DOI:
10.1039/C5FO01012H
(Paper)
Food Funct., 2016,
7, 434-444
The hypolipidemic effect and antithrombotic activity of Mucuna pruriens protein hydrolysates
Received
21st August 2015
, Accepted 8th October 2015
First published on 19th October 2015
Abstract
Hydrolysates and peptide fractions (PF) obtained from M. pruriens protein concentrates with commercial and digestive enzymatic systems were studied for their hypolipidemic and antithrombotic activities. Hydrolysates obtained with Pepsin–Pancreatin (PP) and their peptide fractions inhibited cholesterol micellar solubility with a maximum value of 1.83% in PP. Wistar rats were used to evaluate the hypolipidemic effect of hydrolysates and PF. The higher reductions of cholesterol and triglyceride levels were exhibited by PP and both peptide fractions <1 kDa obtained from PP and Alcalase®–Flavourzyme® hydrolysate (AF) at a dose of 15 mg kg−1 of animal weight. PF > 10 kDa from both hydrolysates showed the maximum antithrombotic activity with values of 33.33% for PF > 10 kDa from AF and 31.72% for PF > 10 kDa from PP. The results suggest that M. pruriens bioactive peptides with the hypolipidemic effect and antithrombotic activity might be utilized as nutraceuticals.
Introduction
Elevated concentrations of some lipids in the plasma have been associated with an increased risk of heart diseases in humans.1 These increases are elicited by changes in the lipid metabolism pathways induced by environmental and genetic factors leading to hyperlipidemia and hypercholesterolemia, which are not diseases but health conditions characterized by elevated serum lipids and cholesterol, respectively.2,3 Raised cholesterol is estimated to cause 2.6 million deaths (4.5% of total) and 29.7 million disability adjusted life years. Although several drugs have been developed to manage severe cases of hypercholesterolemia in humans, dietary and lifestyle modification approaches have been recommended for maintaining low blood levels of proatherogenic cholesterol.1 In addition, various research investigations have focused on the use of less toxic functional foods and nutraceuticals to treat and manage mild cases of hypercholesterolemia.4–8
Thrombosis is a serious pathological issue that causes high rates of morbidity and mortality. In the USA alone, 100
000 to 180
000 deaths occur annually because of venous thromboembolism. Thrombosis occurs as a result of excessive adhesion and aggregation of platelets due to the disruption, rupture and erosion of the atherosclerotic plaque. The suppression or the inhibition of platelet aggregation is believed to decrease the incidence of the disease.9 Antiplatelet agents act as metabolic inhibitors of platelet activation, and drugs such as aspirin and ticlopidine have some ability to prevent arterial thrombi during chronic use but do not have a predictable effect when given during acute arterial thrombosis.10 Food derived peptides that inhibit the blood platelet aggregation can be useful natural ingredients for the prevention of thrombosis.11 For instance, papain-hydrolyzed pork meat was found to inhibit platelet reactivity. In addition, a tetrapeptide, asp-gly-glu-ala (DGEA), derived from type I collagen was found to inhibit collagen-induced platelet aggregation.12
Ingestion of a fatty meal appears to cause venous endothelial dysfunction in healthy adults, while postprandial lipemia has been associated with transient changes in factor VII coagulant activity in humans, suggesting a temporary and reversible hypercoagulable state.13 Dyslipidaemia may exert an influence on the risk of venous thromboembolism, as determined by a recent meta-analysis in which patients with venous thromboembolism had high triglyceride and low HDL cholesterol levels, while no effect of total cholesterolaemia on venous thromboembolism was seen.14
Mucuna pruriens is a legume grown in Africa, South America and South Asia.15 It is rich in protein (23–35%) and has a nutritional quality comparable to that of other pulses like lima bean and has good potential as a cheap and alternate source of proteins.16 The aim of the present study was to evaluate the hypolipidemic effect and the inhibition of platelet aggregation of protein hydrolysates and ultrafiltered peptide fractions from Mucuna pruriens protein concentrates hydrolyzed with digestive and commercial sequential enzymatic systems.
Materials and methods
Seeds and chemicals
Pods of Mucuna pruriens were collected in Yucatan, Mexico. After drying the pods were thrashed to remove the seeds. Matured and dried seeds were stored in airtight plastic jars at room temperature. All chemicals were reagent grade or better and purchased from Sigma Chemical Co. (St Louis, MO, USA).
