DOI:
10.1039/C5NJ02579F
(Paper)
New J. Chem., 2016,
40, 330-339
A rhodamine embedded bio-compatible smart molecule mimicking a combinatorial logic circuit and ‘key-pad lock’ memory device for defending against information risk†
Received
(in Montpellier, France)
23rd September 2015
, Accepted 16th October 2015
First published on 23rd October 2015
Abstract
Organic molecules with the possibility of logic operations are highly useful building blocks for the development of molecule-based “intelligent” devices for information processing applications. We have designed herein a very simple bio-friendly chemosensor (LC) equipped with a rhodamine fluorophore moiety. This probe showed a chromo-fluorescence response profile for Al3+ but a colorimetric response for Cu2+ metal. The absorption responses of LC caused by these metal ions along with the “OFF–ON–OFF” fluorescence behavior of an LC–Al3+ complex towards EDTA were employed for the development of a three-input and one output combinatorial molecular system. Interactions of the mentioned metal ions with LC in controlled sequential experiments gave fluorescence responses, enabling us to fabricate a ‘key-pad-logic’ function. So, a single molecular system performing such multiple ‘Boolean’ operations not only simplifies the complexity of a chemical driven ‘Intelligence’ device but also enriches the security of such a device against information invasion due to the sequence controlled sensor–analyte interactions and may find potential applications in biocompatible molecular logic platforms.
Introduction
In recent years the development of fluorescent molecular sensors triggered by several input signals and their applications in computation and analytical devices is gaining more and more research interest.1 Their most striking feature is their ability to imitate the function of electronic logic gates and circuits2 which makes them suitable for cellular imaging,3 clever chemosensing4 and digital molecular tags.5 Since the first pioneering proposal of a molecular AND gate by de Silva and co-workers,2a a variety of molecule-based systems capable of acting as Boolean logic gates like AND, OR, NOT, INH, NOR, XNOR, XOR etc. have been realized with the prospect of creating more sophisticated and complex molecular circuits.2c,6 It has been argued that the limitations of conventional silicon based devices in miniaturization down to the nanoscale level could be addressed successfully in a wireless manner with the appropriate design of molecular operators capable of executing various complicated and integrated logic operations.
In addition, recent interest has focused on a new strategy that enables the high-throughput, multi-analyte sensing shown by a molecule to be used to fabricate a combinatorial device.7 The combination of independent molecular gates as basic logic operators could in principle allow one to obtain any combinatorial logic circuit programmed into a single molecular switch. Such molecular switches can be used in complex circuits in the design and development of molecular electronic and photonic devices for information processing, sensing, and computation.7 So, it is necessary to design a chemical system that can respond to multiple inputs in order to improve complexity within the entire circuit of a single molecular switch.
But the possibility to develop such complex molecular logic systems along with the concept of constructing a sequence dependent memory device-like key-pad logic function within a single molecular system is more appealing and at the same time very challenging.8–10 Among these various memory devices, molecular keypad systems have drawn more attention; as these systems are based on a sequence of a correct combination of chemical inputs that signifies a more secure and classified password, providing considerable advantages over many other simple molecular logic gate devices.9 As we know, the logic circuit behind the keypad of a bank machine is of the sequential type that remembers a “three key-strokes history” as well as reading the fourth number in the PIN-code before generating the output response to authorize the user. Given the correct input sequence, the output of the keypad lock is switched to the ‘on-state’, thereby authorizing the user to initiate further processing, and strengthening security to defend the hardware of computing devices as well as the confidential data stored within it against information invasion during its execution and communication processing. Such sequential integration of molecular logic gates brings about the realization of information storage processes.
Due to a poor coordination ability and a lack of spectroscopic characteristics, the detection of Al3+ has always been a problematic task.11 The detection of Al3+ is of great interest due to its potential toxicity arising out of its widespread applications in automobiles, computers, packaging materials, electrical equipment, machinery, food additives and building construction.12,13 It is well known that 40% of soil acidity is due to aluminium toxicity.14
A number of analytical methods like chromatography,15 accelerator mass spectroscopy (AMS),16 graphite furnace atomic absorption spectrometry (GFAAS),17 neutron activation analysis (NAA),18 inductively coupled plasma-atomic emission spectrometry (ICP-AES),19 inductively coupled plasma-mass spectrometry (ICP-MS),20 laser ablation microprobe mass analysis (LAMMA),21 electrothermal atomic absorption spectrometry (ETAAS),22 fluorescence chemosensing etc. are available for the detection of Al3+; out of these the last one is the best choice as it is the simplest, it is a sensitive, fast and inexpensive technique23 offering significant advantages over other methods.24 Hence, recently the design and synthesis of Al3+ selective fluorescent probes has received intense attention from chemists.25–27 Compared to other metal ions, only a few fluorescent chemosensors have been reported for the detection of Al3+,28–33 and most of the sensors are insoluble in polar solvents and require complicated synthetic procedures. For practical applications, it is necessary to develop Al3+ sensors that are easily prepared and possess selective and sensitive signalling mechanisms.
We demonstrate herein a new simple bio-compatible chemosensor equipped with rhodamine to mimic the advanced arithmetic logic operation within a combinatorial logic circuit as well as the memory logical function by investigating absorbance as well as fluorescence “ON and OFF” states as outputs with controlled experiments based on sensor–analyte interactions at physiological conditions. Such a molecular ‘Boolean’ logic operation improves not only the complexity of logic gate circuitry but also can be used in potential biocompatible molecular logic platforms. More importantly, it may be applied to ensure information security against the illegal invasion of data with respect to its sequence dependent key-pad logic function.
Experimental section
Materials and methods
All solvents used for the synthesis of ligands were of reagent-grade (Merck) unless otherwise mentioned. For spectroscopic (UV/Vis and fluorescence) studies HPLC-grade MeOH and double-distilled water were used. Rhodamine 6G hydrochloride and metal salts such as the perchlorates of Na+, K+, Ca2+, Fe2+, Co2+, Ni2+, Zn2+, Pb2+, Cd2+, Hg2+ and Cu(NO3)2·2H2O were purchased from Sigma-Aldrich and used as received. All other compounds were purchased from commercial sources and used as received.
