DOI:
10.1039/C6FO00635C
(Paper)
Food Funct., 2016,
7, 3458-3467
Malondialdehyde and 4-hydroxy-2-hexenal are formed during dynamic gastrointestinal in vitro digestion of cod liver oils
Received
2nd May 2016
, Accepted 27th June 2016
First published on 30th June 2016
Abstract
Marine long-chain polyunsaturated fatty acids (LC n-3 PUFA) are associated with reduced risk for inflammatory diseases, such as cardiovascular diseases and rheumatoid arthritis. These fatty acids, however, are rapidly oxidized, generating highly reactive malondialdehyde (MDA), 4-hydroxy-2-hexenal (HHE) and 4-hydroxy-2-nonenal (HNE). These oxidation products may interact with DNA and proteins, thus possibly leading to impaired cell functions. Little is known about the formation of MDA, HHE and HNE in fish oil in the gastrointestinal (GI) tract. In this study, the effect of dynamic in vitro digestion of cod liver oil on the generation of MDA, HHE and HNE was evaluated using the TNO Gastro-Intestinal Model (tiny-TIM). Effects of pre-formed oxidation products, pre-emulsification of the oil, and addition of oxidants (EDTA and hemoglobin, Hb) on GI oxidation were evaluated. Formation of aldehydes occurred during GI digestion. However, only emulsified oil fortified with 11.5 μM Hb oxidized to a degree that overcame the dilution induced by gastric secretion, which caused increased aldehyde concentrations in gastric lumen up to 90 min. The maximum levels of aldehydes generated in this study were 24.5 μM MDA, 1.6 μM HHE and 0.07 μM HNE. Oils containing different amounts of pre-formed lipid oxidation products maintained the same oxidation ranking order during digestion, even though the relative changes were not directly proportional. Emulsification of the oil had an unclear effect in the gastric phase, but a pro-oxidative effect in the intestinal phase. In general, higher aldehyde levels were reached in the intestinal lumen than in the initial meal, demonstrating that GI digestion promotes oxidation. Hence, epithelial cells may be exposed to elevated amounts of reactive aldehydes for several hours after a meal containing fish oil.
1 Introduction
Marine food, such as fish and fish oil, is considered to be an important source of long chain n-3 polyunsaturated fatty acids (LC n-3 PUFA) in the human diet. LC n-3 PUFA, or omega-3, have been linked to various positive health effects in the human body. LC n-3 PUFA, e.g. in the form of eicosapentaenoic acid (EPA) and docosahexaenoic acid (DHA), are believed to reduce the risk of cardiovascular diseases (CVD),1 and have also been connected with beneficial effects on inflammatory diseases.2
However, PUFA are highly susceptible towards lipid oxidation, which degrades the oil and generates a cascade of lipid oxidation products.3 Some of the lipid oxidation products formed are highly reactive and can continue to interact with the surrounding molecules, creating protein-, and DNA-adducts, as well as cross-linkages between proteins.3 The lipid oxidation reaction is generally undesirable in the food industry, since it not only decreases the initial nutritional status of the food, e.g. by decreasing the LC n-3 PUFA content, but also gives rise to various qualitative changes, such as changes in the odor, color and texture of food.3,4 In the last 10–15 years, the potential of lipids to be oxidized also during passage through the gastrointestinal (GI) tract has been increasingly addressed,5,6 and here, the reactivity of the formed oxidation products is indeed particularly important.
A common marker molecule for the lipid oxidation reaction is malondialdehyde (MDA). MDA can be derived from a variety of molecules, but is generally connected with PUFA.7 The impact of MDA on a biological material has been widely studied,7,8 and the molecule has been shown to act in both carcinogenic and genotoxic routes.7 Other reactive lipid oxidation products that can be found in fish are 4-hydroxy-trans-2-hexenal (HHE), specifically derived from LC n-3 PUFA9,10 and 4-hydroxy-trans-2-nonenal (HNE) coming from LC n-6 PUFA.9,11 The latter could be expected to develop specifically in farmed fish fed vegetable oils since their level of LC n-6 PUFA is higher than in wild carnivorous fish.12 When adding tuna oil (10% w/w), containing ∼25% LC n-3 PUFA, to turkey meat, Tirosh et al.13 found that higher levels of MDA equivalents were detected (600 μM), compared to when refined olive oil containing 0.5% n-3 PUFA was added to the turkey meat (10% w/w; 90 μM MDA equivalents), after 180 min gastric in vitro digestion. This indicates the potency of marine oils with a high content of LC n-3 PUFA to oxidize and form MDA.
There are several factors of importance for the onset and development of the lipid oxidation reaction in the GI tract. Halliwell et al.14 stated that chemical conditions in the GI tract, such as the presence of catalyzing agents like hydroperoxides, hemoglobin (Hb) and iron ions enhance the risk of lipid oxidation.14 Kanner and Lapidot5 raised the possibility of the gastric tract to act as a hub for the lipid oxidation reaction due e.g. to the low pH and presence of oxygen that could induce lipid oxidation.5 Since then, several studies have confirmed the concerns raised, and it has recently been shown that the lipid oxidation reaction can take place in the GI tract during digestion of marine oil.15–19 Larsson et al.15 showed that lipid oxidation could occur both in the gastric and duodenal step during static in vitro digestion of bulk and emulsified cod liver oil. It was shown that pre-oxidized oil gave rise to increased formation of lipid oxidation products, and that the addition of Hb had a catalyzing effect on the lipid oxidation.15
Static in vitro digestion models are easy to use, but not fully realistic since they do not account for the addition of digestive enzymes and removal of dissolved low molecular weight digestion products and water from the system, by dialysis and filtration, over time. Dynamic models fit better to in vivo conditions and hence should give a better picture of the actual digestion mechanisms. One of the dynamic in vitro digestion systems commonly used today is the TNO Gastro-Intestinal Model (TIM), where a gradual change in pH, addition of enzymes and bile, and removal of liquid and nutrients over time are included. It has not earlier been investigated to what extent pre-formed oxidation products, emulsification and the presence of pro- and antioxidants affect oxidation of cod liver oil under dynamic in vitro digestion conditions.
