilage chondrocytes were harvested from the femoral heads and knees (condyles and patellar grooves) of four-week-old New Zealand white rabbits under sterile conditions. Briefly, the cartilage was washed three times with Dulbecco's modified Eagle's medium (DMEM) and cut into 1 mm3 pieces. The pieces were digested overnight with 0.1% collagenase type II in DMEM at 37 °C in a 5% carbon dioxide (CO2) incubator. Chondrocytes were isolated by centrifugation at 1800 rpm for 10 min and washed twice with PBS. Following centrifugation, the supernatant was discarded, and the cells were resuspended in a basal culture medium consisting of DMEM with 10% fetal bovine serum (FBS), 100 U ml−1 penicillin, and 100 U ml−1 streptomycin. The cells were seeded in 10 cm cell-culture plates at an initial density of 40
000 cells per cm2. The chondrocytes were passaged (1
:
4) every 5 days, for a total of two passages after the initial plating, or in other words, until passage 2 (P2).
In vitro cytological evaluation
For cell adhesion and proliferation tests, the chondrocytes of passage 2 were mixed with the different microspheres (nanofibrous CMS and solid CMS) at a ratio of 10
:
1 and cultured in spinner flasks. DMEM with 10% fetal bovine serum was changed every 2 days. The mixture was pipetted out at different time points. Cells attached on the microspheres were harvested and counted by using an automatic cell counter. For the bottom-up construction of microscopic cartilage-like composite, the chondrocytes of passage 2 were mixed with the different microspheres at a ratio of 10
:
1 and cultured in spinner flasks for 7 days, then the mixture was transferred into molds with the shape of a 5 mm × 2 mm cake and cultured for another two weeks. DMEM with 10% fetal bovine serum was changed every 2 days.
DNA and GAG contents
The NCMS–cell mixture cultured in vitro were collected and digested with 1 ml of papain solution (300 mg mL−1 papain, 0.1 M sodium acetate (pH 6.0), 5 mM cysteine hydrochloric acid (HCl), 5 mM disodium salt EDTA (Na2EDTA)) at 60 °C for 16 h. The total DNA content was determined using a PicoGreen dsDNA quantitation kit (Invitrogen) according to the manufacturer's instructions. The glycosaminoglycan (GAG) content was quantified by adding the sample to 1,9 dimethylmethylene blue (16 mg mL−1 in glycine/NaCl, pH 3.0) dye solution using diluted shark chondroitin sulphate (Sigma Chemical Co.) solutions as the standard. The optical density was measured using a 595 nm filter.
SEM sample preparation
Specimens were fixed using 2.5% (v/v) glutaraldehyde in PBS at 4 °C for 24 h followed by rinsing in 1× PBS for 5 min. After that, the samples were washed three times with 100% tertiary butanol for 10 min. The samples were then dried by using a freeze dryer, and then sputter-coated with gold and observed under a SEM.
Histological and immunohistochemical analyses
The specimens were fixed, dehydrated, and subsequently embedded in paraffin. 4 μm thick sections were cut and deparaffinized, then stained with hematoxylin and eosin, DAPI, and safranin-O.
Mechanical testing
The compressive tests of the cartilage-like composite was performed by using an Instron 5565A, equipped with two flat-surface compression stages and 10 N load cells. The strain ramp rate was maintained at 0.2 mm s−1 for all of the tests.
