DOI:
10.1039/C6FO00073H
(Paper)
Food Funct., 2016,
7, 1655-1663
Grape skin phenolics as inhibitors of mammalian α-glucosidase and α-amylase – effect of food matrix and processing on efficacy
Received
18th January 2016
, Accepted 21st February 2016
First published on 23rd February 2016
Abstract
Type-2 diabetes is continuously increasing worldwide. Hence, there is a need to develop functional foods that efficiently alleviate damage due to hyperglycaemia complications while meeting the criteria for a sustainable food processing technology. Inhibition of mammalian α-amylase and α-glucosidase was studied for white grape skin samples recovered from wineries and found to be higher than that of the drug acarbose. In white grape skins, quercetin and kaempferol derivatives, analysed by UPLC-DAD-MS, and the oligomeric series of catechin/epicatechin units and their gallic acid ester derivatives up to nonamers, analysed by MALDI-TOF-MS were identified. White grape skin was then used for enrichment of a tomato puree (3%) and a flat bread (10%). White grape skin phenolics were found in the extract obtained from the enriched foods, except for the higher mass proanthocyanidin oligomers, mainly due to their binding to the matrix and to a lesser extent to heat degradation. Proanthocyanidin solubility was lower in bread, most probably due to formation of binary proanthocyanin/protein complexes, than in tomato puree where possible formation of ternary proanthocyanidin/protein/pectin complexes can enhance solubility. Enzyme inhibition by the enriched foods was significantly higher than for unfortified foods. Hence, this in vitro approach provided a platform to study potential dietary agents to alleviate hyperglycaemia damage and suggested that grape skin phenolics could be effective even if the higher mass proanthocyanidins are bound to the food matrix.
Introduction
The World Health Organization (WHO) estimated that there are 346 million people worldwide suffering from diabetes and this figure will double by the year 2030. Hence, there is a need to develop new and sustainable dietary strategies that efficiently alleviate damage due to hyperglycaemia complications.
The first step to limit excessive postprandial glucose excursion is to inhibit the activity of starch digestion enzymes, i.e., α-glucosidase and α-amylase. Many studies have shown that phenolic phytochemicals can act as enzyme inhibitors towards these enzymes, along with various enzymes involved in inflammation.1–5 To make healthier foods while meeting the criteria for sustainable production, byproducts of plant food processes could be recovered and used as phenolic-rich food ingredients.6–9 Furthermore, an additional recommendation to avoid inefficiencies is to use less refined ingredients, aimed at ingredient functionality rather than purity.10 Grape skin, the main byproduct of winemaking, is a rich source of phenolics and it is available in large amounts at a low price. Grape skin phenolics comprise various compounds including monomeric flavonoids and especially proanthocyanidins.11–13 Studies carried out on animal models have opened a promising strategy to specifically prevent postprandial hyperglycaemia by using grape skin phenolics. Hogan and coworkers14 reported that phenolic-rich red and white grape pomace (skins and seeds) extract suppresses postprandial hyperglycaemia in diabetic mice following a potato starch challenge, most likely due to α-glucosidase activity inhibition. da Silva and coworkers15 found that extracts rich in procyanidins, the main components among grape skin phenolics, inhibit α-amylase activity in rats following a corn starch challenge, thus leading to the prevention of a postprandial increase in blood glucose level.
It is worth noting that white grape skin has been proposed for use as a food ingredient for common foods, such as bread and tomato puree.16,17 A liking test with consumers was performed to evaluate the impact of grape skin addition on the overall liking of the fortified foods and establish the threshold level at which it can be incorporated. Based on sensory assessment, white grape skin was added up to 10% in bread16 and up to 3% in tomato puree.17 Hence, grape skin could be incorporated into foods and provide a dietary means to alleviate hyperglycaemia and diabetes’ complications in a sustainable perspective. On the other hand, effectiveness of the unfractionated phenolic pool of grape skin as α-glucosidase and α-amylase inhibitors with respect to a standard, such as the conventional drug acarbose, has not been evaluated. Additionally, it is important to test the effectiveness of grape skin phenolics when they are incorporated in a complex food system, where these compounds could bind to the food matrix thus decreasing or losing their efficacy. Hence, the aims of the current study were: (1) to evaluate inhibitory activity of various white grape skin batches towards mammalian starch digestion enzymes; (2) to study the effect of the food matrix on phenolic solubility and inhibition of mammalian starch digestion enzymes in a tomato puree and unleavened bread enriched with grape skin.
Materials and methods
Chemicals
Standard phenolics, namely, catechin, epicatechin, quercetin 3-O-galactoside, quercetin 3-O-glucuronide, quercetin 3-O-glucoside, quercetin 3-O-rhamnoside, kaempferol 3-O-galactoside, kaempferol 3-O-glucuronide, kaempferol 3-O-glucoside, isorhamnetin 3-O-glucoside, quercetin and kaempferol, trans-resveratrol, rat intestinal acetone powder (N1377-5G), p-nitrophenyl α-D-glucoside (p-NPG), acarbose, porcine pancreatic α-amylase, type VI-B (A3176), p-nitrophenyl α-D-maltopentaoside (p-NPGP), low mass standard peptides for MALDI-TOF MS analysis and all other chemicals were from Sigma Aldrich (Milan, Italy).
Grape skin collection and processing
Unfermented white grape pomace samples were collected from various wineries and sieved (with a 5 mm sieve) to separate the skins from the seeds, which were dispatched for oil extraction. The skins are a vegetable material rich in sugars that rapidly undergo spontaneous fermentation,18 hence they were frozen, transferred to the lab and dried at 55 °C for 48 h to reach a water activity level below 0.3 to prevent microbial growth and to stabilize flavonols19 and flavanols.20 Dried samples were milled by a grinder (Sunbeam Osterizer blender, Boca Raton, FL, USA) and sieved by using an Octagon Digital sieve shaker (Endecotts Ltd, London, United Kingdom), with three certified sieves (openings: 125, 250 and 500 μm), by continuous sieving for 10 min at amplitude 8. The medium fraction (particle sizes ≥125 μm ≤ 250 μm) was used. All the samples were collected and stored under vacuum in the dark at 4 °C.