In this study, cod liver oil was evaluated for its sensitivity towards GI lipid oxidation using the TIM system. The effects of pre-emulsification as well as initial oil quality were investigated on subsequent GI oxidation. The impact of including Hb, a pro-oxidant, and EDTA, a chelating agent, in the test meal was additionally evaluated. Furthermore, we wished to investigate the influence of endogenous ascorbic acid in the gastric fluid because of its bifunctional role in lipid oxidation. As oxidation responses, we determined the concentrations of reactive aldehydes (MDA, HHE and HNE), which could give an insight into the risks for potential toxicological effects from GI oxidation.
2 Materials and methods
2.1 Chemicals
Pepsin (2188 U mg−1) from porcine gastric mucosa, α-amylase (1333 U mg−1) from Bacillus sp., trypsin from bovine pancreas, Patent Blue V sodium salt, ethylenediaminetetraacetic acid (EDTA), disodium salt dihydrate, ascorbic acid, 1,1,3,3-tetraethoxypropane and 2,4-dinitrophenylhydrazine (DNPH) were purchased from Sigma-Aldrich (Stockholm, Sweden). Brij 35 was obtained from Fisher Scientific (Acros Organics, Västra Frölunda, Sweden). Lipase F-AP 15 (150 U mg−1) from Rhizopus oryzae was purchased from Amano Enzyme Inc. (Nagoya, Japan). Fresh porcine bile was obtained from TNO (Zeist, The Netherlands). Pancreatin (Pancrex-Vet, Pfizer, Karlsruhe, Germany) was purified by centrifugation (9000g, 20 min, 4 °C) before use. Wheat flour bread containing 1% vegetable oil (0.4% saturated) was bought in a local supermarket. 4-Hydroxy-2-hexenal (HHE) and 4-hydroxy-2-nonenal (HNE) were purchased from Cayman Chemicals (Michigan, USA). All other chemicals used were of analytical grade.
2.2 Cod liver oil
Refined cod liver oil without added antioxidants was supplied by LÝSI hf. (Reykjavík, Iceland). The content of total PUFA in the oil was 27.5%, the content of n-3 PUFA was 24.4% (8.2% EPA and 10.5% DHA), and the content of n-6 PUFA was 1.3%.20 In addition, it contained 48% MUFA, and 17.2% SFA.20 The fatty acid profile of the oil is reported in Jónsdóttir et al.20 The anisidine value (AV) and the peroxide value (PV) are both methods commonly used by the oil industry when assessing the quality of fish oil.21 PV detects primary oxidation products, while AV detects secondary oxidation products.22 The AV of the fresh oil was 8.5 meq kg−1 according to the manufacturer, and the PV was 1.3 mmol kg−1 oil when measured in-house. The oil was used either fresh or after storage in Erlenmeyer flasks for 6 and 9 days at room temperature to create in total three different levels of pre-formed oxidation products (Table 1). The two oxidized oils are referred to as “medium ox” (PV 12.4 mmol kg−1 oil) and “high ox” (PV 20.9 mmol kg−1 oil). All oil samples were then stored at −80 °C until use.
Table 1 Amount (mean ± SD; n = 3) of pre-formed lipid oxidation products in fresh and oxidized cod liver oil (CO). “Medium ox” oil was stored 6 days at room temperature and “highly ox” oil was stored 9 days at room temperature
|
PV (mmol kg−1 oil) |
MDA (μmol kg−1 oil) |
HHE (μmol kg−1 oil) |
HNE (μmol kg−1 oil) |
CO, cod liver oil. |
CO fresh |
1.3 ± 0.1 |
2.8 ± 0.2 |
1.9 ± 0.1 |
0.03 ± 0.01 |
CO medium ox |
12.4 ± 0.6 |
25 ± 1 |
4.1 ± 0.5 |
0.06 ± 0.01 |
CO high ox |
20.0 ± 1.8 |
47 ± 8 |
5.5 ± 1.2 |
0.08 ± 0.02 |
2.3 Test meals
Cod liver oil was thawed in ice-cold water, and for meals containing emulsified oil, an oil-in-water emulsion (20%, w/w) was freshly prepared by homogenizing (CAT X620, M. Zipperer GmbH, Germany) the oil with an emulsifier solution (17 mM Brij 35 in 10 mM phosphate buffer, pH 7.0) for 2 min at 24
000 rpm, while being kept on ice-cold water. Test meals were freshly prepared from wheat flour bread, water and 5 g cod liver oil or oil-in-water emulsion (containing 5 g cod liver oil) and mixed with 20 mL simulated saliva (containing NaCl 6.2 g L−1, KCl 2.2 g L−1, CaCl2·2H2O 0.3 g L−1, amylase 0.007 g per test meal) according to Table 2. Bread was added as a bulk food to the test meal to ensure proper gastric emptying when the oil was given. To evaluate any influence of the bread on the GI oxidation of cod liver oil during digestion, selected samples with emulsified oil were given without bread (Table 3). To simulate the gastric residues present in an empty stomach, 5 g of gastric residue (NaCl 6.1 g L−1, KCl 2.2 g L−1, CaCl2·2H2O 0.3 g L−1, 10 mM sodium acetic buffer pH 5, pepsin 264 mg L−1 and lipase 248 mg L−1) was added together with the test meal prior to the experiments. The pH of the intake was adjusted to 6.5 with 1 M NaHCO3. Blank experiments without any cod liver oil, but with or without bread, were included to determine the background level of oxidation products in the model. In test meals including EDTA (7.5 mg) or Hb (11.5 μmol L−1 emulsion), the oxidants were added just prior to feeding.