Protein concentrates
Selected grains were ground in a disk mill (model 4-E Quaker, Mill Straub Co., Philadelphia, PA, USA) and then sifted through 4.76 and 2.38 mm screens in order to remove the smallest particles. Hulls were removed with a fluidizing air bed and the material resulted was milled in a Cyclotec Mill (Tecator, Höganas, Sweden) until passing through a 0.841 mm screen. The protein concentrate of M. pruriens bean was obtained by wet fractionation.17
Enzymatic hydrolysis
Hydrolysis of the protein isolates was performed using a totally randomized design. Treatments were the sequential enzymatic system applied: Alcalase® 2.4L FG and Flavourzyme® 500MG (Novo Nordisk, Bagsvaerd, Denmark); or pepsin from porcine gastric mucosa (Sigma, P7000-100G) and pancreatin from porcine pancreas (Sigma, P3292-100G). The response variable was the degree of hydrolysis (DH). The hydrolysis with the AF system was performed according to Pedroche et al.18 The hydrolysis with the sequential PP system was performed according to Megías et al.19 The degree of hydrolysis was calculated by determining free amino groups with o-phthaldialdehyde:20 DH = h/htot × 100; where htot is the total number of peptide bonds per protein equivalent, and h is the number of hydrolyzed bonds.
Hydrolysate fractionation
The Alcalase®–Flavourzyme® (AFH) and pepsin–pancreatin (PPH) hydrolysates were fractionated by ultrafiltration21 with a high performance ultrafiltration cell (Model 2000, Millipore). The supernatants of AFH and PPH were collected. A part of them was studied as whole hydrolysates, and the rest was fractionated with a high performance ultrafiltration cell (Model 2000, Millipore). Five fractions were prepared using four molecular weight cut-off (MWCO) membranes: 1 kDa, 3 kDa, 5 kDa and 10 kDa. The protein content in ultrafiltered peptide fractions was quantified using the method of Lowry et al.22 Ultrafiltration-membrane-based separations of enzymatic hydrolysates can achieve the removal of peptides from non-hydrolyzed proteins and proteolytic enzymes. In addition, ultrafiltration can also be used to perform peptide separation according to their molecular mass and also their charge.
Protein and peptide patterns
Sodium dodecyl sulfate polyacrylamide gel electrophoresis (SDS-PAGE) was performed according to the method described by Schägger et al.23 using 15% (w/v) resolving polyacrylamide gel. Protein concentrates (i.e., control sample), hydrolysates and ultrafiltered peptide fractions were dissolved at 2 mg mL−1 in distilled water, then diluted to a final concentration of 1 mg mL−1 with a sample buffer containing β-mercaptoethanol and heated to 85 °C for 10 min. 10 μL of each sample and molecular weight markers, containing low molecular weight peptides and high molecular weight proteins, were loaded onto the gel. The analysis was run at a constant current of 25 mA. The gel was silver stained and de-stained according to Sorensen et al.24
In vitro cholesterol micellar solubility inhibition
The in vitro micellar solubility of cholesterol with hydrolysates and peptide fractions was measured according to the method described by Zhong et al.6 This assay consist of a micellar solution (7.0 mL) containing 10.0 mM sodium taurocholate, 2.0 mM cholesterol, 5.0 mM oleic acid, 132.0 mM NaCl, 15.0 mM sodium phosphate (pH 7.4), and 1.0 mL of hydrolysates or peptide fractions was prepared by sonication. The mixture was incubated at 37 °C for 24 h and ultracentrifuged at 100
000g for 60 min at 37 °C. The supernatant was collected for the determination of the total cholesterol content using a COBAS C 111 analyzer (Roche Diagnostics, Indianapolis, IN, USA).
Hypocholesterolemic effect in rats
The experimental procedures were approved by the Care and Use Committee of Laboratory Animals (Faculty of Medicine, Autonomous University of Morelos) and were performed according to the Official Mexican Norm (NOM-062-ZOO-1999) “Technical specifications for the care and use of laboratory animals” as well as all Federal and Institutional regulations. 8–10-week-old female Wistar alloxan-induced diabetic rats weighing 280 ± 40 g were used to evaluate the hypocholesterolemic effect of the following products: AFH, PPH, and peptide fractions <1 kDa (5, 10 and 15 mg kg−1). All the above-mentioned products were administered to the rats by intraperitoneal injection (IP), between 9 and 10 a.m. Five animals were used per treatment. Animals were housed in an air conditioned room (22–24 °C with a 12 h light cycle) and consumed tap water and a standard diet for rats (nu3lab, Research Global Solution, Burlington, Ontario, Canada) ad libitum during the experiments. To induce diabetes in rats a solution of alloxan at 2% diluted in physiological saline solution (PSS) (Sigma, USA H4034) at 0.9% was administered to the animals in a single dose corresponding to 40 mg of alloxan per kg of animal weight injected intraperitoneally. PSS served to dilute hydrolysates and as a positive control, and pravastatin sodium (0.5 mg kg−1 of animal weight), a known lipid-lowering compound which reduces cholesterol biosynthesis, as a negative control. The content of cholesterol was measured weekly for a period of four weeks.