Moisture, dietary fibre, protein, carbohydrates and fat contents
Moisture content was determined by drying in a vacuum oven at 70 °C and 50 Torr for 18 h. Protein, fat and carbohydrate contents were measured according to AOAC21 official methods of analysis. Glucose and fructose were determined as described previously.22 Dietary fibre content was determined by the Megazyme total dietary fibre assay procedure based on an AOAC method.21
Preparation of the fortified model foods
For preparation of the first model food, a commercial tomato puree was used. In the fortified tomato purees, a white grape skin ingredient (milled fraction with particle sizes in the range: ≥125 μm ≤ 250 μm) was added at a ratio of 3.0 g in 100 g on fresh weight basis (i.e. 36.8% on dry weight basis). The puree was filled into different glass bottles (250 mL capacity) and subjected to microwave heating (8 min at 900 Watt). During heating, the temperature of the tomato puree was monitored by using a thermocouple set in the geometric centre of one of the bottles (the slowest heating point) to attain the heating curve and calculate the pasteurisation effect. Six decimal reductions (6D) of the target microorganism Alicyclobacillus acidoterrestris was achieved (Dref = 1.5 min, Tref = 95 °C and z-value = 7 °C), which can be considered effective for acidic foods.23 For preparation of the second model food, a commercial wheat flour was used. The same white grape skin ingredient used for fortified tomato puree preparation was added to wheat flour at a ratio of 10 g in 100 g of bread on fresh weight basis (i.e. 11.6% on dry weight basis) and then water was added. The resulting dough was baked at 200 °C for 30 min. Control tomato puree and unleavened flat bread with no addition of the grape skin ingredient were considered in parallel.
Sample extraction
To perform a screening study, phenolics were extracted from white grape skin as described previously.24 For phenolic extraction from the fortified tomato puree and control tomato puree, 3.75 g was weighed in triplicate and added to 1.9 mL of water, 7 mL of methanol and 0.3 mL of formic acid and extracted for 2 h at 60 °C with continuous stirring. The mixture was centrifuged at 10
000g for 10 min, the supernatant recovered and the solid residue was re-extracted using 10 mL of a methanol/water/formic acid (70
:
29.9
:
0.1, v/v/v) extractant. The extracts were then pooled. For phenolic extraction from the fortified bread and control bread, 2 g was weighed in triplicate and extracted with 20 mL of the methanol/water/formic acid (70
:
29.9
:
0.1, v/v/v) extractant in two steps as described for tomato puree. For phenolic extraction from the grape skins used for the preparation of the model systems, an aliquot of 1 g was weighed in duplicate, added with 20 mL methanol/water/formic acid (70
:
29.9
:
0.1, v/v/v) and extracted in two steps as described for the model systems. Extracts were stored at −20 °C until use (within 1 month).
Oligomeric proanthocyanidins
Oligomeric proanthocyanidin content was analysed in grape skins as described previously.24 Briefly, 1 mL of the extract was added to 6 mL of n-butanol/HCl (95
:
5, v/v) and 0.2 mL of 2% NH4Fe(SO4)2. 12 H2O in 2 M HCl. Hydrolysis was carried out at 95 °C for 40 min. The reaction mixtures were cooled and the absorbance was measured at 550 nm against a blank made as for the sample but incubated at room temperature. For each extract, 2–4 dilutions in methanol/water/formic acid (70
:
29.9
:
0.1, v/v/v) were assessed in duplicate. Oligomeric proanthocyanidin amount was expressed as grams per kilogram of dry product, using 1.79 as a conversion factor.
Oligomeric proanthocyanidin analysis by matrix-assisted laser desorption ionization time-of-flight mass spectroscopy (MALDI-TOF MS)
For MALDI-TOF MS analysis, sample extracts containing oligomeric proanthocyanidins were purified on SPE C18 EC cartridges (Isolute from Step-Bio, Bologna, Italy), rinsed with 5 mL methanol and 5 mL Milli-Q water; 2 mL of the sample was loaded by gravity, the cartridge was washed with 5 mL of Milli-Q water and dried under vacuum for 30 min. The SPE column was eluted with 5 mL of trifluoroacetic acid (TFA) (1% in methanol v/v); the sample was dried under a nitrogen stream at 40 °C, then redissolved in 1 mL methanol and analysed by MALDI-TOF-MS. MALDI-TOF MS experiments were carried out using a PerSeptive BioSystems (Framingham, MA, USA) Voyager DE-Pro instrument, equipped with an N2 laser (337 nm, 3 ns pulse width, 20 Hz repetition rate). Mass spectrum acquisition was performed in both positive linear and reflectron mode. The instrument operated with an accelerating voltage of 20 kV. Even if several matrices have been tested (including α-cyano-4-hydroxycinnamic acid, CHCA), in agreement with most of the literature, 2,5-dihydroxybenzoic acid (DHB) provided clearly superior performances. The matrix solution was prepared by dissolving 10 mg of crystalline DHB in 1 mL methanol containing 0.1% TFA. Typically, 250 laser pulses or more were acquired for each mass spectrum. External mass calibration was performed with a separate acquisition using a mixture of low mass standard peptides provided by the instrument producer. To check for repeatability, samples were run at least in triplicate. Mass spectra were analysed using Data Explorer 4.0 software (PerSeptive BioSystems).