Table 2 Composition of 150 g of each test meal (referred to as intake) digested in the dynamic GI model
|
Saliva (g) |
Bread (g) |
Water (g) |
Oil/emulsion (g) |
Cod liver oil (bread) |
20 |
20 |
105 |
5 |
Cod liver oil emulsion (bread/no bread) |
20 |
20/0 |
85/105 |
25 |
Blank (bread/no bread) |
20 |
20/0 |
110/130 |
0 |
Table 3 Overview of digestion experiments with each test meal in the dynamic GI model with specification of the number of replicates with and without the addition of wheat flour bread
Test meal |
Digestion exp. |
With bread |
Without bread |
asc, ascorbic acid (85 μM in gastric secretion, 1 mL min−1). |
Blank |
3 |
2 |
1 |
Oil fresh |
2 |
2 |
|
Oil medium ox |
2 |
2 |
|
Oil medium ox + asc |
1 |
1 |
|
Oil high ox |
2 |
2 |
|
Emulsion fresh |
4 |
2 |
2 |
Emulsion fresh + Hb |
1 |
1 |
|
Emulsion high ox |
2 |
|
2 |
Emulsion high ox + EDTA |
1 |
|
1 |
2.4 Hemolysate preparation and hemoglobin quantification
Blood was obtained from freshly slaughtered farmed Atlantic cod (Gadus morhua) and purified according to Fyhn et al.23 After conversion to the carbon monoxide form, the Hb concentration was quantified against a bovine Hb standard.24 Both methods were modified as described by Richards and Hultin.25
2.5 Dynamic in vitro digestion
Computer-controlled dynamic digestions were performed in the two-compartmental digestion system called tiny-TIM (TNO, Zeist, Netherlands),26 according to the detailed digestion settings described in Larsson et al.17 Tiny-TIM simulates the gastric phase and the average digestive actions in the small intestine, including absorption. It is a slightly simplified version of the original TIM-1 system, simulating four successive compartments (stomach, duodenum, jejunum and ileum).27 Secretion of hydrochloric acid and simulated gastric juice (electrolytes, pepsin and lipase) to the stomach (1 mL min−1), as well as secretion of bicarbonate, pancreatic juice (pancreatin) and bile to the small intestine (1 mL min−1) is regulated and programmed to simulate human adult digestion of a semi-solid food. An intestinal residue containing 35 mL bile, 35 mL pancreatin and 70 mg trypsin was added prior to the start of the ingestion experiment. To evaluate the effect of endogenous concentration of ascorbic acid in gastric juice on GI oxidation, one digestion was made with 85 μM ascorbic acid in the gastric secretion. Each digestion was run for at least 180 min (prolonged digestion for 300 min). Sampling of gastric and intestinal lumen (3 mL) was done every 30 min, and sample aliquots were stored at −80 °C until analysis for lipid oxidation products.
The dilution of the test meals during dynamic digestion, due to continuous secretion of digestive compounds and removal of compounds via intestinal absorption and sampling, was verified in two experiments with the coloring agent Patent Blue V dissolved in an electrolyte. At every sampling point the change in concentration was followed by absorbance readings at 638 nm.
2.6 Analysis of MDA, HHE and HNE
The amounts of MDA, HHE and HNE were determined by LC/APCI-MS in gastric and intestinal lumen samples and in bulk oils after derivatization with DNPH according to the method described by Tullberg et al.16 Briefly, 500 μL digesta was mixed with 20 μL BHT (0.1 g mL−1 in MeOH), 40 μL EDTA (0.02 M) and 500 μL 0.25 M HCl, vortexed and incubated for 5 min. For bulk oils, 50 mg oil was vortexed with 200 μL water and the additions of EDTA, BHT and HCl were downscaled by a factor of two. Precipitated proteins were separated by centrifugation (16
000g for 2 min). Derivatization of aldehydes was performed by mixing 400 μL supernatant with 25 μL DNPH (2 mg mL−1 in MeOH) and incubated for 1 h at room temperature. Derivatives were extracted twice with 500 μL dichloromethane (16
000g for 2 min at 20 °C) and pooled extracts were evaporated under a stream of N2 gas and then dissolved in MeOH before separation and detection by LC/APCI-MS. Quantification of aldehydes was made against external standard curves of MDA, HHE and HNE treated in the same way as the samples. The limit of quantification is a signal-to-noise ratio of 10, which corresponds to 40 nM MDA, 8 nM HHE and 4 nM HNE in the external standards. All samples reported were above the limit of quantification. Since no significant differences were seen among sample types, or over time from the detection of HNE, data from this analysis is only briefly discussed in the text.
2.7 Calculations and statistics
The relative aldehyde concentration for each time point was calculated from the mean aldehyde concentration of the test meal at that point divided by the calculated remaining aldehyde concentration of the intake (see equation below). The background level provided by digested blanks was subtracted from the intestinal data, to calculate the true GI formation of oxidation products. The relative change is shown between 0–120 min in the gastric lumen. Thereafter the uncertainties of the relative values increased, due to large dilution of the intake together with the remaining small gastric digesta volume.
In general, two to four replicate digestion experiments of each test meal were made; each digestion is here referred to as “n”, i.e. n = 2–4. To screen the effect of selected oxidants, EDTA and Hb, and the effect of ascorbic acid in gastric juice, only single digestion experiments were carried out. The amount of lipid oxidation products in digests at each time point was determined by one analytical replicate (here referred to as “a”, i.e. a = 1), except for in the case of the intake, then a = 2–4. Results are shown as mean values and error bars represent standard deviation where n > 2, and (max − min)/2 where n = 2, and concentrations presented as μM of each aldehyde in the digesta. Statistical differences between two groups were determined by Student's t-test (IBM SPSS Statistics 19, IBM Corp., NY, USA). A significance level of p < 0.05 was used.
3 Results and discussion
3.1 Properties of the dynamic gastrointestinal in vitro model
Our aim in this study was to determine what levels of lipid oxidation products can be formed in the gastric and the intestinal compartments during digestion of cod liver oil using a dynamic in vitro model (tiny-TIM). Therefore, the concentration of aldehydes in the gastric and in the intestinal lumen is reported as μM in the digests over time. In order to evaluate to what extent the fish oil gets oxidized under these conditions, it is necessary to understand the dilution effect of the dynamic system. Without any formation or degradation, the initial intake concentration will change over time, according to Fig. 1. A linear decrease is observed in the gastric phase up to 150 min, and approximately 50% of the initial intake concentration remains at 90 min of digestion. At the same time, a peak of 35% is reached at 90 min after intake in the intestinal lumen.
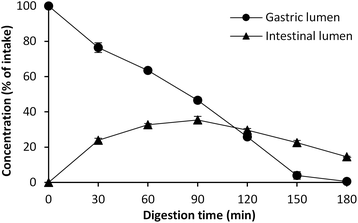 |
| Fig. 1 Concentration of Patent Blue V marker in the gastric and the intestinal compartments, expressed as percent of intake, during dynamic in vitro digestion in tiny-TIM. Values are mean ± (max − min)/2; n = 2, a = 2. | |
3.2 Effect of emulsification on MDA and HHE formation
The effect of pre-emulsification of the cod liver oil on the formation of lipid oxidation products during subsequent dynamic digestion was evaluated in both fresh and highly oxidized cod liver oil. After the emulsification treatment, the initial MDA and HHE levels of emulsion made of oxidized cod liver oil were lower compared to bulk oxidized oil (Fig. 2). In agreement with this, lower aldehyde concentrations were generated by the emulsified oxidized oil during the entire gastric digestion. However, for MDA, this difference disappeared in the intestinal phase, and in fact, significantly higher (p < 0.05) HHE levels were formed by the emulsified oxidized oil than by bulk oil at 60–180 min of digestion. Emulsification of fresh oil had no apparent impact on the absolute aldehyde values. Similar to the oxidized oil, lower concentrations of MDA were seen in the gastric digesta of emulsified fresh oil compared to bulk oil, but no difference in intestinal digesta was observed. Again, emulsified fresh oil caused elevated HHE levels (∼2-fold) compared to bulk oil during intestinal digestion. In our earlier study,17 a pro-oxidative effect of emulsification of isolated herring oil on MDA and HHE was shown in both the gastric and intestinal phase using the same emulsifier and digestion system.