Physical measurements
Elemental analyses were carried out using a Perkin-Elmer 240 elemental analyzer. Infrared spectra (400–4000 cm−1) were recorded in the liquid state on a Nicolet Magna IR 750 series-II FTIR spectrometer. 1NMR spectra were recorded in CD3OD or DMSO-d6 on a Bruker 300 MHz NMR spectrometer using tetramethylsilane (δ = 0) as an internal standard. ESI-MS+ (m/z) of the ligand and Al3+-complex were recorded on a Waters' HRMS spectrometer (Model: XEVO G2QTof). UV-Vis spectra were recorded on an Agilent diode-array spectrophotometer (Model, Agilent 8453). Steady-state fluorescence measurements were performed with a PTI QM-40 spectrofluorimeter.
Syntheses and characterization
Preparation of N-(rhodamine-6G)lactam-ethylenediamine (LA).
LA was prepared according to a literature method.34
Preparation of 3-acetyl-4-hydroxy-6-methyl-2H-pyran-2-one35 (LB).
390 mmol of freshly vacuum distilled ethylacetoacetate and 0.3 g of sodium bicarbonate were heated until the liquid reached 200–210 °C. The time for heating was usually 7–8 h, during which period a bulk of distillate (mostly ethanol) was collected and the color of the reaction mixture became dark brown (Scheme 1). The resulting reaction mixture was distilled under reduced pressure (the desired crude product was collected at 140 °C – 12 mm). The crude product was re-crystallized using ethanol (yield 48%) for the further steps of the synthesis.351H-NMR in CDCl3: 2.26 (s, 3H), 2.66 (s, 3H), 5.93 (s, 1H) (Fig. S1, ESI†); 13C: 20.66, 30.02, 99.81, 101.39, 161.17, 169.08, 181.03, 205.20 (Fig. S2, ESI†); molecular formula (C8H8O4): C, 57.14; H, 4.80; found C, 57.12; H 4.79; ESI-MS+ (m/z): 169.13 (C8H8O4 + H+) (Fig. S3, ESI†).
 |
| Scheme 1 Methodology adopted for the synthesis of the target ligand (LC). | |
Preparation of the target molecule (LC).
0.5 mmol (0.228 g) of N-(rhodamine-6G)lactam-ethylenediamine in MeOH (10 mL) was added dropwise over a period of 30 min to a 10 mL methanolic solution of 3-acetyl-4-hydroxy-6-methyl-2H-pyran-2-one (LB) (0.55 mmol, 0.428 g) containing 2 drops of acetic acid under hot (ca. 50–60 °C) conditions. Then the reaction mixture was stirred under reflux for 2 h. An off-white precipitate was formed, which was collected by filtration after cooling the solution The residue was washed thoroughly with cold methanol/ether (1
:
1 v/v) to isolate LC in a pure form (78% yield). M.p. 214.4 °C. 1H NMR (CD3OD): δ = 1.28 (t, 6H), 1.82 (s, 6H), 2.12 (s, 3H), 2.29 (s, 3H), 3.26 (q, 4H), 3.29 (2H), 3.4 (t, 2H), 5.67 (s, 1H), 6.11 (s, 2H), 6.34 (s, 2H), 7.05 (m, 1H), 7.56 (m, 2H), 7.89 (m, 1H); (Fig. S4, ESI†). 13C-NMR: (DMSO-d6) δppm: 13.23, 15.55, 16.88, 18.14, 37.68, 38.35, 41.55, 65.65, 95.90, 96.15, 104.54, 106.96, 118.74, 122.39, 123.75, 127.65, 128.20, 130.51, 132.14, 148.17, 151.83, 153.54, 162.67, 134.62, 169.23, 176.59, 183.97 (Fig. S5, ESI†). CHN analysis for C36H38N4O5: calculated (%): C, 71.27; H, 6.31; N, 9.23. Found (%): C, 71.26; H, 6.32; N 9.24. ESI-MS+ (m/z): 607.328 (C36H38N4O5 + H+) (Fig. S6, ESI†). IR: 1688 cm−1 (spirolactam ring) (Fig. S7, ESI†).
Cell culture.
HepG2 (human hepatocellular liver carcinoma) and HCT116 (human colon cancer) cell lines from NCCS, Pune, India, were grown in DMEM supplemented with 10% FBS and antibiotics (penicillin – 100 μg mL−1; streptomycin – 50 μg mL−1) at 37 °C in 95% air, 5% CO2 incubator cells.
Cell cytotoxicity assay.
To determine % cell viability of LC, an MTT assay was performed. HepG2 and HCT116 cells (1 × 104 cells per well) were cultured in a 96-well plate at 37 °C, and exposed to varying concentrations of LC, 1, 10, 20, 50 and 100 μM, for 12 h. 20 μl of MTT solution [5 mg mL−1 in 1× phosphate-buffered saline (PBS)] was added to each well of a 96-well culture plate and incubated continuously at 37 °C for a period of 4 h. All media were removed from the wells and 100 μl of DMSO was added to each well and absorbance was measured at 550 nm (EMax Precision MicroPlate Reader, Molecular Devices, USA). All experiments were performed in triplicate and the relative cell viability (%) was expressed as a percentage relative to the untreated control cells.
Cell imaging study.
HepG2 and HCT116 cells were cultured and incubated in a 35 × 10 mm culture dish with a 10 μM solution of LC prepared by dissolving LC in mixed solvent (DMSO
:
water = 1
:
9 (v/v)) in the culture medium, which had been allowed to incubate for 30 min at 37 °C. After incubation, cells were washed twice with 1× PBS. Bright field and fluorescence images of HepG2 and HCT116 cells were taken using a fluorescence microscope (Leica DM3000, Germany) with an objective lens of 20× magnification. Fluorescence images of HepG2 and HCT116 cells were taken separately from another set of experiments where cells were incubated with 10 μM LC + 10 μM Al3+ for 30 min at 37 °C. Similarly, in another set of experiments, cells were incubated with 10 μM LC + 10 μM Al3+ for 30 min at 37 °C and fluorescence images were taken. HepG2 and HCT116 cells showed almost equal fluorescence intensities with LC forming a complex with Al3+.