Results and discussion
As illustrated in Scheme 1, a coaxial glass microcapillary emulsion device47,48 made in-house was used to prepare monodisperse and uniform CS emulsions (Fig. 1a). It is well-known that CS can only be dissolved in dilute acids but is insoluble in neutral deionized water. Thus, we can transform acidic CS liquid emulsions directly into solid microgels by neutralizing them with an alkaline solution. To obtain regular and rounded solid microgels, the emulsions in the oil phase should be rapidly immersed into an alkaline collection solution to permit the neutralization surrounding them to be homogeneous. However, NaOH aqueous solution could not work because the CS liquid emulsions could not pass through the mutually exclusive oil–water interface. So, we used here ethanol as the solvent to dissolve NaOH, and the collection solution could permit the CS emulsions to directly flow into it from the oil phase without obstruction and transform into CS microgels as the OH− subsequently penetrates into the CS emulsions (Fig. 1b). The mechanisms involved in this alkaline induced physical gelation of CS should be similar to previously reported multi-membrane hydrogels by Domard et al.,35 according to whom, the NH3+ sites were neutralized into NH2 and the ionic repulsions between the original CS chains disappeared, so that the rearrangement and entanglement of CS chains that occurred arise from hydrogen bonding, hydrophobic interactions and crystallite formation. This process thus leads CS chains to be self-assembled into a nanofiber network. By utilizing scCO2 drying, the formed nanofiber network of the CSM was retained and products with a nanofibrous microstructure were obtained (Fig. 1c–f). As depicted in Fig. 1e and 1f, we can see that the whole microsphere is consisted of a homogeneous nanofiber network, which exhibits a great similarity to the nanofibrous structure of native ECM of the cartilage (Fig. 1g).
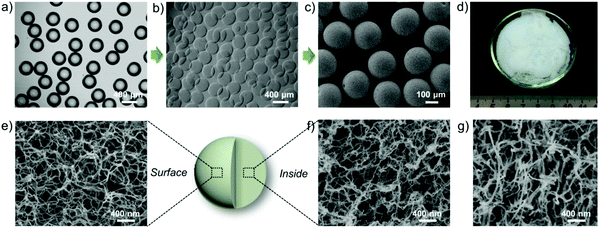 |
| Fig. 1 (a) Optical microscopy image of uniform CMS emulsions. (b) Optical microscopy image of uniform CMS microgels after solidified by NaOH. (c) SEM image of uniform CMS after scCO2 drying. (d) Digital photograph shows the white products (CMS) after scCO2 drying. (e, f) SEM images show the typical nanofibrous microstructure of the CMS. (g) SEM image reveals the natural ECM of cartilage from a pig's knee, which consist of nanofiber network. | |
As a powerful manufacturing technique for the preparation of uniform micro-particles,36–43 this microfluidic technology is also convenient for us to prepare NCSM with different sizes. By coordinating the diameter of the capillaries or regulating the flow rates of the outer oil and inner CS phases, CS emulsions with tunable sizes can be obtained (ESI Fig. S1 and Table S1†). The tunability of particle size facilitates us to further study the influence of the component size on cell adhesion, proliferation and even bottom-up TE tissue regeneration, which would then provide a reference for selecting the most appropriate size for an actual case of tissue repair. ESI Fig. S1† shows that NCMS with four different sizes were obtained. Notably, the sizes of all the NCMS decreased by approximately 20% after scCO2 drying, but they were restored to their original size when dispersed into water again (ESI Fig. S1†), which is attributed to the swelling behavior of the CMS aerogel.49,50