In vitro inhibition of human platelet aggregation
The in vitro inhibition of human platelet aggregation was analyzed following Miyashita et al.25 Blood from human volunteers, diluted with 130 mM trisodium citrate (4.5 mL), was centrifuged at 127g for 15 min at room temperature in a Solbat J-600 centrifuge to give platelet-rich plasma (PRP) as the supernatant. After removing most of the PRP, the residual phase was centrifuged at 1918g for 15 min at room temperature to obtain platelet-poor plasma (PPP). Immediately, a platelet adjustment was done to the PRP (200 × 103 μL−1) using a hematic cytometer (Sysmex KX-21). PRP (450 μL) was incubated with each test compound, which was dissolved in saline (154 mM NaCl at pH 6.4) for 1 min at 37 °C. Platelet aggregation was induced by adding 10 μL of an aqueous solution of 10 μm ADP. Aggregation was measured by using an aggregometer (Chrono-Log 400) as the increase in the light transmission through PRP. PPP, which was used as the control, gave 100% transmission. The platelet aggregation inhibition percentage was determined to subtract the platelet aggregation percentage from the PRP with proteins hydrolyzed to the platelet aggregation percentage from the PRP under basal conditions.
Amino acid composition
Amino acid composition was determined for the ultrafiltered peptide fractions by high performance liquid chromatography.26 Samples (4 mg of protein) were treated with 4 mL of 6.0 N HCl, placed in hydrolysis tubes and gassed with nitrogen at 110 °C for 24 h. They were then dried in a rotavapor (Büchi, Rotavapor R-215, Flawil, Switzerland) and suspended in sodium borate buffer (1.0 M, pH 9.0). Derivatization was performed at 50 °C using diethyl ethoxymethylenemalonate. Amino acids were separated using HPLC with a reversed-phase column (300 × 3.9 mm, Nova Pack C18, 4 mm; Waters), and a binary gradient system with sodium acetate containing 25 mM (A) of 0.02 g L−1 sodium azide at pH 6.0, and (B) acetonitrile as solvents.
Statistical analysis
All results were analyzed using central tendency and dispersion measures. One-way ANOVA was run to evaluate protein isolate hydrolysis. A LSD multiple range test was used to determine differences between treatments and biological activities in hydrolysates and ultrafiltered peptide fractions. All analyses were performed according to Montgomery27 and processed with the Statgraphics Plus version 5.1 software.
Results and discussion
Hydrolysate fractionation
The protein content was determined in filtrates and retentates of both sequential enzymatic systems (Fig. 1). Some differences between the protein content and molecular weight distribution profiles of both hydrolysates were observed.
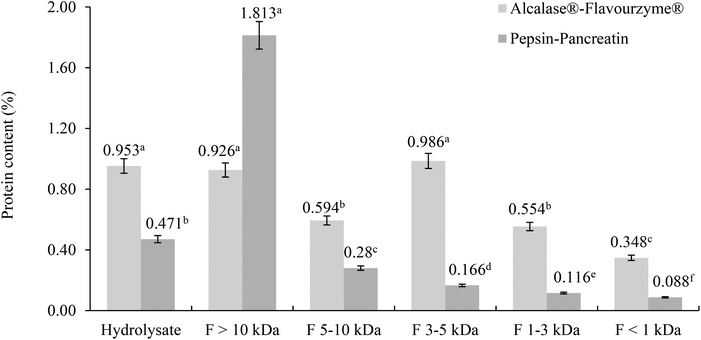 |
| Fig. 1 Peptide content (mg mL−1) in total hydrolysates and ultrafiltered peptide fractions obtained from M. pruriens protein concentrates hydrolyzed with Alcalase®–Flavourzyme® and pepsin–pancreatin. a–fDifferent letters in the same system indicate statistical difference (p < 0.05). | |
In the Alcalase®–Flavourzyme® system the content of protein did not differ between F >10 kDa and F 3–5 kDa, and between F 5–10 kDa and F 1–3 kDa, and the lowest content was observed in F <1 kDa. The hydrolytic activity of this enzymatic system generated peptides with a wide range of molecular weights.28–30 Protein hydrolysis with endoproteinases and exopeptidases like Alcalase® and Flavourzyme® is known to yield a large proportion of small peptides with molecular weight <2 kDa.31–33 For the pepsin–pancreatin system the highest content of protein was observed in the F >10 kDa and the lowest in the F <1 kDa. Although the hydrolytic activity of the pepsin–pancreatin enzymatic system was higher (40.15%) this produced primarily high molecular weight peptides >10 kDa and a little amount of low molecular weight peptides.