Results and discussion
General features
Owing to the interesting photophysical properties of the rhodamine moiety, it is an attractive choice for the design of a receptor platform for various metal ions.34 Also, the various chemical output (absorbance or fluorescence) signals on such a platform may provide a good opportunity to fabricate interesting ‘Boolean’ logic operations at physiological conditions. The recent development of a multiple logic gate function on a single rhodamine based platform confirms this opinion.7a,b But this field is not well explored at an advanced level for the rhodamine system. All of the logical arithmetic developed from rhodamine derivatives is devoid of any concept related to memory devices with a combinatorial logic circuit. However, we have synthesized the target probe by a simple Schiff's condensation reaction between N-(rhodamine-6G)lactam-ethylenediamine and 3-acetyl-4-hydroxy-6-methyl-2H-pyran-2-one in methanolic conditions and thoroughly characterized it by NMR and ESI+-mass spectrometry analysis.
Spectroscopic interaction of metal ions with LC
The electronic absorption and emission properties of LC interacting with several metal ions [Mn+ = Na+, K+, Ca2+, Cr3+, Ag+, Pb2+, Mn2+, Fe2+, Fe3+, Co2+, Ni2+, Cu2+ and Zn2+, Cd2+, Hg2+ and Al3+] were investigated in MeOH
:
buffer (7
:
3, v/v HEPES buffer at pH 7) at 25 °C and the corresponding spectral changes of LC upon the addition of 5 equiv. of each metal ion are illustrated in Fig. 1. It is revealed that on addition of Al3+ and Cu2+ ions to a solution of the ligand there occurs a significant change in absorption spectra along with the development of an absorption band at ∼525 nm, clearly demonstrating the formation of the ring-opened amide form of LC upon binding to Al3+ or Cu2+ ions (Fig. 1A and Fig. S8, ESI†).13 No such change is observable by the addition of other metal ions. The steady-state fluorescence studies, under identical reaction conditions, reveal that upon excitation at 505 nm the free ligand itself is very weakly or non-fluorescent, while in the presence of Al3+ there is a significant fluorescence enhancement (FE) at 553 nm due to an Al3+ induced opening of the spirolactam ring of LC.36 No such prominent FE takes place for other metal ions including Cu2+ (Fig. 1B and Fig. S9, ESI†). In the case of Cu2+ the opening of the spirolactam ring of LC does occur and is expected to be fluorescent too; but the paramagnetic (d9) Cu2+ fully quenches the fluorescence making the system silent toward emission.37 Hence the above observations clearly demonstrate that the present probe, LC has the ability to detect oxophilic Al3+ both chromo/fluorometrically and Cu2+ ions only colorimetrically.
 |
| Fig. 1 (A) UV-Vis spectral studies and (B) fluorescence studies of the selective binding of LC (20 μM) toward Al3+ over other metal ions in HEPES buffer at pH 7.0 in H2O–MeOH = 3 : 7 (v/v) at 25 °C. | |
Spectroscopic determination of the stoichiometry and binding constants of LC–Al3+ and LC–Cu2+
Looking at the strong response towards the change in absorption properties of LC through Al3+ and Cu2+ chelation induced opening of the spirolactam ring, we were interested to find out their binding stoichiometries and corresponding binding constants. The composition of each of the metal complexes was determined by Job's method (Fig. 2A(b) and B(b)) and it was found to be 1
:
1 for both the complexes which was further confirmed by mass spectrometric analysis. ESI-MS+ (m/z): 632.59 [Al(C36H37N4O5)]+ and 650.27 [Al(C36H37N4O5)(H2O)]+ for Al3+ and 669.27 [Cu(C36H37N4O5) + H]+ for Cu2+ (Fig. S10 and S11, ESI†).
 |
| Fig. 2 (A) (a) Change in the absorption spectra of LC (20 μM) upon the addition of [Al3+] = 0–100 μM, (b) the 1 : 1 binding stoichiometry shown by Job's plot, (c) the B–H plot of absorbance (at 525 nm) vs. [Al3+] for the corresponding UV-Visible titration. (B) (a) Change in the absorption spectra of LC (20 μM) upon the addition of [Cu2+] = 0–100 μM, (b) the 1 : 1 binding stoichiometry shown by Job's plot, (c) the B–H plot of absorbance (at 525 nm) vs. [Cu2+] for the corresponding UV-Vis titration. Conditions: HEPES buffer at pH 7.0 in H2O–MeOH = 3 : 7 (v/v) at 25 °C. | |
UV titrations for the interaction between the receptor LC and Al3+ or Cu2+ in MeOH–aqueous buffer solution (7
:
3 v/v, HEPES at pH 7) at 25 °C for the complexation process were done in order to find out the stability constants. These were evaluated by the linear least-square fitting of the absorption data at 525 nm to the well known Benesi–Hildebrand equation (1).
|  | (1) |
where,
A0 and
Amax are the absorbances of the pure ligand and the ligand in the presence of excess metal ions, respectively. The evaluated parameters are
Kd = (5.8 ± 0.21) × 10
−6 M and
n = 1 for Al
3+ and
Kd = (5.53 ± 0.13) × 10
−6 M and
n = 1 for Cu
2+.