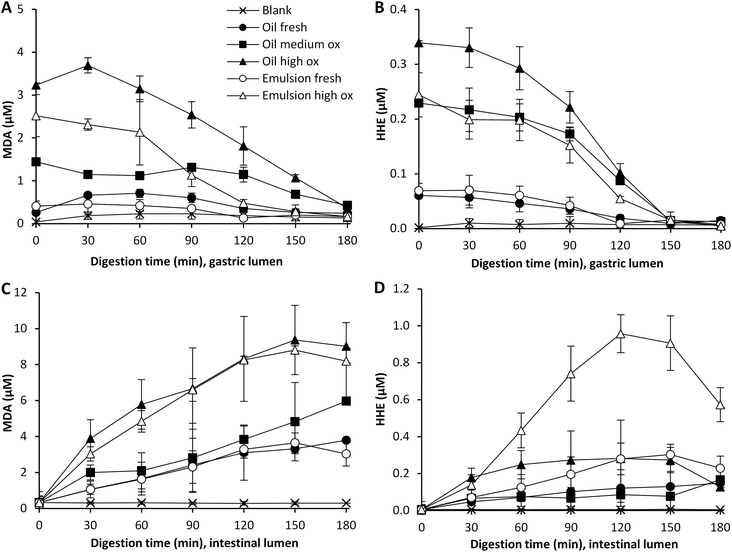 |
| Fig. 2 Formation of MDA and HHE in gastric (A and B) and intestinal lumen (C and D) during dynamic in vitro digestion of cod liver oil. Values are mean ± SD (n > 2) or (max − min)/2 (n = 2). | |
It has often been reported that a low pH increases the lipid oxidation in emulsions,28,29 however, in a study by Mei et al.30 it was shown that the susceptibility towards lipid oxidation of an emulsion stabilized by Brij 35 did not change in the pH range 3–7, when measuring TBARS and peroxide formation. The same emulsifier was used in our study, and therefore it is not surprising that the emulsions were fairly stable from an oxidative perspective, also at the low pH of the gastric phase.
Emulsions are frequently reported to be more susceptible towards lipid oxidation than their corresponding bulk oil,31,32 however, this is believed to mainly be caused by the harsh mechanical treatment during the emulsification process in the industry. The results from our study show that the emulsifier used had a somewhat protective effect in the gastric lumen. This is in line with the different initial aldehyde levels in the emulsion and the bulk oil. A slightly lower formation of MDA and HHE was observed in the emulsified oxidized oil compared to the bulk oxidized oil. Depending on several factors, such as pH, emulsifier, and droplet size distribution, an emulsion can be more or less susceptible to lipid oxidation compared to its corresponding bulk oil.31 Also the role of the concentration and structure of the emulsifier for the oxidative stability of the emulsion was pointed out by Kargar et al.,33 as well as by Nielsen et al.34 An emulsifier can act as a protective barrier, and the charge, permeability and thickness of the interface can affect the diffusion of both pro- and antioxidants over the interfacial layer of the emulsion droplet.28 It has additionally been reported that pro-oxidants can be trapped within micelles formed by emulsifier in excess,35 which could protect the oil in the emulsion droplet.
The observed effect of emulsification in the intestinal phase could be due to the change in pH and the addition of bile salts, which could lead to a shift in emulsifier distribution of the emulsion droplet surface. The unequivocal protective effect of Brij 35 in the gastric phase might also be lost in the intestinal phase due to the pH change, a shift in the composition of the aqueous phase and the competition to the droplet interface from other emulsifiers.
3.3 Effect of pre-formed oxidation products
Bulk oil with different amounts of pre-formed oxidation products developed different degrees of oxidation during digestion. Gastric digests of fresh oil showed increased MDA levels already at 30 min of digestion, and developed the highest relative increase in MDA (5-fold) at 90 min compared to digests of medium and highly oxidized oil, which generated a 2-fold and 1.7-fold increase, respectively at this point (Fig. 3A and B). The MDA levels detected were however still significantly higher (p < 0.05) in the medium and highly oxidized oil compared to the fresh oil at 60–120 min of digestion. The highly oxidized oil gave also rise to significantly higher MDA levels compared to the medium oxidized oil at the same time interval. The formation of HHE was more moderate and independent of the initial oxidation level of the oil. The relative increase was in the range of 1.3 to 1.6 at 90 min of gastric digestion. Also the emulsion made of fresh oil led to a raised relative change in MDA (1.9-fold) during the gastric phase at 90 min. MDA levels in the emulsion made of highly oxidized oil did not change. HHE increased 1.3-fold in digests of both emulsions. A drop in the relative change of HHE was seen at prolonged digestion, indicating that the dilution effect became predominant. When looking at the absolute levels of HHE detected in the gastric lumen, significantly higher levels (p < 0.05) were detected when digesting both the highly and medium oxidized oil compared to the fresh oil at 60–120 min of digestion.