Protein and peptide patterns
The sodium dodecyl sulphate polyacrylamide gel electrophoresis (SDS-PAGE) pattern of Mucuna bean protein concentrates and hydrolysates are depicted in Fig. 2. The gel indicates the presence of five polypeptide protein sub-units of apparent molecular weights 200, 116, 82, 63, and 59 kDa. Similar ranges of polypeptide molecular weight distribution have also been reported for several other legume proteins.34
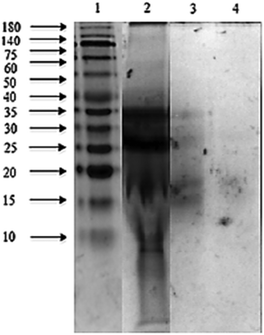 |
| Fig. 2 SDS-PAGE patterns of Mucuna pruriens protein concentrates and hydrolysates obtained with Alcalase®–Flavourzyme® and pepsin–pancreatin enzymatic systems. Lane 1: molecular weight markers (10–180 kDa); lane 2: M. pruriens protein concentrate; lane 3: Alcalase®–Flavourzyme® hydrolysate; lane 4: pepsin–pancreatin hydrolysate. | |
At the end of both hydrolysis, the majority of high molecular weight proteins (200–30 kDa) were hydrolyzed into peptides with a lower molecular size as shown in lanes 3 and 4 (Fig. 3). The electrophoresis pattern reveals higher hydrolysis efficiency for pepsin–pancreatin. These results agree with those observed in the degree of hydrolysis. The gel electrophoresis (SDS-PAGE) pattern of ultrafiltered peptide fractions are depicted in Fig. 3.
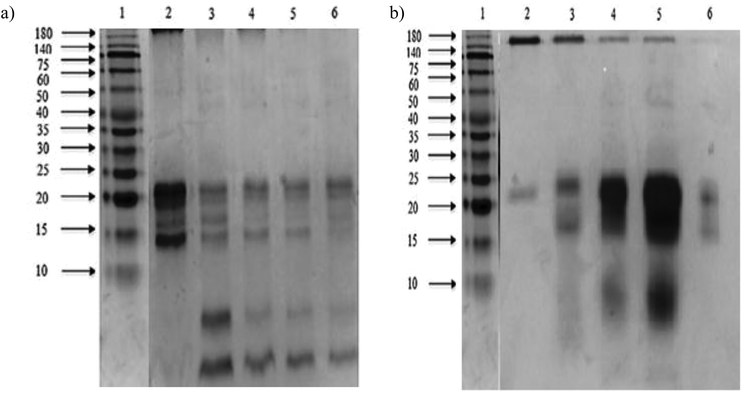 |
| Fig. 3 SDS-PAGE patterns of Mucuna pruriens ultrafiltered peptide fractions. (a) Ultrafiltered peptide fractions from the Alcalase®–Flavourzyme® system. Lane 1: molecular weight markers (10–180 kDa); lane 2: F > 10 kDa; lane 3: F 5–10 kDa; lane 4: F 3–5 kDa; lane 5: F 1–3 kDa; lane 6: F < 1 kDa. (b) Ultrafiltered peptide fractions from the pepsin–pancreatin system. Lane 1: molecular weight markers (10–180 kDa); lane 2: F > 10 kDa; lane 3: F 5–10 kDa; lane 4: F 3–5 kDa; lane 5: F 1–3 kDa; lane 6: F < 1 kDa. | |
The SDS-PAGE patterns of Alcalase®–Flavourzyme® ultrafiltered peptide fractions from F > 10 kDa to F < 1 kDa, respectively, showed that the intensity of some bands decreased after ultrafiltration steps. The same behavior was observed by pepsin–pancreatin ultrafiltration. The average molecular weight of protein hydrolysates is one of the most important factors, which determines their functional properties.35 An ultrafiltration membrane system could be a useful method for obtaining peptide fractions with a desired molecular size and enhanced biological activity. This system has been successfully applied in the fractionation and functional characterization of hydrolysates;36 and also as a first step in the isolation and further purification of bioactive peptides from similar sources.37,38 Under conditions studied in the present study the ultrafiltration process with different membranes could be used to partially concentrate peptides with a defined molecular weight range.