Similarly, the fluorescence titration (Fig. 3a) of LC (10 μM) against variable Al3+ concentrations (0–50 μM) in MeOH–aqueous buffer solution (7
:
3 v/v, HEPES at pH 7) at 25 °C gives Kd = (6.07 ± 0.12) × 10−6 M for Al3+ by fitting the corresponding fluorescent intensity at 553 nm to the well known Benesi–Hildebrand equation (n = 1) (Fig. 3b). There is an excellent agreement between the Kd values for the LC–Al3+ system obtained from absorption and fluorescence titration data and this clearly manifests itself in the self-consistency of our results. It looked quite interesting that the association constants of the LC–Al3+ system were almost similar to that of the LC–Cu2+ system determined by absorption titrations.
 |
| Fig. 3 (a) Change in the emission spectra of LC (10 μM) upon the addition of Al3+ in HEPES buffer at pH 7.0 in H2O–MeOH = 3 : 7 (v/v) at 25 °C, [Al3+] = 0–50 μM; (b) B–H plot of fluorescence (at 553 nm) vs. [Al3+] for the corresponding emission titration. (c) Fluorescence intensity vs. pH plot at 553 nm LC (10 μM, denoted by black mark) and LC–Al3+ complex (denoted by red mark). | |
Effect of pH and reversibility study
To study the practical applicability of the probe, the effect of pH on the fluorescence response of LC towards Al3+ was investigated. Experimental results show that the free LC is highly fluorescent at pH ≤ 2 which arises due to the opening of the spirolactam ring by strong protonation on the secondary amine-N atom (Fig. 3c). On a gradual increase in pH of the medium the fluorescence intensity decreases gradually, reaches a minimum at pH ∼ 6.0 and then remains almost constant up to pH 12.0. However, in the presence of Al3+, there was an obvious fluorescence switch ON of LC at pH 5.0 to 9.0 indicating the fact that the probe LC can work well at physiological pH conditions. Above pH ≥ 9.0 the fluorescence intensity falls rapidly and becomes constant at pH ≥ 11.0 and this arises mainly due to the formation of [Al(OH)3], which comes out of the LC–Al3+ complex and re-establishes the non-fluorescent spirolactam ring.
We subsequently studied the chemical reversibility behaviour of the binding of LC and Al3+ in methanol–water solution. With the addition of EDTA to the LC–Al3+ complex, release of Al3+ from this complex took place resulting in the bleaching of the absorption band at 525 nm and the emission band at around 553 nm. The corresponding LC–Cu2+ complex also showed reversibility in the presence of EDTA (Fig. S12 and S13, ESI†). This reversibility assay indicates the reusability of the chemo-sensor LC.38
Evidence in support of the spirolactam ring opening mechanism
The mechanistic pathway for the reaction of LC with Al3+via the opening of the spirolactam ring has been well established through 1H, 13C-NMR and IR studies.39 IR studies revealed that the characteristic stretching frequency for the ‘C
O’ in the amide group of the rhodamine moiety at 1688 cm−1 was shifted to a lower wave number of 1612 cm−1 in the presence of Al3+ (Fig. S14, ESI†). This larger shift (Δν = 66 cm−1) towards a lower wave number signifies a higher polarization of the C
O bond upon efficient binding to the Al3+ ion, indicating the cleavage of the above mentioned bond. Also in 1H NMR (CD3OD), the ring protons (i and j; see the labelling in Fig. S15, ESI†) of the rhodamine part moved in the downfield direction (Δδi = 0.717 ppm, Δδj = 0.638 ppm) in the presence of Al3+ ions. Also in 13C-NMR (DMSO-d6) the disappearance of the signal at 65.65 ppm for the tertiary carbon (sp3 hybridized) of the spirolactam ring of LC (labelled with a star mark, see Fig. S6 and S16, ESI†) upon addition of Al3+ convincingly supports the opening of the spirolactam ring and coordination through the O atom of the –CONH group of the rhodamine moiety with an Al3+ ion.40 Due to the paramagnetic nature of Cu2+, it was not included for NMR studies.37d
Demonstration of various ‘Boolean’ logic operations
Based on various spectroscopic observations like the chromo-fluorogenic dual signalling response of Al3+ and the colorimetric response of the chemosensor LC towards Cu2+ along with the very close Kd values of LC–Al3+ and LC–Cu2+ complexes it will be quite interesting to see what will happen if they co-exist in a system to coordinate with LC. So, investigating the different absorbance and fluorescence “ON and OFF” states of LC through controlled experiments some interesting chemistry related to mimicking advanced logic operations and various output information is noted, which could be interpreted within the framework of various molecular logic circuits.41 In order to correlate various arithmetic logic operations, we arbitrarily choose threshold values of 0.20 for absorbance and 2 × 106 for fluorescence intensity. Any spectral values of outputs lower than the respective threshold limits are taken as 0 (OFF-state) while those greater than the threshold limit are taken as 1 (ON-state).
Using Cu2+ (In 1), Al3+ (In 2) and EDTA (In 3) as inputs, an integrated logic circuit is constructed in the LC system (Fig. 4). In the presence of both inputs Cu2+ and Al3+, the output at 525 nm is 1 (OD > 0.20); while in the presence of EDTA the resultant output is always 0 (OD < 0.2). Hence, this combinational complex logic circuit incorporates an AND gate that integrates an OR and a NOT gate. Fig. 5 illustrates the corresponding truth table and logic circuit.
 |
| Fig. 4 (A) UV-Vis spectra of LC (in CH3OH : H2O 7 : 3; pH 7.0) under different input conditions. (B) Corresponding bar diagram at 525 nm in the presence of different input sequences. | |
 |
| Fig. 5 A three-input, one-output molecular switch representation of the absorbance of LC based on Fig. 4. (A) Truth table. (B) Combinatorial logic scheme. | |
As the binding constants of the LC–Al3+ and LC–Cu2+ systems are comparable, so the introduction of the first input will be dominant over the second one. Based on this idea a ‘Keypad lock’ for device security can be constructed in a sequential logical manner.9a In the absence of any chemical input, the receptor LC shows no prominent emission band at 553 nm, i.e. output is 0 (OFF-state). Now, for the first input sequence, Al3+ and Cu2+ were used as inputs A (In A) and B (In B), respectively. Operation by Al3+ as (In A) to receptor LC, gives the output “1” (ON-state) and with the sole sequential addition of another input Cu2+ (In B), the output still remained ‘1’ (ON-state), i.e. for both cases fluorescence intensity crosses the threshold limit (fluorescence intensity > 2 × 106, output 1). But, on reversal of the input sequence, i.e. for Cu2+ as the first input followed by Al3+, it causes the fluorescence intensity to be far below its threshold limit and the corresponding output is taken as zero. On the basis of the above observations (Fig. 6A–D) our system can be used as a confidential password entry for a keypad lock; inputs Al3+ and Cu2+ were designated as ‘N’ and ‘E’ respectively. Out of two possibilities of inputs: (a) first addition of ‘N’ followed by ‘E’ where the receptor (LC) showed emission ‘ON’ at 553 nm and this “switch ON” is represented by ‘W’ creating a secret code ‘NEW’. While on the other hand, by reversing the order of inputs, i.e. the first input ‘E’ followed by ‘N’, the fluorescence at 553 nm remained switched “OFF” and is represented by ‘N’, which is the wrong entry (END), and failed to open the keypad lock; i.e. a three-digit identification number is obtained by the sequence controlled sensor–analyte interaction with fluorescence. Thus, a sequence dependence of inputs is mandatory for the construction of the molecular keypad lock, i.e. ‘NEW’ only (Fig. 7B). It is due to the fact that the use of numerical digits (0–9) and letters (A–Z) as ‘PIN’ numbers in a two-digit password allows a total of more than 700 different combinations,9d this adds to the complexity of cracking the keypad lock and improves remarkably the security of the molecular devices. Therefore, only an authorized user who knows the exact password can open the lock, which is a new approach for protecting information at the molecular scale.