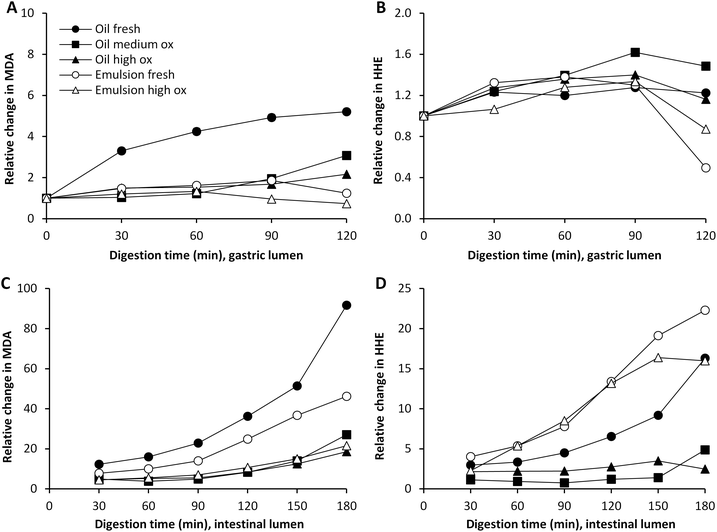 |
| Fig. 3 Relative change in concentration of MDA and HHE during gastric (A and B) and intestinal digestion (C and D) of emulsified and non-emulsified cod liver oil with different amounts of pre-formed lipid oxidation products. | |
Relative changes during the intestinal digestion related to different initial oil quality were in agreement with the pattern from the gastric digestion. Higher relative changes in MDA and HHE of fresh oil (both emulsified and non-emulsified) were seen compared to its corresponding oxidized oil (Fig. 3C and D). The results thus indicate that there is not a strict proportional formation of MDA and HHE based on the initial amount of oxidation products of the oil during dynamic in vitro digestion of cod liver oil. However, because of the relatively large differences in pre-formed oxidation products in the oils, the highest aldehyde concentrations in the gastric and intestinal lumen were in general obtained by the initially more oxidized oils (Fig. 2). In the intestinal lumen, the levels of MDA and HHE became significantly higher (p < 0.05) with the highly oxidized oil compared to digestion with the less oxidized oil, at 60–120 min of digestion. The aldehyde levels detected in the intestinal lumen with the fresh oil and the medium oxidized oil were however not significantly different any more at this time interval (MDA p = 0.55, HHE p = 0.50). In the case of low GI oxidation, as seen for HHE, the initial proportional differences will remain. Despite similar or different relative changes in aldehyde concentration, the actual concentration of reactive aldehydes to which the GI tract is exposed is crucial.
3.4 Influence of hemoglobin on gastrointestinal oxidation
Hb is one of the most pro-oxidative endogenous compounds in fish, therefore the impact of adding cod Hb to emulsified fresh cod liver oil (11.5 μmol L−1 emulsion) on GI oxidation was evaluated (Fig. 4). The addition of Hb to the emulsified oil had a strong immediate effect on the initial MDA and HHE levels in the test meal, which were about 17-fold and 3-fold higher, respectively, compared to the control without Hb. During gastric digestion in the presence of Hb, both the MDA and the HHE levels peaked at 90 min (25 μM and 0.5 μM, respectively), and the levels determined were 70- and 12-fold higher compared to fresh emulsions, and the levels of both MDA and HHE detected at 60–120 min were significantly higher (p < 0.05) compared to the control. When looking at the relative changes, emulsion with Hb gave rise to the highest levels of both MDA and HHE in the gastric tract, and HHE in the intestinal tract, compared to all test meals (Fig. 4 and 5).
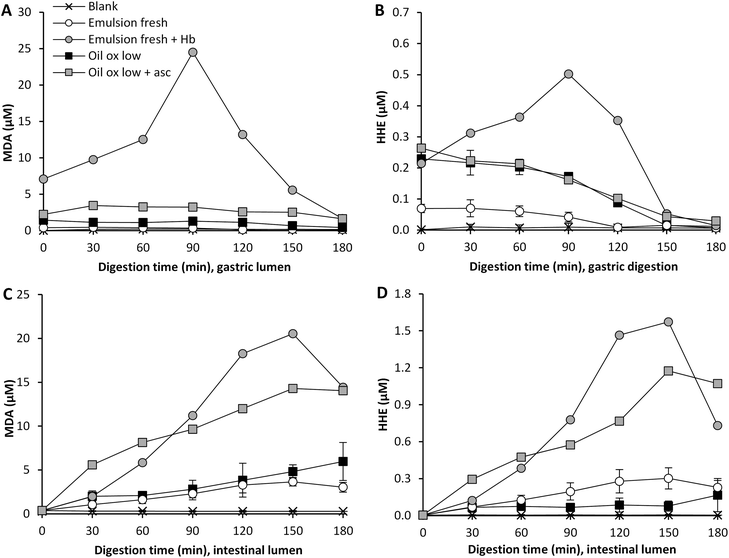 |
| Fig. 4 Effect of Hb addition to (11.5 μM) or the inclusion of 85 μM ascorbic acid in gastric secretion on the formation of MDA and HHE in gastric (A and B) and intestinal lumen (C and D) during dynamic in vitro digestion of cod liver oil. Values are mean ± SD (n > 2) or (max − min)/2 (n = 2). | |
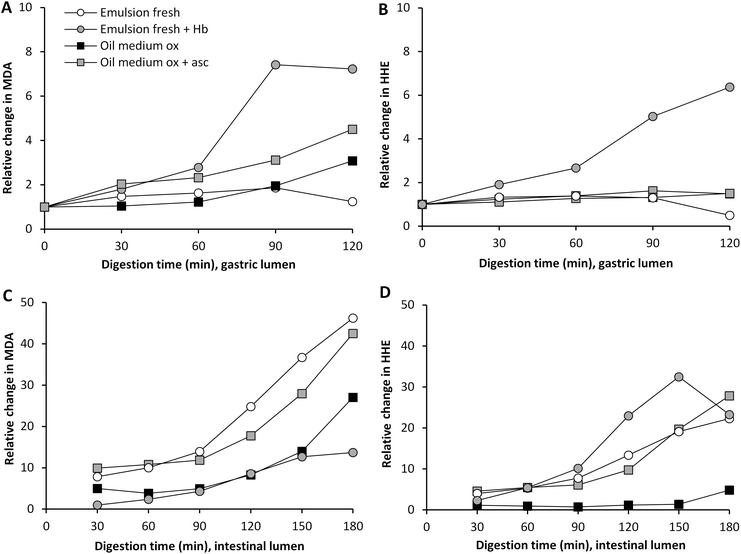 |
| Fig. 5 Relative changes in concentration of MDA and HHE during gastric (A and B) and intestinal digestion (C and D). | |
A pro-oxidative effect of 20 μM met-myoglobin was seen during in vitro digestion of emulsions made of tuna oil, soybean oil and linoleic acid.5,18 A low pH has been shown to promote lipid oxidation in fish muscle, which has been proposed to be due to the oxidation of Hb to metHb, followed by subsequent loss of the hemin group.36 A mild denaturation of the heme protein at acidic pH could also increase the exposure of the heme group, thereby promoting lipid oxidation.37 One of the suggested mechanisms for the pro-oxidative effect of heme iron is decomposition of hydroperoxides. Kumar Sau et al.38 also discussed that a change in the heme pocket could lead to binding of the iron to a distal histidine, which implies that metHb or oxyHb could be changed into low spin Fe3+ hemichromes. This transformation could lead to the generation of superoxide radicals.38 As shown in liposomes, the autooxidation of Hb generating the more pro-oxidative metHb form can moreover be stimulated by lipid hydroperoxides and aldehydes.39
3.5 Influence of ascorbic acid in simulated gastric juice
Ascorbic acid is endogenously secreted in the gastric juice, and studies on healthy human volunteers have previously reported levels of 89 and 87 μM ascorbic acid.40,41 This molecule can act both as a pro- and as an antioxidant depending on the surrounding matrix and its concentration.42 Ascorbic acid can promote lipid oxidation in the presence of trace metal ions; this is due to its reducing ability creating e.g. ferrous ions which can participate in the Fenton reaction.42 Ascorbic acid can, however, also regenerate tocopherols and act as a reactive oxygen scavenger.43 To our knowledge, it has not earlier been shown whether ascorbic acid, as a constituent in human gastric juice, would work as a pro- or an antioxidant during in vitro digestion. Ascorbic acid was added to the gastric residue prior to the experiment, which was then added to the sample at the point of intake, giving a starting concentration of 2.7 μM ascorbic acid. The gastric juice also contained 85 μM of ascorbic acid and the secretion was set at 1 mL min−1. The inclusion of ascorbic acid had a clear pro-oxidative effect on the formation of MDA in highly oxidized cod liver oil during digestion (Fig. 4). Approximately 3-fold higher relative MDA concentrations were formed in the gastric and in the intestinal compartment when ascorbic acid was present (Fig. 5), and the levels of MDA detected at 60–120 min of digestion in both the gastric and the intestinal step were significantly higher (p < 0.05) compared to the control. Ascorbic acid, however, had no effect on the gastric formation of HHE, but generated significantly higher HHE concentrations in the intestinal step at 60–120 min of digestion.