Polyphenol analysis by high performance liquid chromatography with diode-array detector-mass spectrometry (UPLC-DAD-MS)
Polyphenol analysis was performed as described previously.25 The chromatographic system consisted of an UPLC mod. Acquity (Waters, Milford, MA, USA) equipped with a mod. E-lambda photodiode array detector (Waters) and a triple quadrupole mass spectrometer mod. Quattro micro (Waters), operated by Masslynx 4.0 software (Micromass) with Quan-Optimize option for a fragmentation study. A 1.7 lm BEH C18 column (150 × 2.1 mm; Waters) was used for separation at a flow-rate of 0.55 mL min−1. The column was maintained at 55 °C and the separation was performed by means of a linear gradient elution. Eluents were: (A) 0.1% formic acid and (B) 0.1% formic acid in acetonitrile. The gradient was as follows: 5–20% B in 9 min, 20–35% B in 3 min, and then 80% B for 3 min. DAD analysis was carried out in the range of 200–450 nm (integration 280 and 354 nm). The mass spectrometer was operated in negative full-scan mode in the range of 100–1000 m/z. The capillary voltage was set to 3 kV, the cone voltage was specific for each compound, the source temperature was 130 °C, and the desolvating temperature was 300 °C. Calibration curves were built with catechin (280 nm), epicatechin (280 nm) and quercetin 3-O-glucoside (reference compound for all flavonols, at 353 nm). Concentrations of phenolic compounds were expressed as milligrams per kilogram of dry product.
In vitro α-glucosidase and α-amylase inhibition assay
A crude α-glucosidase solution was prepared from rat intestinal acetone powder. Briefly, 200 mg of rat intestinal acetone powder was dissolved in 4 mL of 50 mM ice cold phosphate buffer (pH 6.8) and sonicated for 15 min at 4 °C. The suspension was vortexed for 20 min and then centrifuged at 10
000 g at 4 °C for 30 min. The resulting supernatant was used for the assay. For the α-glucosidase activity assay, 650 μL of 50 mM phosphate buffer, pH 6.8; 100 μL of the enzyme solution and 50 μL of grape skin extract were added in Eppendorf tubes and pre-incubated for 5 min at 37 °C. Then, 200 μL of 1 mM pNPG was added as substrate and the mixture was further incubated at 37 °C for 25 min.2
For the pancreatic α-amylase assay, 550 μL of 50 mM phosphate buffer, pH 6.8, 200 μL of the enzyme solution (10 μM in the same buffer) and 50 μL of grape skin extract were added in Eppendorf tubes, pre-incubated for 5 min at 37 °C. Then, 200 μL of 1 mM pNPGP was added to the tubes as the substrate and the mixture was further incubated at 37 °C for 55 min.5
For both enzymatic reactions, the assay mixture was centrifuged at 10
000 g for 3 min and the absorbance of the clear supernatant was recorded at 405 nm. The control was run by addition of the extraction solvent replacing the sample. A sample blank and a control blank were run without addition of substrate and without addition of both substrate and sample, respectively.
Acarbose was used as a reference inhibitor for both enzymatic reactions. Dose–response curves were made for samples and acarbose. For acarbose results are reported as I50 (μg mL−1), i.e. concentration that inhibited the reaction by 50%; for grape skin samples, results are reported as I50 GAE, i.e. concentration of phenolics (μg mL−1) that inhibited the reaction by 50%.
Statistical analysis of data
Experimental data were analysed by one-way ANOVA, using the least significant difference (LSD, p < 0.05) as a multiple range test by Statgraphics 5.1 (STCC Inc.; Rockville, MD, USA). Results are reported as the average ± SD.
Results and discussion
Inhibition of α-glucosidase by grape skins
Rat intestine is used as a source for mammalian α-glucosidase activity, i.e. exohydrolysis of the 1-4-α-glucosidic linkage, due to the presence of two intestinal brush border membrane-bound glycohydrolases: maltase–glucoamylase and sucrase–isomaltase. Given that the genes for these glycohydrolases arose from duplication and divergence of an ancestral gene, these enzymes are similar in sequence and both of them are able to hydrolyze pNPG.26 Unrefined phenolic fractions of various white grape skin samples recovered from wineries were studied as potential inhibitors of this enzyme, in comparison with the drug acarbose. Soluble white grape skin antioxidants are mainly comprised of oligomeric proanthocyanidins, monomeric flavanols and flavonols, with a variety-dependent concentration of total phenolics, in the range from 4.6–14.5 g per kg d.w.24 The inhibition efficacy was expressed on a phenolic content basis (I50 GAE values), as in previous screening studies of natural phenolic sources.27 As shown in Table 1, inhibitory activity of various grape skins samples was in the range 30.9 (MO)–93.1 (MT) μg GAE mL−1. These values are relatively good in comparison with values found for other berries under similar assay conditions, which were in the range 20–200 μg GAE mL−1.27 The I50 for the therapeutically used inhibitor, i.e., acarbose was 100 μg mL−1. Hence, the efficacy ranking was generally higher for the white grape skins than for acarbose as previously observed for black currant and rowanberry, but not for raspeberry and cloudberry.27 Crude extracts of 24 plants, not including grape skins, have been previously analyzed for α-glucosidase inhibitory activity and 11 out of 24 extracts were found to be more efficient than acarbose. Phenolic characterization was provided only for the most efficient extract and quercetin and its derivatives were identified as main α-glucosidase inhibitors.3 Correspondingly, studies on purified phenolic compounds have revealed flavonol compounds, such as quercetin and its derivatives28 have a particularly high affinity towards the α-glucosidase enzyme in vitro. Quercetin derivatives are also present in white grape skins.12,24 In addition, proanthocyanidins, the main class among grape skin phenolics, have been demonstrated to inhibit this enzyme.29 It is worth noting that, grape skin has an additional advantage with respect to the sources considered previously, since it is available in large amount as a low-cost byproduct of winemaking. Taking into account these observations, grape skins could provide sustainable inhibitors of α-glucosidase.