To further study the formation mechanism of the nanofibrous network, the relationship between the concentration of the neutralizing agent (NaOH) and the ultimate microstructure was investigated. Interestingly, the NaOH concentration was found to be closely related to the morphology of the product (Fig. 2a–d, ESI Fig. S2a–d†), which demonstrates that the diameter of the nanofibers in NCMS increased with the increasing NaOH concentration. This might be explained by combining the two mechanisms proposed by Domard et al.35 and Zhang et al.,24 respectively. Both the mechanisms were about self-assembly of polymer chains in physical processes. According to them, the CS chains were more rapidly and completely neutralized at high NaOH concentrations, leading to a high physical cross-linking density.35 Thus, rearrangement and entanglement of the CS chains were improved, favoring the self-aggregation of more CS chains through hydrogen bonding and hydrophobic interactions and ultimate formation of bigger nanofibers.24 We also found that an increase in the concentration of the CS solution resulted in an increase of the nanofiber density of the NCMS, but it appeared to have a weak influence on the diameter of the nanofibers (Fig. 2e–h). Remarkably, collapsed CSMs were obtained when the CS concentration was too low (ESI Fig. S2e and f†), which is explained by the fact that if the initial CS concentration is below the critical concentration for CS chain entanglement the formed nanofiber network would not be dense enough to support itself. On the other hand, a high CS concentration would not allow the formation of uniform CS emulsions due to the high viscosity of the CS solution (ESI Fig. S2h†). Based on these results, NCMS made of 10 mg ml−1 CS solution and neutralized with 0.3 M NaOH solution was selected as the optimal condition for further study. Furthermore, the specific BET surface area was determined to be approximately 339.7 ± 68.6 m2 g−1 (ESI Fig. S3†). Owing to the ECM-mimicking nanofibrous microstructure of the NCMS and the appealing intrinsic properties of CS, NCMS should be a promising candidate for cartilage regeneration. Thus, we first evaluated the ability of NCMS as cell-carriers for in vitro chondrocyte attachment and their biocompatibility. NCMS with different surface topographies and sizes were then investigated to compare their cell seeding efficiency and proliferation ability for chondrocytes. When culturing chondrocytes with NCMS, the chondrocytes were found to readily attach to the NCMS. As depicted in Fig. 3a, chondrocytes readily attached to the three types of nanofibrous CMS, and over 90% of the chondrocytes were attached to all of three types of NCMS 6 hours after cell seeding, whereas less than 45% of the chondrocytes were attached to the solid CMS without an ECM-mimicking structure. This enhanced attachment efficiency should be attributed to the ECM-mimicking nanofibrous structure of the NCMS, which provide more effective anchoring sites for cell attachment and proliferation than the solid CMS.23 In addition, a decrease in the seeding efficiency corresponding to an increase in the NCMS size was revealed (Fig. 3b), which might attribute to the higher specific surface area of the smaller NCMS. Cell proliferation test showed that almost no difference was observed for these three types of NCMS, but chondrocytes cultured with the nanofibrous CMS demonstrated significantly higher proliferation rates than that of the solid CMS (Fig. 3c). With respect to NCMS with different sizes, the proliferation rate of chondrocytes showed no visible difference (Fig. 3d). As shown in Fig. 3e, the NCMS are covered with a large number of round-shaped chondrocytes after 7 days of culture, whereas only a few chondrocytes were spread isolated on the surface of solid CMS with a smooth surface (Fig. 3f, ESI Fig. S4†). DAPI/phalloidin staining revealed the nuclei and cytoskeletons of the chondrocytes adhered to the individual NCMS (Fig. 3g–j), demonstrating that the chondrocytes adhered and spread over the whole surface of the NCMS after 7 days of agitated culture. The results indicate a good biocompatibility of NCMS and a rapid cell proliferation rate in contrast to that of the solid CMS.