The pro-oxidative effect of ascorbic acid could be due to its relatively low concentration in combination with the presence of trace metal ions in the oil, 0.13 μM Cu and 0.7 μM Fe at intake. Decker et al.44 described that ascorbate levels found in fish muscle (60–100 μM) are effective in activating ionic iron to stimulate oxidation. Studies from beef and fish muscle have reported a shift in the activity of ascorbic acid from anti- to pro-oxidative at 2.8 mM;45,46 in purified white fish muscle with lower Fe-levels, the pro-oxidative effect was seen already to be <280 μM.45 Ascorbic acid turned from an antioxidant into a pro-oxidant at 8 mM in phosphatidylcholine liposomes fortified with 9 μmol Fe kg−1.47 Ascorbate in combination with copper or iron ions has also been shown to generate OH˙ in the gastric compartment of rats.48,49 Additionally, when ascorbic acid was included at 100 μM during static gastric in vitro digestion of a herring oil emulsion, Kristinova et al.50 saw a pro-oxidative effect.
3.6 Effect of EDTA on GI oxidation
EDTA has a metal chelating activity in oil-in-water emulsions,28 however, the effect of EDTA appears to depend on the concentration and type of oil being emulsified.51 In this study, 50 μg EDTA g−1 intake was tested together with highly oxidized oil based on the earlier findings that 10 and 50 μg EDTA g−1 fish oil enriched dressing strongly reduced lipid oxidation products during storage at room temperature.52 EDTA had a slightly decreasing effect on MDA and HHE formation in the gastric step, with a decrease of MDA (30%) and HHE (10%) formation at 90 min, compared to the control with no added EDTA (data not shown). This effect appeared to be lost in the intestinal phase, where we only saw a slight decrease in MDA (1%) and an increase in HHE (70%) formation. However, due to the low sample number with EDTA, our results can only be considered as trends.
Frankel et al.32 showed that EDTA only worked as an antioxidant in mackerel oil-in-water emulsions when added in higher concentrations than that of iron. In some food systems, the EDTA
:
iron ratio of 3.4–4.5
:
1 did not have any effect.53 In our experiments, the EDTA
:
iron ratio in the oil at the point of intake was ∼200
:
1. EDTA concentrations above 2.5 μM significantly decreased lipid oxidation in fish oil emulsions using the same emulsifier as was used here (Brij 35).54 Also during gastric digestion of herring oil, EDTA (0.7 μM) was found to reduce lipid oxidation by more than 50%.19 The EDTA concentration used in our study (0.13 mM in the test meals) should be sufficient to get an antioxidative effect of GI oxidation, however this was not the case. An explanation could be the fact that we used highly oxidized oil, containing pre-formed radicals, and thus were less dependent on the presence of iron ions for de novo radical formation.
3.7 Which concentrations of MDA, HHE and HNE are formed during digestion?
The overall highest MDA and HHE concentrations reached during dynamic digestion of cod liver oil in this study were determined in Hb enriched emulsion, 24.5 and 0.5 μM, respectively, in the gastric phase at 90 min. An almost equally high peak MDA concentration was determined in the intestinal phase at 150 min (20.5 μM). However, the HHE concentration reached was extensively higher in the intestinal phase (1.6 μM) than the gastric phase (0.5 μM). Among the oils without any added pro-oxidants, the MDA and HHE levels did generally not exceed their intake concentrations in the gastric phase. In contrast, most samples generated higher MDA and HHE concentrations in the intestinal phase with maxima at 9.4 and 1.0 μM, respectively. When digesting fresh bulk oil, the highest MDA and HHE levels reached were 3.8 μM and 0.15 μM, respectively. The relative formation of MDA and HHE was rather stable in the gastric tract (Fig. 3 and 5), the exception being fresh bulk oil, which increased already after 30 min. The high levels of MDA and HHE detected in the intestinal phase demonstrated a continued oxidation. The maximum levels reached in cod liver oil emulsion fortified by Hb were similar to the levels earlier obtained during dynamic digestion of herring mince: 27 μM MDA and 0.9 μM HHE in the gastric phase at 90 min, and 23 μM MDA and 0.5 μM HHE in the intestinal phase at 150 min.17
HNE levels, detected in the gastric and the intestinal phases did not exceed 0.07 μM in any sample, and thus were very low compared to MDA and HHE. This is in accordance with the low LC n-6 PUFA content of the cod liver oil. During gastric digestion of fresh oil, the relative increase of HNE showed a similar formation pattern to MDA, increasing 3.5 and 5-fold, respectively, at 90 min.