Table 1 α-Glucosidase and α-amylase inhibition (I50 GAE, μg GAE mL−1) by phenolic extracts of white grape skins recovered from winemaking byproducts
Grape skins |
α-Glucosidase inhibition |
α-Amylase inhibition |
Values are the mean ± SD. Different apices in the same column indicate significant differences (LSD, p < 0.05). |
NA |
45.2b ± 2.1 |
17.1c ± 2.1 |
MO |
30.9a ± 1.5 |
12.5a ± 0.8 |
MT |
93.1d ± 3.2 |
26.3d ± 0.2 |
CH |
39.4b ± 2.1 |
15.1b ± 1.1 |
ER |
45.0b ± 3.2 |
15.6b ± 0.8 |
AR |
41.6b ± 0.8 |
16.0c ± 0.2 |
RI |
64.8c ± 2.3 |
27.4d ± 1.3 |
Inhibition of α-amylase by winemaking byproducts
Alpha-amylase is an endo-acting enzyme, which hydrolyses the 1-4-α-glucosidic linkage but cannot hydrolyze the 1-6-α-glucosidic linkage.26Table 1 shows the inhibitory activity of phenolic extracts obtained from various white grape skin varieties towards α-amylase. The I50 GAE values varied from 12.5 (MO) to 27.4 (RI) μg GAE mL−1. The I50 of acarbose was 30 μg mL−1. Hence, the efficacy ranking was generally higher for white grape skins than for acarbose. Accordingly, proanthocyanidin-rich extracts of fruit peel were found to inhibit α-amylase, with equal or higher efficacy than acarbose: the inhibitory potential increased with increasing mean degree of polymerization of proanthocyanidin.30 Proanthocyanidins specifically interact with α-amylase in vitro, by forming aggregates that hinder enzymatic activity.30,31 In addition, proanthocyanidin-rich extracts have been demonstrated to diminish the postprandial glycemic level in rats after starch administration.15
Inhibition of α-glucosidase and α-amylase activities by foods enriched with grape skins
Molecular interactions occur between polyphenols and other components present in the food matrix. Binding of polyphenols with protein molecules is driven by hydrogen bonding or hydrophobic interaction leading to the formation of soluble or insoluble polyphenol–protein aggregates.32–34 The main features of the polyphenol structure that influence their interactions with protein have been reported to be the number of galloyl ester groups and the degree of polymerization.35 In fact, heat changes associated with the addition of a model proline-rich peptide to non-galloylated monomers (catechin and epicatechin) were negligible compared to the addition of galloylated monomers (epicatechin gallate, with binding constant of 8.1 × 104 M−1) and especially to flavanol oligomers (mean degree of polymerization 4, with binding constant of 34.3 × 104 M−1).35 Proanthocyanidins have more potential interaction sites than monomeric phenolic compounds and therefore may bind to several protein molecules, causing protein crosslinking.30 Hence, proanthocyanidins, which are the main phenolic class in grape skins, can potentially interact with the food matrix.
The presence of polysaccharides like pectin is another important factor that affects the interaction between proanthocyanidin and protein.36 Pectins were demonstrated to form ternary complexes among protein/polyphenol/polysaccharide with enhanced solubility in aqueous medium.36 Hence, it may be hypothesized that the solubility of grape skin phenolics incorporated in a complex food mixture and their efficacy as α-glucosidase and α-amylase inhibitors could be lower than that resulting from grape skin alone.
To investigate the “matrix effect”, both tomato puree, which is frequently consumed with starch containing foods and flat wheat bread, which is rich in starch, were fortified with a grape skin ingredient and studied. The white grape variety Chardonnay was used for fortification since it is one of the oldest and most widely distributed wine grape cultivars and it is of commercial importance for the world's wine-producing nations.37 Both the amount of white grape skin added to these matrices and the processing steps have been optimized in a pilot-plan scale to get good consumer acceptance and nutritional benefits.16,17 Hence, these fortified foods can be considered as “real food matrices”. Major components in tomato puree were: carbohydrate: 3.6 ± 0.1 g per 100 g f.w., protein: 0.80 ± 0.1 g per 100 g f.w., dietary fibre: 0.6 ± 0.1 g per 100 g f.w., and fat: 0.05 ± 0.01 g per 100 g f.w. Major components in wheat flour were: carbohydrate: 73.0 ± 0.1 g per 100 g f.w., protein: 9.0 ± 0.1 g per 100 g f.w., dietary fibre: 2.0 ± 0.1 g per 100 g f.w., and fat: 0.10 ± 0.01 g per 100 g f.w. The amount of grape skin ingredient added in the model foods was different. In fact in a semiliquid food such as a puree, 3% addition of the grape skin ingredient results in acceptable liking from consumers.17 In a baked product, the addition rate can be increased to 10%.16
The qualitative profile of monomeric flavonoids was detected in grape skin extract by UPLC-DAD-MS (Fig. 1). The content of major phenolics namely, catechin, epicatechin, quercetin and kaempferol derivatives is reported in Table 2. Structural data about oligomeric proanthocyanidins were obtained by MALDI-TOF MS analysis (Fig. 2). MALDI-TOF allowed for measurement of masses in complex mixtures of low and high molecular weight compounds. In the grape skin sample, an oligomeric series of catechin/epicatechin units and their gallic acid ester derivatives (sodium adduct ions, MNa+), up to the nonamers (Table 3) was detected (Fig. 2a). Additionally, masses corresponding to a series of polygalloyl polyflavans were also detected. The oligomeric proanthocyanidin content of grape skins was evaluated upon hydrolysis with n-butanol/HCl and found to be much higher than that of monomeric flavonoids (Table 2).