 |
| Fig. 2 (a–c) SEM images show the surface of the NCMS (made of 10 mg ml−1 CS solution) collected with 0.15 M (a), 0.30 (b), and 0.6 M (c) NaOH solution, respectively. (d) Statistical fiber diameters of NCMS collected with different NaOH concentrations. (e–g) SEM images show the surface of NCMS (collected by 0.3 M NaOH solution) made from 5 (e), 10 (f), and 20 (g) mg ml−1 CS solution, respectively. (h) Statistical fiber diameters of NCMS made of different CS concentrations. Scale bar is 200 nm. | |
 |
| Fig. 3 (a, b) Seeding efficiency of chondrocytes on CMS with different surface topographies (a) and sizes (b). (c, d) Proliferation of chondrocytes on the surface of CMS with different surface topographies (c) and sizes (d). (e) SEM image shows the typical rounded chondrocytes on the surface of the CMS with nanofibrous surface. (f) SEM image shows only a few chondrocytes on the surface of the solid CMS with smooth surface. (g–i) Fluorescent confocal microscopy images show the nucleus (g), cytoskeleton (h) and merged (i) images of chondrocyte adhesion on the surface of the NCMS after culture for 7 days under agitated (60 rpm) culture conditions. F-actin was stained with FITC-conjugated phalloidin (green), and the cell nuclei were stained with DAPI (blue). (j) 3D reconstructed confocal image shows the chondrocytes on the surface of the NCMS. Cytoskeletons were stained with phalloidin-rhodamine (red), and cell nuclei were stained with DAPI (blue). | |
The potential of the NCMS as cell-carrier building blocks for the in vitro bottom-up construction of a larger-sized 3D geometrically cartilage-like composite was then studied. Because the sizes of the most commonly used microspheres as cell-carriers for bottom-up TE are about 100–500 μm,42 the NCMS with sizes ranging from 165 μm to 425 μm were investigated here. It was found that chondrocytes cultured with smaller NCMS were more easily formed into cell-NCMS aggregates. As shown in Fig. 4a, macroscopic millimeter-sized aggregates were easily formed for the NCMS-1 (165 μm) group after seeding for 7 days even under agitated culture conditions. Cytoskeleton and nucleus staining displays that a high density of chondrocytes was uniformly distributed across the whole 3D aggregate (Fig. 4a). A high magnification laser scanning confocal microscopy image clearly reveals that the chondrocytes maintain good vitality and are situated in a 3D spatially organized cell community (Fig. 4b). Fig. 4c and d demonstrate distinct cell–material interactions, and the NCMS were tightly bridged by the chondrocytes together with the synthesized ECM-like structure. Furthermore, the total contents of the cartilage-specific ECM (GAG) and the DNA of the chondrocytes in these four NCMS groups were quantified at various times. As demonstrated in Fig. 4e–g, the GAG/ww, DNA/ww and the GAG/DNA ratios in the smaller NCMS groups were higher than those in the larger NCMS groups. This result demonstrates that chondrocytes co-cultured with these NCMS, especially the smaller ones, were continuously producing GAG-positive ECM-like structure at high levels, indicating that the ECM-mimicking nanofibrous architecture of the NCMS favours the proliferation of the chondrocytes. It can be recognized that the produced ECM-like structure would help to bridge the micro-components and promote the formation of aggregated cell-NCMS composite.
 |
| Fig. 4 (a) Fluorescent confocal microscopy image shows the in vitro cell-NCMS-1 aggregate after agitated culturing for 7 days. (b) High magnification fluorescent confocal microscopy image reveals the 3D cell morphology in the aggregate. (c) High magnification fluorescent confocal microscopy image shows dense chondrocytes bridging two NCMS. (d) SEM image shows that dense chondrocytes with round shape are bridging among several NCMS. (e–g) GAG content (d), DNA content (e) and GAG/DNA ratio (f) of chondrocytes adhered on the surface of the NCMS after culturing for various times. (h) Digital photograph shows a typical microscopic in vitro cartilage-like composite. (i, j) HE staining (i) and safranin-O staining (j) show the cross section of a cartilage-like composite. (k) Cyclic stress–strain curves of a cartilage-like composite at a maximum strain of 50%. (l) SEM image shows the surface of a cartilage-like composite. (m) SEM images of the inside of a cartilage-like composite. The cartilage-like composite in (a–f) are all constructed with NCMS-3 (325 μm). | |
After 7 days of agitated incubation the NCMS–cells mixtures were transferred into molds with the shape of a 5 mm × 2 mm cake and further in vitro static incubation was conducted. Two weeks later, macroscopic semitransparent 3D cake-like composites constructed by NCMS with four different sizes (165–425 μm) were obtained and no difference was observed (Fig. 4h and 5a–d). DAPI and hematoxylin & eosin (HE) staining reveal that the chondrocytes are distributed among the NCMS in the whole composite (Fig. 4i and Fig. 5i–l†). Furthermore, safranin-O staining indicates that the spaces among the NCMS are filled with a cartilage-specific ECM-like structure (GAG) (Fig. 4j). Remarkably, the cartilage-like composite can even withstand a mechanical compression process without collapsing at a maximum strain of 50% after 10 compression cycles and displays a certain degree of elasticity, indicating that the scattered NCMS were tightly bonded together by the chondrocytes and the secreted ECM (Fig. 4k). After freeze drying, we can see a typical nanofibrous ECM-like network structure at the surface of the composite (Fig. 4l). As depicted in Fig. 4m, chondrocytes together with the nanofibrous ECM-like network are tightly connected with NCMS filling the space among NCMS. The nanofibrous structure of the secreted ECM demonstrates great similarity to that of our NCMS.