In static digestions of beef, 28 and 0.52 μM MDA and HNE were found at the end of the duodenal phase.55 Similar levels were found after approximate recalculations by the authors, from μmol kg−1 product to μM in the digesta, detected by Steppeler et al.56 in the duodenal phase during static in vitro digestion of salmon; 20, 0.5, 0.1 μM MDA equivalents, HHE and HNE. Further, Kenmogne-Domguia et al.18 found 240 μM MDA, 7.2 μM HHE, 1.2 μM HNE after static in vitro digestion of tuna
:
sunflower oil (50% w/w, containing 17.5% n-3 PUFA and 10% n-6 PUFA) at the end of the intestinal phase. In our own previous study57 22 μM total MDA and 0.26 μM HHE was found after static digestion of fresh cod liver oil. Since there are no available other studies reporting MDA, HHE and HNE data from dynamic in vitro digestion of marine lipids, these are the most related data we can access. As outlined above, the present study revealed that fresh cod liver oil generated 3.8 μM MDA, 0.15 μM HHE and 0.02 μM HNE after 180 min dynamic digestion. Although static models are very useful to compare e.g. different treatments, meals or oil types, it is however obvious that absolute aldehyde levels could be different from that in dynamic models due to lack of continuous secretion and product removal. This is an important feature to consider, and to establish further, if data could be used to understand potential physiological effects from aldehyde-containing digests.
4 Conclusion
The results presented in this study shows that reactive aldehydes were formed during dynamic in vitro digestion of cod liver oil, using the tiny-TIM system. The presence of cod Hb and pre-formed oxidation products largely elevated the aldehyde levels reached. Oils containing different amounts of pre-formed lipid oxidation products, maintained the same oxidation ranking order in the gastric and in the intestinal phase, even though the relative changes were not directly proportional to the initial oxidation status. This implies that it is important to prevent lipid oxidation prior to ingestion in order to minimize subsequent aldehyde formation in the GI tract. For most test meals, the highest aldehyde concentrations were formed in the intestinal phase, and the elevated levels lasted for several hours. The outcome indicates the importance of considering the whole food chain: processing, product design, storage, and meal composition to avoid post-prandial oxidative changes, and thereby maximizing the desired bioactive effects from the LC n-3 PUFA.
Conflict of interest
The authors have declared no conflict of interests.
Acknowledgements
This work was financially supported by FORMAS (The Swedish Research Council for Environment, Agricultural Sciences and Spatial Planning, Project 222-2007-1007 and Project 222-2012-1331).
Notes and references
- C. J. Lavie, R. V. Milani, M. R. Mehra and H. O. Ventura, J. Am. Coll. Cardiol., 2009, 54, 585–594 CrossRef CAS PubMed.
- P. C. Calder, Br. J. Clin. Pharmacol., 2013, 75, 645–662 CAS.
-
G. Márquez-Ruiz, F. Holgado and J. Velasco, in Food Oxidants and Antioxidants, CRC Press, 2013, pp. 79–114, DOI:10.1201/b15062-5.
- S. J. Chapple, X. Cheng and G. E. Mann, Redox Biol., 2013, 1, 319–331 CrossRef CAS PubMed.
- J. Kanner and T. Lapidot, Free Radicals Biol. Med., 2001, 31, 1388–1395 CrossRef CAS PubMed.
- S. Gorelik, T. Lapidot, I. Shaham, R. Granit, M. Ligumsky, R. Kohen and J. Kanner, J. Agric. Food Chem., 2005, 53, 3397–3402 CrossRef CAS PubMed.
- D. Del Rio, A. J. Stewart and N. Pellegrini, Nutr., Metab. Cardiovasc. Dis., 2005, 15, 316–328 CrossRef PubMed.
- H. Esterbauer, R. J. Schaur and H. Zollner, Free Radicals Biol. Med., 1991, 11, 81–128 CrossRef CAS PubMed.
- M. Guichardant, S. Bacot, P. Molière and M. Lagarde, Prostaglandins, Leukotrienes Essent. Fatty Acids, 2006, 75, 179–182 CrossRef CAS PubMed.
- F. J. G. M. Van Kuijk, L. L. Holte and E. A. Dratz, Biochim. Biophys. Acta, Lipids Lipid Metab., 1990, 1043, 116–118 CrossRef CAS.
- W. A. Pryor and N. A. Porter, Free Radicals Biol. Med., 1990, 8, 541–543 CrossRef CAS PubMed.
- C. Strobel, G. Jahreis and K. Kuhnt, Lipids Health Dis., 2012, 11, 144 CrossRef CAS PubMed.
- O. Tirosh, A. Shpaizer and J. Kanner, J. Agric. Food Chem., 2015, 63(31), 7016–7023 CrossRef CAS PubMed.
- B. Halliwell, K. Zhao and M. Whiteman, Free Radical Res., 2000, 33, 819–830 CrossRef CAS PubMed.
- K. Larsson, L. Cavonius, M. Alminger and I. Undeland, J. Agric. Food Chem., 2012, 60, 7556–7564 CrossRef CAS PubMed.
- C. Tullberg, K. Larsson, N. G. Carlsson, I. Comi, N. Scheers, G. Vegarud and I. Undeland, Food Funct., 2016, 7, 1401–1412 CAS.
- K. Larsson, H. Harrysson, R. Havenaar, M. Alminger and I. Undeland, Food Funct., 2016, 7, 1176–1187 CAS.
- H. B. Kenmogne-Domguia, S. Moisan, M. Viau, C. Genot and A. Meynier, Food Chem., 2014, 152, 146–154 CrossRef CAS PubMed.
- V. Kristinova, I. Storrø and T. Rustad, Food Chem., 2013, 141, 3859–3871 CrossRef CAS PubMed.
- R. Jónsdóttir, M. Geirsdottir, P. Y. Hamaguchi, P. Jamnik, H. G. Kristinsson and I. Undeland, J. Sci. Food Agric., 2016, 96, 2125–2135 CrossRef PubMed.
- GOED, GOED Voluntary Monograph Version 5: Global Organisation for EPA and DHA Omega-3, http://goedomega3.com/index.php/files/download/350 (accessed 06-14, 2016).