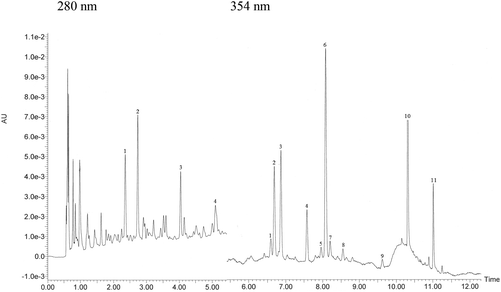 |
| Fig. 1 UPLC-DAD profile of monomeric flavonoids of white grape skin. Identified compounds at 280 nm: 1 catechin–catechin dimer, 2 catechin, 3 epicatechin, 4 epicatechin–epicatechin dimer, and at 354: 1 quercetin 3-O-galactoside, 2 quercetin 3-O-glucuronide, 3 quercetin 3-O-glucoside, 4 kaempferol 3-O-galactoside, 5 kaempferol 3-O-glucuronide, 6 kaempferol 3-O-glucoside, 7 quercetin 3-O-rhamnoside, 8 isorhamnetin 3-O-glucoside, 9 trans-resveratrol, 10 quercetin and 11 kaempferol. | |
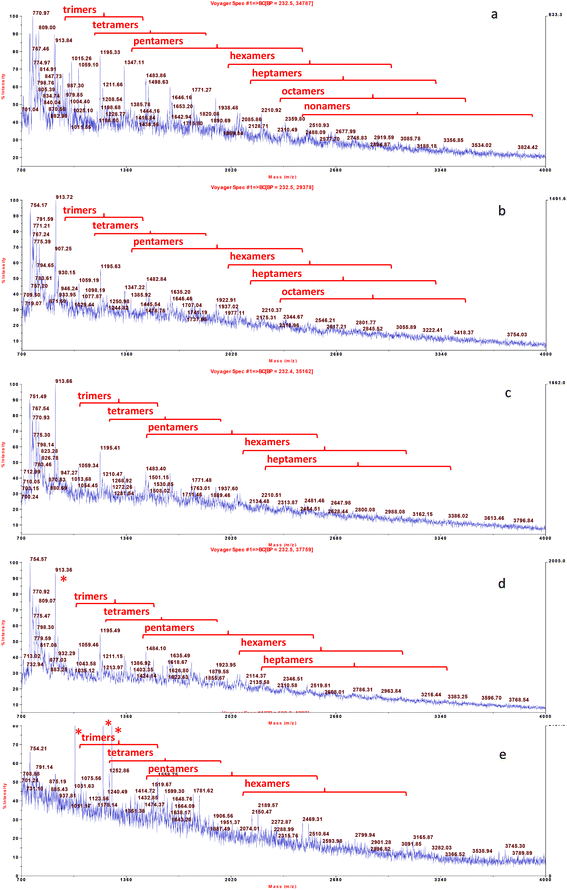 |
| Fig. 2 MALDI-TOF-MS analysis of proanthocyanidin oligomers extracted from white grape skin and its formulations with food matrices. From the top: grape skin extract (a), fortified dough (b), fortified bread (c), fortified tomato puree after mixing (d), and fortified tomato puree after heat treatment (e). Oligomeric series of catechin/epicatechin units and their gallic acid ester derivatives (sodium adduct ions, MNa+), were detected up to nonamers (Table 3). * Compounds from tomato. | |
Table 2 Monomeric flavanol (g per kg d.w.), flavonol (g per kg d.w.) and oligomeric proanthocyanidins (g per kg d.w.) contents of the white grape skin ingredient used for model food preparation
Phenolic content |
Data are the mean values ± SD (n = 3). |
Catechin |
0.25 ± 0.02 |
Epicatechin |
0.53 ± 0.04 |
Quercetin 3-O-glucuronide |
0.11 ± 0.02 |
Quercetin 3-O-glucoside |
0.10 ± 0.01 |
Quercetin 3-O-rhamnoside |
0.025 ± 0.005 |
Kaempferol 3-O-galactoside |
0.077 ± 0.005 |
Kaempferol 3-O-glucuronide |
0.041 ± 0.008 |
Kaempferol 3-O-glucoside |
0.27 ± 0.04 |
Quercetin |
0.14 ± 0.06 |
Kaempferol |
0.10 ± 0.02 |
Oligomeric proanthocyanidins |
18.5 ± 0.2 |
Table 3 Oligomeric proanthocyanidin detected by MALDI-TOF MS in grape skin (GS) extract and in the extracts obtained from the food matrices added with white grape skins (GS)
|
Molecular mass, Da (Na + ions) |
Theor. |
Measured (samples) |
|
Wheat flat bread + GS |
Tomato puree + GS |
|
Galloyl units |
|
GS |
Before baking |
After baking |
After mixing |
After heating |
Y, detected; nd, not detected. |
Dimer |
0 |
601.3 |
601.2 |
Y |
Y |
Y |
Y |
1 |
753.3 |
753.4 |
Y |
Y |
Y |
Y |
2 |
905.3 |
905.7 |
Y |
Y |
Y |
Y |
Trimer |
0 |
889.8 |
890.1 |
Y |
Y |
Y |
Y |
1 |
1041.9 |
1041.9 |
Y |
Y |
Y |
Y |
2 |
1194.0 |
1194.6 |
Y |
Y |
Y |
Y |
3 |
1346.1 |
1347.0 |
Y |
Y |
Y |
Y |
Tetramer |
0 |
1178.0 |
1178.9 |
Y |
Y |
Y |
Y |
1 |
1330.1 |
1331.9 |
Y |
Y |
Y |
Y |
2 |
1482.2 |
1483.1 |
Y |
Y |
Y |
Y |
3 |
1634.4 |
1634.9 |
Y |
Y |
Y |
Y |
4 |
1786.5 |
1787.1 |
Y |
Y |
Y |
Y |
Pentamer |
0 |
1466.3 |
1466.7 |
Y |
Y |
Y |
Y |
1 |
1618.4 |
1618.9 |
Y |
Y |
Y |
Y |
2 |
1770.5 |
1770.9 |
Y |
Y |
Y |
Y |
3 |
1922.6 |
1922.9 |
Y |
Y |
Y |
Y |
4 |
2074.7 |
2075.8 |
Y |
Y |
Y |
Y |
Hexamer |
0 |
1754.5 |
1755.2 |
Y |
Y |
Y |
Y |
1 |
1906.7 |
1907.4 |
Y |
Y |
Y |
Y |
2 |
2058.8 |
2059.4 |
Y |
Y |
Y |
Y |
3 |
2210.9 |
2211.4 |
Y |
Y |
Y |
Y |
4 |
2363.0 |
2365.9 |
Y |
nd |
Y |
nd |
5 |
2515.1 |
nd |
nd |
nd |
nd |
nd |
6 |
2667.2 |
nd |
nd |
nd |
nd |
nd |
Heptamer |
0 |
2042.8 |
2042.9 |
Y |
Y |
Y |
nd |
1 |
2194.9 |
2194.9 |
Y |
Y |
Y |
nd |
2 |
2347.0 |
2347.7 |
Y |
Y |
nd |
nd |
3 |
2499.1 |
2499.64 |
Y |
Y |
nd |
nd |
4 |
2651.2 |
2651.6 |
Y |
Y |
nd |
nd |
5 |
2803.3 |
nd |
nd |
nd |
nd |
nd |
6 |
2955.4 |
nd |
nd |
nd |
nd |
nd |
7 |
3107.5 |
nd |
nd |
nd |
nd |
nd |
Octamer |
0 |
2331.1 |
2331.5 |
Y |
nd |
nd |
nd |
1 |
2483.2 |
2483.2 |
Y |
nd |
nd |
nd |
2 |
2635.3 |
2635.0 |
Y |
nd |
nd |
nd |
3 |
2787.4 |
2788.4 |
Y |
nd |
nd |
nd |
4 |
2939.5 |
2939.8 |
Y |
nd |
nd |
nd |
5 |
3091.0 |
3092.8 |
Y |
nd |
nd |
nd |
6 |
3243.0 |
3244.0 |
Y |
nd |
nd |
nd |
7 |
3395.0 |
3395.7 |
Y |
nd |
nd |
nd |
Nonamer |
0 |
2619.3 |
2619.9 |
nd |
nd |
nd |
nd |
1 |
2771.4 |
2771.8 |
nd |
nd |
nd |
nd |
2 |
2923.5 |
2923.2 |
nd |
nd |
nd |
nd |
3 |
3075.6 |
3074.9 |
nd |
nd |
nd |
nd |
4 |
3227.7 |
3228.1 |
nd |
nd |
nd |
nd |