 |
| Fig. 5 (a–d) Digital photographs show the semitransparent cartilage-like composite constructed with NCMS with four different sizes (165–425 μm). (e–h) Bright field microscopy images show the dense cartilage-like composite consisted of chondrocytes and NCMS with different sizes. (i–l) Fluorescent microscopy images by DAPI staining show that chondrocytes was uniformly distributed across the whole cartilage tissue constructs. Scale bar for (a–d) is 2 mm, (e–h) is 400 μm and (i–l) is 500 μm. | |
Conclusions
In conclusion, chitosan microspheres with an ECM-mimicking nanofibrous structure were fabricated for the first time based on a physical crosslinking process, in which chitosan chains were induced to self-assembled into a 3D nanofiber network without using any potentially toxic or denaturizing chemical crosslinking agent. Uniform NCMS with tunable sizes and a controllable microstructure can also be obtained. A systematic study on the in vitro chondrocyte culture revealed that enhanced cell attachment, and proliferation were realized, and a macroscopic 3D geometrically shaped cartilage-like composite with a certain degree of mechanical elasticity can be easily constructed with the NCMS as the bottom-up cell-carrier building blocks. Despite smaller NCMS demonstrate some advantages, such as a bigger surface area for anchoring more cells, their lower yields due to the preparation technics should also be taken into account. Particularly, the biodegradation of chitosan has been systematically studied and had been proved that it is degraded mainly by lysozyme, which exists in various human body fluids and tissues.28,51,52 Therefore, NCMS should provide us with promising bottom-up tissue engineering candidates and possess significant application potential for further in vivo regeneration of cartilage or other tissue defects in tissue engineering and regenerative medicine.
Acknowledgements
We acknowledge the funding support from the National Natural Science Foundation of China (Grants 21431006, 91227103, 31370983), the Foundation for Innovative Research Groups of the National Natural Science Foundation of China (Grant 21521001), the National Basic Research Program of China (Grants 2014CB931800, 2013CB933900), and Scientific Research Grant of Hefei Science Center of Chinese Academy of Sciences (Grant 2015SRG-HSC038), Anhui Province Funds for Distinguished Young Scientists (1508085J08), and the Young Top-Notch Talent Support Scheme from Anhui Medical University.
Notes and references
- R. J. Wade and J. A. Burdick, Mater. Today, 2012, 15, 454 CrossRef CAS.
- M. M. Stevens and J. H. George, Science, 2005, 310, 1135 CrossRef CAS PubMed.
- L. G. Griffith and M. A. Swartz, Nat. Rev. Mol. Cell Biol., 2006, 7, 211 CrossRef CAS PubMed.
- F. Rosso, A. Giordano, M. Barbarisi and A. Barbarisi, J. Cell. Physiol., 2004, 199, 174 CrossRef CAS PubMed.
- J. E. Meredith, B. Fazeli and M. A. Schwartz, Mol. Biol. Cell, 1993, 4, 953 CrossRef CAS PubMed.
- D. Gullberg and P. Ekblom, Int. J. Dev. Biol., 1995, 39, 845 CAS.
- B. Z. Tian, J. Liu, T. Dvir, L. H. Jin, J. H. Tsui, Q. Qing, Z. G. Suo, R. Langer, D. S. Kohane and C. M. Lieber, Nat. Mater., 2012, 11, 986 CrossRef CAS PubMed.