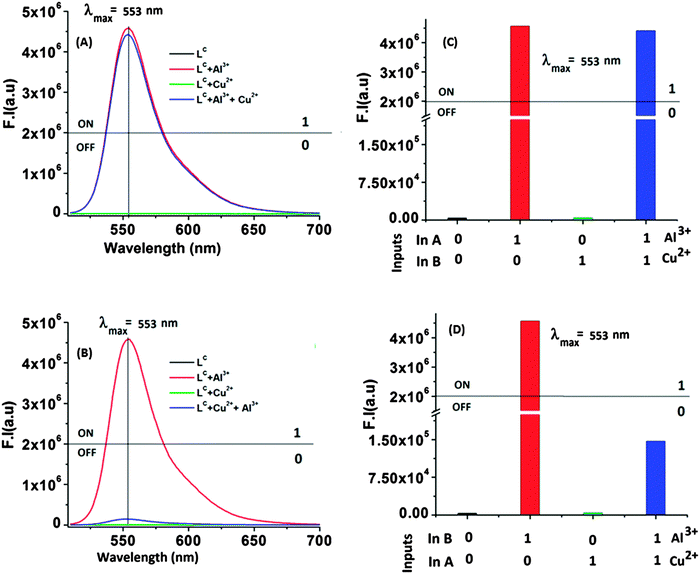 |
| Fig. 6 (A) Emission output of LC following excitation at 505 nm with different input sequences: (A) Al3+ as the first input A followed by Cu2+ as the second input B; (B) Cu2+ as the first input A followed by Al3+ as the second input B. The corresponding emission output bar diagram at 553 nm is shown in an inset: (C) In A (Al3+ first) and In B (Cu2+ second); (D) In B (Cu2+ first) and In A (Al3+ second). The threshold limit (fluorescence intensity = 2 × 106 nm) was taken for the concerned logic operation. | |
 |
| Fig. 7 (A) Combined truth table based on Fig. 6 for key-pad logic operation. (B) Sequence-dependent crossword puzzle with the right code. | |
Cell imaging experiments
To determine whether LC has any cytotoxic effect, an MTT assay42 was performed to check its viability on HepG2 and HCT116 cells. No significant cell cytotoxicity was observed for both HepG2 and HCT116 cells with up to 100 μM of LC for 24 hours (Fig. S17, ESI†). So, this probe is found to be suitable for bioimaging of intracellular Al3+ ions. The intracellular imaging behaviours of LC on HepG2 and HCT116 cells displayed no fluorescence on treating with 10 μM of LC only (Fig. 8). However, an excellent fluorescence was observed inside the cells, when HepG2 and HCT116 cells were incubated with 10 μM of LC followed by 10 μM of Al3+. This suggests that LC with a rhodamine fluorophore platform is useful for the intracellular detection of Al3+.
 |
| Fig. 8 The phase contrast and fluorescence images of HepG2 and HCT116 cells were captured after incubation with LC or LC + Al3+ for 30 min at 37 °C. HepG2 and HCT116 cells showed almost equal fluorescence intensities with LC forming a complex with Al3+. | |
Conclusion
We have designed, and easily synthesized herein, a very simple bio-compatible chemosensor LC bearing rhodamine as a signaling moiety. Based on the receptor–analyte interactions along with its chemosensing behaviour we have fabricated three inputs and one output, and a one output combinatorial molecular logic system with an absorption response. Also controlled sequential experiments with an emission response enabled us to fabricate a ‘key-pad-logic’ function. So, a single molecular system performing such interesting ‘Boolean’ operations not only improves the complexity of a chemical driven ‘Intelligence’ device but also enriches the security of such a device against information invasion through sequence controlled sensor–analyte-interactions. Its bio-compatible nature along with these logical functions may be used in constructing potential biocompatible molecular logic platforms too. Such kinds of ‘Lab-on-molecule’ for the development of a chemical driven smart molecular circuit is in progress in our laboratory.
Acknowledgements
Financial support from DST (Ref. SR/S1/IC-20/2012) and CSIR (Ref No. 01(2490)/11/EMR-II), New Delhi, India, are gratefully acknowledged. T. M. acknowledges the fellowship from UGC (UGC-NET).
References
-
(a) A. P. de Silva and S. Uchiyama, Nat. Nanotechnol., 2007, 2, 399 CrossRef CAS PubMed;
(b) H. Tian, Angew. Chem., 2010, 122, 4818 CrossRef;
(c) J. Andrasson and U. Pischel, Chem. Soc. Rev., 2010, 39, 174 RSC;
(d) K. Szacilowski, Chem. Rev., 2008, 108, 3481 CrossRef CAS PubMed;
(e) Y. V. Gerasimova and D. M. Kolpashchikov, Chem. – Asian J., 2012, 7, 534 CrossRef CAS PubMed;
(f) P. Ghosh, P. K. Bharadwaj, S. Mandal and S. Ghosh, J. Am. Chem. Soc., 1996, 118, 1553 CrossRef CAS;
(g) P. Singh, H. Singh, G. Bhargava and S. Kumar, J. Mater. Chem. C, 2015, 3, 5524 RSC.