-
M. Hu, in Oxidative Stability and Shelf Life of Foods Containing Oils and Fats, AOCS Press, 2016, pp. xvii–xxxviii, DOI:10.1016/B978-1-63067-056-6.02001-2.
- U. E. H. Fyhn, H. J. Fyhn and B. J. Davis, Comp. Biochem. Physiol., Part A: Mol. Integr. Physiol., 1979, 62, 39–66 CrossRef.
- W. D. Brown, J. Biol. Chem., 1961, 236, 2238–2240 CAS.
- M. P. Richards and H. O. Hultin, J. Agric. Food
Chem., 2002, 50, 555–564 CrossRef CAS PubMed.
- R. Havenaar, A. De Jong, M. E. Koenen, J. Van Bilsen, A. M. Janssen, E. Labij and H. J. M. Westerbeek, J. Agric. Food Chem., 2013, 61, 7636–7644 CrossRef CAS PubMed.
- M. Minekus, P. Marteau and R. Havenaar, Altern. Lab. Anim., 1995, 23, 197–209 Search PubMed.
- T. Waraho, D. J. McClements and E. A. Decker, Trends Food Sci. Technol., 2011, 22, 3–13 CrossRef CAS.
- C. Jacobsen, M. Timm and A. S. Meyer, J. Agric. Food Chem., 2001, 49, 3947–3956 CrossRef CAS PubMed.
- L. Mei, D. J. McClements, J. Wu and E. A. Decker, Food Chem., 1998, 61, 307–312 CrossRef CAS.
-
C. Jacobsen, in Oxidative Stability and Shelf Life of Foods Containing Oils and Fats, ed. H. a. Jacobsen, AOCS Press, 2016, ch. 8, pp. 287–312, DOI:10.1016/B978-1-63067-056-6.00008-2.
- E. N. Frankel, T. Satué-Gracia, A. S. Meyer and J. B. German, J. Agric. Food Chem., 2002, 50, 2094–2099 CrossRef CAS PubMed.
- M. Kargar, F. Spyropoulos and I. T. Norton, J. Colloid Interface Sci., 2011, 357, 527–533 CrossRef CAS PubMed.
- N. S. Nielsen, A. F. Horn and C. Jacobsen, Eur. J. Lipid Sci. Technol., 2013, 115, 874–889 CrossRef CAS.
- C. C. Berton-Carabin, M.-H. Ropers and C. Genot, Compr. Rev. Food Sci. Food Saf., 2014, 13, 945–977 CrossRef CAS.
- I. Undeland, H. G. Kristinsson and H. O. Hultin, J. Agric. Food Chem., 2004, 52, 4444–4451 CrossRef CAS PubMed.
- C. P. Baron, L. H. Skibsted and H. J. Andersen, J. Agric. Food Chem., 2002, 50, 883–888 CrossRef CAS PubMed.
- A. Kumar Sau, D. Currell, S. Mazumdar and S. Mitra, Biophys. Chem., 2002, 98, 267–273 CrossRef CAS PubMed.
- R. Maestre, M. Pazos and I. Medina, J. Agric. Food Chem., 2009, 57, 7013–7021 CrossRef CAS PubMed.
- G. M. Sobala, C. J. Schorah, M. Sanderson, M. F. Dixon, D. S. Tompkins, P. Godwin and A. T. R. Axon, Gastroenterology, 1989, 97, 357–363 CrossRef CAS.
- A. J. Waring, I. M. Drake, C. J. Schorah, K. L. M. White, D. A. F. Lynch, A. T. R. Axon and M. F. Dixon, Gut, 1996, 38, 171–176 CrossRef CAS PubMed.
- S. Kojo, Curr. Med. Chem., 2004, 11, 1041–1064 CrossRef CAS PubMed.
- M. Laguerre, J. Lecomte and P. Villeneuve, Prog. Lipid Res., 2007, 46, 244–282 CrossRef CAS PubMed.
- E. A. Decker, C.-H. Huang, J. E. Osinchak and H. O. Hultin, J. Food Biochem., 1989, 13, 179–186 CrossRef CAS.
- J. C. Deng, R. F. Matthews and C. M. Watson, J. Food Sci., 1977, 42, 344–347 CrossRef CAS.
- K. Sato and G. R. Hegarty, J. Food Sci., 1971, 36, 1098–1102 CrossRef CAS.
- E. A. Decker and H. O. Hultin, J. Food Sci., 1990, 55, 947–950 CrossRef CAS.
- M. B. Kadiiska, P. M. Hanna, L. Hernandez and R. P. Mason, Mol. Pharmacol., 1992, 42, 723–729 CAS.
- A. Slivka, J. Kang and G. Cohen, Biochem. Pharmacol., 1986, 35, 553–556 CrossRef CAS PubMed.
- V. Kristinova, I. Storrø and T. Rustad, Food Chem., 2013, 141, 3859–3871 CrossRef CAS PubMed.
- C. Jacobsen, M. B. Let, N. S. Nielsen and A. S. Meyer, Trends Food Sci. Technol., 2008, 19, 76–93 CrossRef CAS.
- M. B. Let, C. Jacobsen and A. S. Meyer, J. Agric. Food Chem., 2007, 55, 2369–2375 CrossRef CAS PubMed.
- M. B. Let, C. Jacobsen, K. A. Pham and A. S. Meyer, J. Agric. Food Chem., 2005, 53, 5429–5437 CrossRef CAS PubMed.
- J. Alamed, D. J. McClements and E. A. Decker, Food Chem., 2006, 95, 585–590 CrossRef CAS.
- T. Van Hecke, J. Vanden Bussche, L. Vanhaecke, E. Vossen, J. Van Camp and S. De Smet, J. Agric. Food Chem., 2014, 62, 1980–1988 CrossRef CAS PubMed.
- C. Steppeler, J.-E. Haugen, R. Rødbotten and B. Kirkhus, J. Agric. Food Chem., 2016, 64, 487–496 CrossRef CAS PubMed.
- K. Larsson, K. Istenič, T. Wulff, R. Jónsdóttir, H. Kristinsson, J. Freysdottir, I. Undeland and P. Jamnik, J. Sci. Food Agric., 2015, 95(15), 3096–3106 CrossRef CAS PubMed.
|
This journal is © The Royal Society of Chemistry 2016 |
Click here to see how this site uses Cookies. View our privacy policy here.