- J. K. F. Suh and H. W. T. Matthew, Biomaterials, 2000, 21, 2589 CrossRef CAS PubMed.
- Z. Y. Tang, Y. Wang, P. Podsiadlo and N. A. Kotov, Adv. Mater., 2006, 18, 3203 CrossRef CAS.
- J. M. Holzwarth and P. X. Ma, Biomaterials, 2011, 32, 9622 CrossRef CAS PubMed.
- J. Berger, M. Reist, J. M. Mayer, O. Felt and R. Gurny, Eur. J. Pharm. Biopharm., 2004, 57, 35 CrossRef CAS PubMed.
- L. A. Smith, X. Liu, J. Hu and P. X. Ma, Biomaterials, 2010, 31, 5526 CrossRef CAS PubMed.
- S. Shah, P. T. Yin, T. M. Uehara, S. T. Chueng, L. Yang and K. B. Lee, Adv. Mater., 2014, 26, 3673 CrossRef CAS PubMed.
- R. J. Wade and J. A. Burdick, Nano Today, 2014, 9, 722 CrossRef CAS.
- T. G. Kim, H. Shin and D. W. Lim, Adv. Funct. Mater., 2012, 22, 2446 CrossRef CAS.
- R. Langer and J. P. Vacanti, Science, 1993, 260, 920 CAS.
- J. S. Liu and Z. J. Gartner, Trends Cell Biol., 2012, 22, 683 CrossRef CAS PubMed.
- D. L. Elbert, Curr. Opin. Biotechnol., 2011, 22, 674 CrossRef CAS PubMed.
- O. F. Khan, D. N. Voice, B. M. Leung and M. V. Sefton, Adv. Healthcare Mater., 2015, 4, 113 CrossRef CAS PubMed.
- H. Onoe and S. Takeuchi, Drug Discovery Today, 2015, 20, 236 CrossRef CAS PubMed.
- Y. T. Matsunaga, Y. Morimoto and S. Takeuchi, Adv. Mater., 2011, 23, H90 CrossRef CAS PubMed.
- A. Leferink, D. Schipper, E. Arts, E. Vrij, N. Rivron, M. Karperien, K. Mittmann, C. van Blitterswijk, L. Moroni and R. Truckenmuller, Adv. Mater., 2014, 26, 2592 CrossRef CAS PubMed.
- X. H. Liu, X. B. Jin and P. X. Ma, Nat. Mater., 2011, 10, 398 CrossRef CAS PubMed.
- B. Duan, X. Zheng, Z. Xia, X. Fan, L. Guo, J. Liu, Y. Wang, Q. Ye and L. Zhang, Angew. Chem., Int. Ed., 2015, 54, 1 CrossRef.
- O. C. M. Chan, K. F. So and B. P. Chan, J. Controlled Release, 2008, 129, 135 CrossRef CAS PubMed.
- Z. P. Zhang, R. L. Marson, Z. S. Ge, S. C. Glotzer and P. X. Ma, Adv. Mater., 2015, 27, 3947 CrossRef CAS PubMed.
- I. Y. Kim, S. J. Seo, H. S. Moon, M. K. Yoo, I. Y. Park, B. C. Kim and C. S. Cho, Biotechnol. Adv., 2008, 26, 1 CrossRef CAS PubMed.
- A. Di Martino, M. Sittinger and M. V. Risbud, Biomaterials, 2005, 26, 5983 CrossRef CAS PubMed.
- R. A. Kosher, J. W. Lash and R. R. Minor, Dev. Biol., 1973, 35, 210 CrossRef CAS PubMed.
- K. Jiang, C. Xue, C. Arya, C. Shao, E. O. George, D. L. DeVoe and S. R. Raghavan, Small, 2011, 7, 2470 CAS.
- J. H. Xu, H. Zhao, W. J. Lan and G. S. Luo, Adv. Healthcare Mater., 2012, 1, 106 CrossRef CAS PubMed.