-
(a) A. P. de Silva, H. Q. Gunaratne and C. P. A. NMcCoy, Nature, 1993, 364, 42 CrossRef;
(b) A. P. de Silva and N. D. McClenaghan, Chem. – Eur. J., 2002, 8, 4935 CrossRef PubMed;
(c) A. P. de Silva and N. D. McClenaghan, J. Am. Chem. Soc., 2000, 122, 3965 CrossRef CAS;
(d) A. Coskun, E. Deniz and E. U. Akkaya, Org. Lett., 2005, 7, 5187 CrossRef CAS PubMed;
(e) J. Andrasson, S. D. Straight, S. Bandyopadhyay, R. H. Mitchell, T. A. Moore, A. L. Moore and D. Gust, Angew. Chem., 2007, 119, 976 CrossRef;
(f) T. Mistri, R. Alam, M. Dolai, S. K. Mandal, P. Guha, A. R. Khuda-Bukhsh and M. Ali, Eur. J. Inorg. Chem., 2013, 5854 CrossRef CAS;
(g) Z. Dong, X. Le, P. Zhou, C. Dong and J. Ma, RSC Adv., 2014, 4, 18270 RSC;
(h) E. T. Ecik, A. Atilgan, R. Guliyev, T. B. Uyar, A. Gumus and E. U. Akkaya, Dalton Trans., 2014, 43, 67 RSC.
-
(a) Y. Benenson, B. Gil, U. Ben-Dor, R. Adar and E. Shapiro, Nature, 2004, 429, 423 CrossRef CAS PubMed;
(b) L. Yuan, W. Lin, Y. Xie, B. Chen and S. Zhu, J. Am. Chem. Soc., 2012, 134, 1305 CrossRef CAS PubMed.
-
(a) S. Uchiyama, K. Iwai and A. P. de Silva, Angew. Chem., 2008, 120, 4745 CrossRef;
(b) O. A. Bozdemir, R. Guliyev, O. Buyukcakir, S. Selcuk, S. Kolemen, G. Gulseren, T. Nalbantoglu, H. Boyaci and E. U. Akkaya, J. Am. Chem. Soc., 2010, 132, 8029 CrossRef CAS PubMed;
(c) Z. Guo, W. Zhu and H. Tian, Chem. Commun., 2012, 48, 6073 RSC;
(d) J. M. Fang, P. F. Gao, X. L. Hu and Y. F. Li, RSC Adv., 2014, 4, 37349 RSC.
- A. P. de Silva, M. R. James, B. O. F. McKinney, D. A. Pears and S. M. Weir, Nat. Mater., 2006, 5, 787 CrossRef PubMed.
-
(a) B. Valeur and I. Leray, Coord. Chem. Rev., 2000, 205, 3 CrossRef CAS;
(b) A. P. de Silva, Chem. – Asian J., 2011, 6, 750 CrossRef PubMed;
(c) K. S Hettie, J. L. Klockow and T. E. Glass, J. Am. Chem. Soc., 2014, 136, 4877 CrossRef PubMed;
(d) J. Andreasson, U. Pischel, S. D. Straight, T. A. Moore, A. L. Moore and D. Gust, J. Am. Chem. Soc., 2011, 133, 11641 CrossRef CAS PubMed.
-
(a) X. Wu, X. Zhou and J. Yoon, Chem. Commun., 2015, 51, 111 RSC;
(b) M. Yuan, W. Zhou, X. Liu, M. Zhu, J. Li, X. Yin, H. Zheng, Z. Zuo, C. Ouyang, H. Liu, Y. Li and D. Zhu, J. Org. Chem., 2008, 73, 13 Search PubMed;
(c) A.-F. Li, Y.-B. Ruan, Q.-Q. Jiang, W.-B. He and Y.-B. Jiang, Chem. – Eur. J., 2010, 16, 5794 CrossRef CAS PubMed;
(d) M. Yuan, W. Zhou, X. Liu, M. Zhu, J. Li, X. Yin, H. Zheng, Z. Zuo, C. Ouyang, H. Liu, Y. Li and D. Zhu, J. Org. Chem., 2008, 73, 13 Search PubMed;
(e) P. Mahato, S. Saha and A. Das, J. Phys. Chem. C, 2012, 116, 17448 CrossRef CAS;
(f) A. Dhir, V. Bhalla and M. Kumar, Org. Lett., 2008, 10, 4894 CrossRef PubMed;
(g) J.-M. Montenegro, E. Perez-Inestrosa, D. Collado, Y. Vida and R. Suau, Org. Lett., 2004, 6, 2354 CrossRef PubMed;
(h) V. Luxami and S. Kumar, Dalton Trans., 2012, 41, 4588 RSC;
(i) J. Andréasson and U. Pischel, Chem. Soc. Rev., 2015, 44, 1053 RSC;
(j) J. Ling, B. Daly, V. A. D. Silverson and A. P. de Silva, Chem. Commun., 2015, 51, 8403 RSC.
- U. Pischel, Angew. Chem., Int. Ed., 2010, 49, 1356 CrossRef CAS PubMed.
-
(a) D. Margulies, C. E. Felder, G. Melman and A. Shanzer, J. Am. Chem. Soc., 2007, 129, 347 CrossRef CAS PubMed;
(b) Z. Guo, W. Zhu, L. Shen and H. Tian, Angew. Chem., Int. Ed., 2007, 46, 5549 CrossRef CAS PubMed;
(c) J. Andréasson, S. D. Straight, T. A. Moore, A. L. Moore and D. Gust, Chem. – Eur. J., 2009, 15, 3936 CrossRef PubMed;
(d) M. Suresh, A. Ghosh and A. Das, Chem. Commun., 2008, 3906 RSC;
(e) M. Kumar, A. Dhir and V. Bhalla, Org. Lett., 2009, 11, 2567 CrossRef CAS PubMed;
(f) M. Kumar, R. Kumar and V. Bhalla, Chem. Commun., 2009, 7384 RSC;
(g) Y. Wang, Y. Huang, B. Li, L. Zhang, H. Song, H. Jiang and J. Gaom, RSC Adv., 2011, 1, 1294 RSC;
(h) G. Strack, M. Ornatska, M. Pita and E. Katz, J. Am. Chem. Soc., 2008, 130, 4234 CrossRef CAS PubMed;
(i) V. Bhalla, V. Vij, M. Kumar, P. R. Sharma and T. Kaur, Org. Lett., 2012, 14, 1013 Search PubMed;
(j) V. Bhalla, R. Kumar and M. Kumar, Org. Lett., 2012, 14, 2804 Search PubMed.