The grape skin enriched tomato puree and bread were analysed upon mixing and heat treatment to investigate the effect of the food matrix and thermal processing on phenolic solubility. Upon mixing of grape skins with both the food matrices, flavonol profile and solubility was not affected. In fact, these compounds were solubilized in the expected amounts based on the amount of grape skin added (not shown). On the other hand, in the samples extracted from both the fortified food matrices, a lower number of flavanol components was detected especially for the higher mass oligomers. Flavanol nonamers were not detected in the extract from the fortified dough (Fig. 2b) and flavanol nonamers, octamers and part of heptamers were not detected in the extract from unheated tomato puree (Fig. 2d). These results suggest that these higher mass proanthocyanidins are involved in stronger interactions with the food matrix than other grape skin phenolics, in agreement with the binding constants calculated for the interaction between phenolics and a model peptide.35 After thermal treatment (pasteurization for the tomato and baking for the bread), i.e. in the final food product, a further loss of higher mass oligomers was observed. Flavanol octamers were no longer detected in the fortified bread extract (Fig. 2c) and flavanol heptamers were no longer detected in the extract from the heated tomato puree (Fig. 2e). This latter result suggests that heat processing caused either partial degradation of proanthocyanidins or changes in their complexes with matrix components.
It is worth noticing that, absence of the higher mass oligomers from the MALDI-TOF-MS profile shown in Fig. 2 could also be due to a possible lower efficiency in ionization of these compounds under MS conditions of analysis. In particular, this effect could occur for the tomato extract where proanthocyanidins are likely present as ternary protein/pectin/proanthocyanin aggregates.36 To investigate this point, proanthocyanidin solubility was evaluated after mixing and heat treatment upon hydrolysis with n-butanol/HCL (Table 4). The amount of proanthocyanidins solubilized from the fortified foods was lower than the amount added. This result confirmed that part of the proanthocyanidin component was strongly bound to the food matrix. In fact, soluble proanthocyanidin recovery was 55% in the fortified tomato puree before microwave treatment and 25% in the fortified dough (Table 4). Proanthocyanidin solubility was higher in the tomato with respect to flat bread, most probably due to both a lower protein content and the presence of pectins, which can form ternary protein/polysaccharide/proanthocyanidin complexes with enhanced solubility.36 Soluble proanthocyanin content of the fortified tomato puree did not vary significantly after microwave treatment, while in the fortified bread it decreased further after baking.
Table 4 Oligomeric proanthocyanidin content (g per kg d.w.) of tomato puree and wheat flat bread formulated with white grape skins (GS), before and after heat treatment
Oligomeric proanthocyanidin content |
Data are the mean value ± SD. Different apices within the same model food indicate significant differences (LSD, p < 0.05). Percent recovery of oligomeric proanthocyanidins is reported in brackets. Proanthocyanidins were not found in the control unfortified model foods. |
Tomato puree + 3% GS
|
Before heating |
3.71a ± 0.11 (55%) |
After heating |
3.48a ± 0.02 (52%) |
Expected value |
6.70 |
Wheat flat bread + 10% GS
|
Before baking |
0.49b ± 0.03 (25%) |
After baking |
0.34a ± 0.03 (18%) |
Expected value |
1.93 |
The inhibitory activity towards both α-glucosidase and α-amylase of model fortified foods and controls was then investigated. As shown in Table 5, the control unfortified tomato puree and flat wheat bread displayed inhibitory activity. Upon addition of the grape skin ingredient, the increase in inhibitory activity was significant for both tomato puree and flat bread, despite low solubility of the high mass oligomers. On the other hand, the observed increase in percentage of inhibition was lower than the expected increase, calculated based on the amount of added grape skin ingredient, especially in the fortified flat bread, most probably due to the binding of the higher mass proanthocyanidin fraction of grape skin to the food matrix.