- J. H. Xu, S. W. Li, C. Tostado, W. J. Lan and G. S. Luo, Biomed. Microdevices, 2009, 11, 243 CrossRef CAS PubMed.
- C.-H. Yang, K.-S. Huang, P.-W. Lin and Y.-C. Lin, Sens. Actuators, B, 2007, 124, 510 CrossRef CAS.
- H. Tan, Q. Zhou, H. Qi, D. Zhu, X. Ma and D. Xiong, Macromol. Biosci., 2012, 12, 621 CrossRef CAS PubMed.
- S. Ladet, L. David and A. Domard, Nature, 2008, 452, 76 CrossRef CAS PubMed.
- W. Wang, M. J. Zhang and L. Y. Chu, Acc. Chem. Res., 2014, 47, 373 CrossRef CAS PubMed.
- Y. Zhao, H. Gu, Z. Xie, H. C. Shum, B. Wang and Z. Gu, J. Am. Chem. Soc., 2013, 135, 54 CrossRef CAS PubMed.
- J. Ma, Y. S. Hui, M. Zhang, Y. Yu, W. Wen and J. Qin, Small, 2013, 9, 497 CrossRef CAS PubMed.
- P. M. Valencia, O. C. Farokhzad, R. Karnik and R. Langer, Nat. Nanotechnol., 2012, 7, 623 CrossRef CAS PubMed.
- S. Seiffert, Angew. Chem., Int. Ed., 2013, 52, 11462 CrossRef CAS PubMed.
- S. H. Kim, J. G. Park, T. M. Choi, V. N. Manoharan and D. A. Weitz, Nat. Commun., 2014, 5, 3068 Search PubMed.
- S.-W. Choi, Y. Zhang, Y.-C. Yeh, A. Lake Wooten and Y. Xia, J. Mater. Chem., 2012, 22, 11442 RSC.
- S. S. Datta, A. Abbaspourrad, E. Amstad, J. Fan, S. H. Kim, M. Romanowsky, H. C. Shum, B. Sun, A. S. Utada, M. Windbergs, S. Zhou and D. A. Weitz, Adv. Mater., 2014, 26, 2205 CrossRef CAS PubMed.
- J. Cai, S. L. Liu, J. Feng, S. Kimura, M. Wada, S. Kuga and L. N. Zhang, Angew. Chem., Int. Ed., 2012, 51, 2076 CrossRef CAS PubMed.
- S. M. Jung, H. Y. Jung, M. S. Dresselhaus, Y. J. Jung and J. Kong, Sci. Rep., 2012, 2, 849 Search PubMed.
- C. A. Garcia-Gonzalez, M. C. Camino-Rey, M. Alnaief, C. Zetzl and I. Smirnova, J. Supercrit. Fluids, 2012, 66, 297 CrossRef CAS.
- A. S. Utada, E. Lorenceau, D. R. Link, P. D. Kaplan, H. A. Stone and D. A. Weitz, Science, 2005, 308, 537 CrossRef CAS PubMed.
- Y. Zhao, H. C. Shum, H. Chen, L. L. Adams, Z. Gu and D. A. Weitz, J. Am. Chem. Soc., 2011, 133, 8790 CrossRef CAS PubMed.
- X. Qu, A. Wirsen and A. C. Albertsson, Polymer, 2000, 41, 4589 CrossRef CAS.
- M. N. Khalid, F. Agnely, N. Yagoubi, J. L. Grossiord and G. Couarraze, Eur. J. Pharm. Sci., 2002, 15, 425 CrossRef CAS PubMed.
- S. Hirano, H. Tsuchida and N. Nagao, Biomaterials, 1989, 10, 574 CrossRef CAS PubMed.
- W. W. Thein-Han and R. D. K. Misra, Acta Biomater., 2009, 5, 1182 CrossRef CAS PubMed.
Footnotes |
† Electronic supplementary information (ESI) available: Additional figures and table. See DOI: 10.1039/c5nr06876b |
‡ These authors contributed equally. |
|
This journal is © The Royal Society of Chemistry 2016 |