-
(a) G. de Ruiter, E. Tartakovsky, N. Oded and M. E. van der Boom, Angew. Chem., Int. Ed., 2010, 49, 169 CrossRef CAS PubMed;
(b) J. Andreasson, S. D. Straight, T. A. Moore, A. L. Moore and D. Gust, Chem. – Eur. J., 2009, 15, 3936 CrossRef CAS PubMed;
(c) M. Kumar, A. Dhir and V. Bhalla, Chem. Commun., 2010, 46, 6744 RSC;
(d) M. Kumar, R. Kumar and V. Bhalla, Org. Lett., 2011, 13, 366 CrossRef CAS PubMed.
- K. Soroka, R. S. Vithanage, D. A. Phillips, B. Walker and P. K. Dasgupta, Anal. Chem., 1987, 59, 629 CrossRef CAS.
-
J. R. Lakowicz, Topics in Fluorescence Spectroscopy: Probe Design and Chemical Sensing, Kluwer Academic Publishers, New York, 2002, vol. 4 Search PubMed.
- M. G. Soni, S. M. White, W. G. Flamm and G. A. Burdock, Regul. Toxicol. Pharmacol., 2001, 33, 66 CrossRef CAS PubMed.
-
(a) T. P. Flaten and M. Odegard, Food Chem. Toxicol., 1988, 26, 959 CrossRef CAS PubMed;
(b) J. Ren and H. Tian, Sensors, 2007, 7, 3166 CrossRef CAS;
(c) R. A. Yokel, Neurotoxicology, 2000, 21, 813 CAS.
-
(a) O. Y. Nadzhafova, S. V. Lagodzinskaya and V. V. Sukhan, J. Anal. Chem., 2001, 561, 78 Search PubMed;
(b) M. J. Ahmed and J. Hossan, Talanta, 1995, 42, 1135 CrossRef CAS PubMed;
(c) M. Ahmad and R. Narayanaswamy, Sens. Actuators, B, 2002, 81, 259 CrossRef CAS.
-
R. Flarend and D. Elmore, in Aluminum-26 as a biological tracer using accelerator mass spectrometry, ed. P. Zatta and A. C. Alfrey, Aluminum in Infant's Health and Nutrition, 5 World Scientific, Singapore, 1997, p. 16 Search PubMed.
- J. L. McDonald-Stephens and G. Taylor, Plant Physiol., 1995, 145, 327 CrossRef CAS.
- R. F. Fleming and R. M. Lindstrom, J. Radioanal. Nucl. Chem., 1987, 113, 35 CrossRef CAS.
- J. C. Rouchaud, N. Boisseau and M. Fedoroff, J. Radioanal. Nucl. Chem. Lett., 1993, 175, 25 CrossRef CAS.
- B. Rietz, K. Heydorn and G. Pritzl, J. Radioanal. Nucl. Chem., 1997, 216, 113 CrossRef CAS.
- M. A. Lovell, Ann. Neurol., 1993, 33, 36 CrossRef CAS PubMed.
- S. Knežević, R. Milačič and M. Veber, J. Anal. Chem., 1998, 362, 162 Search PubMed.
-
(a) J. W. Zhu, Y. Qin and Y. H Zhang, Anal. Chem., 2010, 82, 436 CrossRef CAS PubMed;
(b) T. Y. Han, X. Feng, B. Tong, J. B. Shi, L. Chen, J. G. Zhi and Y. P. Dong, Chem. Commun., 2012, 48, 416 RSC;
(c) S. Anbu, S. Shanmugaraju, R. Ravishankaran, A. A. Karande and P. S. Mukherjee, Dalton Trans., 2012, 41, 13330 RSC.
-
(a)
U. E. Spichiger-Keller, Chemical Sensors and Biosensors for Medical and Biological Applications, Wiley-VCH, Weinheim, Germany, 1998 Search PubMed;
(b) V. Amendola, L. Fabbrizzi, F. Forti, M. Licchelli, C. Mangano, P. Pallavicini, A. Poggi, D. Sacchi and A. Taglieti, Coord. Chem. Rev., 2006, 250, 273 CrossRef CAS;
(c) K. Rurack and U. Resch-Genger, Chem. Soc. Rev., 2002, 31, 116 RSC;
(d) P. T. Srinivasan, T. Viraraghavan and K. S. Subramanian, Water SA, 1999, 25, 47 CAS.
-
(a) M. Arduini and P. Tecilla, Chem. Commun., 2003, 1606 RSC;
(b) J. S. Kim and J. Vicens, Chem. Commun., 2009, 4791 RSC;
(c) S. Kim, J. Y. Noh, K. Y. Kim, J. H. Kim, H. K. Kang, S. W. Nam, S. H. Kim, S. Park, C. Kim and J. Kim, Inorg. Chem., 2012, 51, 3597 CrossRef CAS PubMed;
(d) F. K. Hau, X. M. He, W. H. Lam and V. W. Yam, Chem. Commun., 2011, 47, 8778 RSC;
(e) Y. Lu, S. S. Huang, Y. Y. Liu, S. He, L. C. Zhao and X. S. Zeng, Org. Lett., 2011, 13, 5274 CrossRef CAS PubMed;
(f) D. Maity and T. Govindaraju, Chem. Commun., 2010, 46, 4499 RSC;
(g) Y. Dong, J. F. Li, X. X. Jiang, F. Y. Song, Y. X. Cheng and C. J. Zhu, Org. Lett., 2011, 13, 2252 CrossRef CAS PubMed;
(h) L. Wang, W. Qin, X. Tang, W. Dou, W. Liu, Q. Teng and X. Yao, Org. Biomol. Chem., 2010, 8, 3751 RSC;
(i) A. Sahana, A. Banerjee, S. Das, S. Lohar, D. Karak, B. Sarkar, S. K. Mukhopadhyay, A. K. Mukherjee and D. Das, Org. Biomol. Chem., 2011, 9, 5523 RSC.