Table 5 Inhibition of α-glucosidase and α-amylase activities (%) by tomato puree and wheat flat bread and their formulations with white grape skins (GS)
Model food |
α-Glucosidase inhibition |
α-Amylase inhibition |
Data are the mean value ± SD. Phenolic extract of the model foods (25 mg f.w. mL−1) was tested in: (a) α-glucosidase assay containing 5 mg mL−1 of rat intestinal brush border proteins and 0.2 mM pNPG in of 50 mM phosphate buffer, pH 6.8, and (b): α-amylase assay containing 2 μM α-amylase and 0.2 mM pNPGP in 50 mM phosphate buffer, pH 6.8. Different apices within the same model food indicate significant differences (LSD, p < 0.05). Percent recovery of the inhibitory activity is reported in brackets. |
Tomato puree
|
19a ± 2 |
16a ± 2 |
Tomato puree + 3% GS
|
26b ± 2 (93%) |
26b ± 2 (63%) |
Expected inhibition |
28 |
41 |
Wheat flat bread
|
21a ± 2 |
22a ± 2 |
Wheat flat bread + 10% GS
|
34b ± 2 (67%) |
29b ± 2 (29%) |
Expected inhibition |
51 |
100 |
The results of this in vitro study cannot be directly extrapolated to the biological effects of phenolics, but can provide information on the nature of their binding with the food matrix. These interactions could negatively affect phenolic bioavailability. On the other hand, under simulated gastrointestinal conditions in vitro, it was demonstrated that in a simple phenolic–protein matrix, phenolics were progressively released from complexes during digestion and hence could become available to exert their biological effects.38 The metabolism of monomeric phenolics of grape occurs via a common pathway: the aglycones can be absorbed from the small intestine while compounds that are present in the form of esters or glycosides must be hydrolyzed by intestinal enzymes or by the colonic microflora before being absorbed and generally metabolized by glucuronidation, glycosylation and sulfation in the small intestine and in the liver.13 Proanthocyanidins, the prevalent grape phenolics, are stable during gastric transit, where depolymerization is negligible. It is reputed that the presence of proanthocyanidins in the small intestinal lumen would be sufficient to act as starch digestion enzyme inhibitors following the consumption of a proanthocyanidin-rich food.39 Then, proanthocyanidins reach the colon and are degraded into phenylvalerolactones and phenolic acids by the colon microbiota.39
Interestingly, studies carried out on animal models have demonstrated that both grape skin phenolic extracts (comprising monomeric flavonoids and proanthocyanidins) and proanthocyanidin-rich extracts can prevent the postprandial increase in the blood glucose level in vivo.14,15 Hence these compounds proved to be effective, even if there is still limited information on the effective dose of grape phenolics that is bioavailable.13
Conclusion
The current in vitro study demonstrated that grape skins rich in monomeric flavanols, monomeric flavonols and either galloylated and non-galloylated oligomeric proanthocyanidins (up to nonamers), could inhibit starch digestion enzymes more efficiently than acarbose. In real food products fortified with grape skins, namely tomato puree and wheat flat bread, enzyme inhibition increased with respect to the unfortified foods, despite partial decrease of solubility of higher mass proanthocyanidins. Proanthocyanidin solubility was lower in bread, most probably due to the formation of binary proanthocyanin/protein complexes, than in tomato puree where the possible formation of ternary proanthocyanidin/protein/pectin complexes can enhance solubility. This in vitro approach provided information on the molecular features that affect interactions between the food matrix and grape skin phenolics, which could assist in the design of functional food matrices aimed at improving the wellbeing of diabetic people. The overall results supported the possibility to control blood glucose levels using grape skin as a sustainable ingredient.
Abbreviations
GAE | Gallic acid equivalents |
I
50 GAE
| Concentration of total phenolics (μg GAE mL−1) that inhibits the enzymatic reaction by 50% |
p-NPG |
p-Nitrophenyl α-D-glucoside |
p-NPGP |
p-Nitrophenyl α-D-maltopentaoside |
Varieties:
AR | Arneis |
CH | Chardonnay |
ER | Erbaluce |
MO | Moscato bianco |
MT | Muller thurgau |
NA | Nascetta |
RI | Riesling |
Acknowledgements
Research supported by AGER (Project number 2010-2222).
References
- C. Nunes, E. Ferreira, V. Freitas, L. Almeida, R. M. Barbosa and J. Laranjinha, Food Funct., 2013, 4, 373–383 CAS.
- G. J. McDougall, F. Shpiro, P. Dobson, P. Smith, A. Blake and D. Stewart, J. Agric. Food Chem., 2005, 53, 2760–2766 CrossRef CAS PubMed.
- K. T. Kongstad, C. Ozdemir, A. Barzak, S. G. Wubshet and D. Staerk, J. Agric. Food Chem., 2015, 63, 2257–2263 CrossRef CAS PubMed.
- V. Lavelli, J. Agric. Food Chem., 2008, 56, 7194–7200 CrossRef CAS PubMed.
- Y. Narita and K. Inouye, J. Agric. Food Chem., 2009, 57, 9218–9225 CrossRef CAS PubMed.
-
C. M. Galanakis, in Food Waste Recovery: Processing Technologies and Industrial Techniques, ed. C. M. Galanakis, Elsevier Inc., London, U.K., 2015, pp. 1–392 Search PubMed.
- A. Girones-Vilaplana, D. A. Moreno and C. Garcıa-Viguera, Food Funct., 2014, 5, 764–772 CAS.