-
(a) W.-H. Ding, W. Cao, X.-J. Zheng, D.-C. Fang, W.-T. Wong and L.-P. Jin, Inorg. Chem., 2013, 52, 7320 CrossRef CAS PubMed;
(b) D. Maity and T. Govindaraju, Inorg. Chem., 2010, 49, 7229 CrossRef CAS PubMed;
(c) D. Maity and T. Govindaraju, Chem. Commun., 2010, 46, 4499 RSC;
(d) S. Sinha, R. R. Koner, S. Kumar, J. Mathew, P. V. Monisha, I. Kazia and S. Ghosh, RSC Adv., 2013, 3, 345 RSC.
-
(a) S. Kim, J. Y. Noh, K. Y. Kim, J. H. Kim, H. K. Kang, S.-W. Nam, S. H. Kim, S. Park, C. Kim and J. Kim, Inorg. Chem., 2012, 51, 3597 CrossRef CAS PubMed;
(b) C.-H. Chen, D.-J. Liao, C.-F. Wan and A.-T. Wu, Analyst, 2013, 138, 2527 RSC.
- S. H. Kim, H. S. Choi, J. Kim, S. J. Lee, D. T. Quang and J. S. Kim, Org. Lett., 2010, 12, 560 CrossRef CAS PubMed.
- M. Dong, Y. M. Dong, T. H. Ma, Y. W. Wang and Y. Peng, Inorg. Chim. Acta, 2012, 381, 137 CrossRef CAS.
- T. Han, X. Feng, B. Tong, J. Shi, L. Chen, J. Zhic and Y. Dong, Chem. Commun., 2012, 48, 416 RSC.
- X. Sun, Y. W. Wang and Y. Peng, Org. Lett., 2012, 14, 3420 CrossRef CAS PubMed.
- K. K. Upadhyay and A. Kumar, Org. Biomol. Chem., 2010, 8, 4892 CAS.
- J. Y. Jung, S. J. Han, J. Chun, C. Lee and J. Yoon, Dyes Pigm., 2012, 94, 423 CrossRef CAS.
- J.-S. Wu, I.-C. Hwang, K. S. Kim and J. S. Kim, Org. Lett., 2007, 9, 907 CrossRef CAS PubMed.
- J. N. Coolie, J. Chem. Soc., 1891, 59, 609 Search PubMed.
- X. Chen, T. Pradhan, F. Wang, J. S. Kim and J. Yoon, Chem. Rev., 2012, 112, 1910 CrossRef CAS PubMed.
-
(a) M. Suresh, A. Shrivastav, S. Mishra, E. Suresh and A. Das, Org. Lett., 2008, 10, 3013 CrossRef CAS PubMed;
(b) J. S. Kim and D. T. Quang, Chem. Rev., 2007, 107, 3780 CrossRef CAS PubMed;
(c) D. Maity, V. Kumar and T. Govindaraju, Org. Lett., 2012, 14, 6008 CrossRef CAS PubMed;
(d) R. Alam, T. Mistri, P. Mondal, D. Das, S. K. Mandal, A. R. Khuda-Bukhsh and M. Ali, Dalton Trans., 2014, 43, 2566 RSC.
-
(a) R. Bhowmick, M. Dolai, R. Alam, T. Mistri, A. Katarkar, K. Chaudhuri and M. Ali, RSC Adv., 2014, 4, 41784 RSC;
(b) Y. Fu, X.-J. Jiang, Y.-Y. Zhu, B.-J. Zhou, S.-Q. Zang, M.-S. Tang, T. C. W. Mak and H.-Y. Zhang, Dalton Trans., 2014, 43, 12624 RSC;
(c) T. Mistri, R. Alam, M. Dolai, S. K. Mandal, A. R. Khuda-Bukhsh and M. Ali, Org. Biomol. Chem., 2013, 11, 1563 RSC.
-
(a) S. Angupillai, J. Y. Hwang, J. Y. Lee, B. A. Rao and Y. A. Son, Sens. Actuators, B, 2015, 214, 101 CrossRef CAS;
(b) H. S. Kim, S. Angupillai and Y. A. Son, Sens. Actuators, B, 2016, 222, 447 CrossRef CAS;
(c) H. Yu, J. Y. Lee, S. Angupillai, S. Wang, S. Feng, S. Matsumoto and Y. A Son, Spectrochim. Acta, Part A, 2015, 151, 48 CrossRef CAS PubMed;
(d) H. Kim, B. A. Rao, J. W. Jeong, S. Mallick, S. M. Kang, J. S. Choi, C. S. Lee and Y. A. Son, Sens. Actuators, B, 2015, 210, 173 CrossRef CAS.
-
(a) B. Sen, M. Mukherjee, S. Banerjee, S. Pal and P. Chattopadhyay, Dalton Trans., 2015, 44, 8708 RSC;
(b) B. Sen, S. Pal, S. Banerjee, S. Lohar and P. Chattopadhyay, Eur. J. Inorg. Chem., 2015, 1383 CrossRef CAS.
- N. Sharma, S. I. Reja, V. Bhalla and M. Kumar, Dalton Trans., 2015, 44, 6062 RSC.
- J. Ratha, K. A. Majumdar, S. K. Mandal, R. Bera, C. Sarkar, B. Saha, C. Mandal, K. D. Saha and R. Bhadra, Mol. Cell. Biochem., 2006, 290, 113 CrossRef CAS PubMed.
Footnote |
† Electronic supplementary information (ESI) available: Additional detailed characterization of synthesized materials along with fluorescence and UV-Vis spectra. See DOI: 10.1039/c5nj02579f |
|
This journal is © The Royal Society of Chemistry and the Centre National de la Recherche Scientifique 2016 |