- J. Nsor-Atindana, F. Zhong and K. J. Mothibe, Food Funct., 2012, 3, 1044–1050 CAS.
- A. Altunkaya, R. V. Hedegaard, J. Harholt, L. Brimer, V. Gokmene and L. H. Skibsted, Food Funct., 2013, 4, 1647–1653 CAS.
- A. J. van der Goot, P. J. M. Pelgrom, J. A. M. Berghout, M. E. J. Geerts, L. Jankowiak, N. A. Hardt, J. Keijer, M. A. I. Schutyser, C. V. Nikiforidis and R. M. Boom, J. Food Eng., 2016, 168, 42–51 CrossRef.
- A. Teixeira, N. Baenas, R. Dominguez-Perles, A. Barros, E. Rosa, D. A. Moreno and C. Garcia-Viguera, Int. J. Mol. Sci., 2014, 15, 15638–15678 CrossRef CAS PubMed.
- M. José Jara-Palacios, D. Hernanz, M. L. Escudero-Gilete and F. J. Heredia, Food Res. Int., 2014, 66, 150–157 CrossRef.
- C. Stockley, P. L. Teissedre, M. Boban, C. Di Lorenzo and P. Restani, Food Funct., 2012, 3, 995–1007 CAS.
- S. Hogan, L. Zhang, J. Li, S. Sun, C. Canning and K. Zhou, Nutr. Metab., 2010, 71, 1–9 Search PubMed.
- S. M. da Silva, E. A. Koehnlein, A. Bracht, R. Castoldi, G. R. de Morais, M. L. Baesso, R. A. Peralta, C. G. M. de Souza, A. B. de Sá-Nakanishi and R. M. Peralta, Food Res. Int., 2014, 56, 1–8 CrossRef.
- S. Mildner-Szkudlarz, R. Zawirska-Wojtasiak, A. Szwengiel and M. Pacynski, Int. J. Food Sci. Technol., 2011, 46, 1485–1493 CrossRef CAS.
- V. Lavelli, P. S. C. Sri Harsha, L. Torri and G. Zeppa, Food Chem., 2014, 152, 162–168 CrossRef CAS PubMed.
- V. Lavelli, E. Pagliarini, R. Ambrosoli, J. L. Minati and B. Zanoni, Postharvest Biol. Technol., 2006, 40, 34–40 CrossRef CAS.
- V. Lavelli and M. C. Torresani, Food Chem., 2011, 125, 529–535 CrossRef CAS.
- V. Lavelli and C. Vantaggi, J. Agric. Food Chem., 2009, 57, 4733–4738 CrossRef CAS PubMed.
-
AOAC, Official Methods of Analysis, Association of Official Analytical Chemists, Washington, DC, 15th edn, 1990 Search PubMed.
- N. Guerrieri, L. Eynard, V. Lavelli and P. Cerletti, Cereal Chem., 1997, 74, 846–850 CrossRef CAS.
- F. V. M. Silva and P. Gibbs, Crit. Rev. Food Sci. Nutr., 2004, 44, 353–360 CrossRef CAS PubMed.
- P. S. C. Sri Harsha, V. Lavelli and A. Scarafoni, Food Chem., 2014, 156, 220–226 CrossRef CAS PubMed.
- P. S. C. Sri Harsha, C. Gardana, P. Simonetti, G. Spigno and V. Lavelli, Bioresour. Technol., 2013, 140, 263–268 CrossRef CAS PubMed.
- G. Williamson, Mol. Nutr. Food Res., 2013, 57, 48–57 CAS.
- A. S. Boath, D. Grussu, D. Stewart and G. J. McDougall, Food Dig., 2012, 3, 1–7 CrossRef CAS.
- Y. Q. Li, F. C. Zhou, F. Gao, J. S. Bian and F. Shan, J. Agric. Food Chem., 2009, 57, 11463–11468 CrossRef CAS PubMed.
- T. Liu, L. Song, H. Wang and D. A. Huang, J. Agric. Food Chem., 2011, 59, 9756–9762 CrossRef CAS PubMed.
- Q. Li, J. Chen, T. Li, C. Liu, Y. Zhai, D. J. McClements and J. Liud, Food Funct., 2015, 6, 3693–3701 CAS.
- A. Barret, T. Ndou, C. A. Hughey, C. Straut, A. Howell, Z. Dai and G. Kaletunc, J. Agric. Food Chem., 2013, 61, 1477–1486 CrossRef PubMed.
- P. Bandyopadhyay, A. K. Ghosh and C. Ghosh, Food Funct., 2012, 3, 592–605 CAS.
- W. Zhao, V. Iyer, F. P. Flores, E. Donhowe and F. Kong, Food Funct., 2013, 4, 899–905 CAS.
- T. M. Granato, F. Piano, A. Nasi, P. Ferranti, S. Iametti and F. Bonomi, J. Agric. Food Chem., 2010, 58, 11969–11976 CrossRef CAS PubMed.
- C. Poncet-Legrand, C. Gautier, V. Cheynier and A. Imberty, J. Agric. Food Chem., 2007, 55, 9235–9240 CrossRef CAS PubMed.
- S. I. Soares, R. M. Gonglaves, I. Fernands, N. Mateus and V. De Freitas, J. Agric. Food Chem., 2009, 57, 4352–4358 CrossRef CAS PubMed.
- J. M. Gambetta, S. E. P. Bastian, D. Cozzolino and D. W. Jeffery, J. Agric. Food Chem., 2014, 62, 6512–6534 CrossRef CAS PubMed.
- A. Oliveira and M. Pintado, Food Funct., 2015, 6, 3444–3453 CAS.
- M. J. Salvadó, E. Casanova, A. Fernández-Iglesias, L. Arola and C. Bladé, Food Funct., 2015, 6, 1053–1071 Search PubMed.
|
This journal is © The Royal Society of Chemistry 2016 |