In vitro inhibition of cholesterol micellar solubility
Hydrolysates obtained with the sequential enzymatic system Alcalase®–Flavourzyme® and their peptide fractions did not exhibit hypocholesterolemic activity. Inhibition of in vitro cholesterol micelle solubility of hydrolysates obtained with the sequential enzymatic system pepsin–pancreatin and its ultrafiltered fractions are shown in Table 1.
Table 1
In vitro inhibition of human platelet aggregation and cholesterol micellar solubility of hydrolysates and ultrafiltered peptide fractions
In vitro biological activity |
Inhibition of human platelet aggregation (%) |
Inhibition of cholesterol micellar solubility (%) |
The data are expressed as means ± SEM (n = 3). Within a column, data with different superscript letters are significantly different. |
Enzymatic system |
AF |
PP |
PP |
Hydrolysate |
22.58d |
5.38d |
1.83e |
F > 10 kDa |
33.33f |
31.72e |
1.25d |
F 5–10 kDa |
2.15a |
0.54a |
1.12c |
F 3–5 kDa |
3.76b |
1.08b |
1.05b |
F 1–3 kDa |
4.84c |
5.38d |
1.12c |
F < 1 kDa |
3.22b |
2.15c |
0.53a |
The in vitro cholesterol micellar solubility inhibition of pepsin–pancreatin hydrolysates and ultrafiltered peptide fraction ranged between 1.83% (hydrolysate) and 0.53% (F <1 kDa). These values do not agree with those obtained by others researchers. Zhong et al.6 reported the cholesterol micellar solubility inhibition of hydrolyzed soy Alcalase protein and found that cholesterol was reduced by 48.6%. Also casein tryptic hydrolysates reduced the cholesterol micellar solubility by 20%.39 The in vitro inhibition of cholesterol micellar solubility is a strategy that has been used toward the search for potent hypolipidemic and hypocholesterolemic food proteins and peptides.6 However this method depends only on the reduction of cholesterol in the micellar solubility solution. Generally, proteins and peptides could exhibit hypocholesterolemic and hypolipidemic in vivo effects by means of increased cholesterol catabolism, reduced cholesterol synthesis or increased expression of LDL reception genes.28 Thus the in vitro evaluation of the hypocholesterolemic activity could not be directly correlated with the in vivo effect. Considering the above, both hydrolysates and their ultrafiltered peptide fractions obtained in the present study could exhibit the hypocholesterolemic effect in vivo.
Hypocholesterolemic and hypolipidemic effects in rats
Significant hyperlipidemia that characterized the diabetic state may therefore be regarded as a consequence of uninhibited actions of lipolytic hormones on the fat depots. The in vivo hypocholesterolemic activity of both hydrolysates and their ultrafiltered peptide fractions (F <1 kDa) was studied with female Wistar alloxan-induced diabetic rats. As shown in Table 2, after 1 week of administration of pravastatin sodium, hydrolysates and ultrafiltered peptide fractions, the controls and the samples exhibited hypocholesterolemic effects in rats. During the study, the hypocholesterolemic effect generated by the administration of hydrolysates and ultrafiltered peptide fractions was higher than the effect of the drug pravastatin sodium. The hypocholesterolemic effect of hydrolysates and peptide fractions depended on the dose delivered and was greater for peptide fractions.
Table 2 Serum cholesterol (C) and triglycerides (TG) levels in alloxan-induced diabetic rats during four weeks at doses of 5, 10 and 15 mg kg−1 of animal weight
|
After 1 week |
After 2 weeks |
After 3 weeks |
After 4 weeks |
Time (mg dL−1) |
C |
TG |
C |
TG |
C |
TG |
C |
TG |
The data are expressed as means (n = 4). Within a line, data with different superscript letters are significantly different. |
5 mg kg−1 of animal weight |
SPS |
159.50a |
476.00f |
162.25c |
245.50f |
162.75b |
336.00e |
153.75a |
130.25a |
Pravastatin sodium |
160.60a |
191.00c |
157.40a |
113.40b |
164.75c |
138.50b |
179.75d |
157.50b |
AF |
170.00b |
262.60e |
160.20b |
145.00d |
154.00a |
134.2b |
164.00c |
343.80f |
PP |
168.60b |
122.60a |
157.00a |
120.20c |
153.75a |
82.00a |
163.50c |
297.25e |
AF F < 1 kDa |
159.40a |
208.40d |
160.00b |
154.60e |
155.60a |
192.00d |
158.60b |
239.20d |
PP F < 1 kDa |
165.80b |
134.00b |
162.80c |
97.80a |
169.00d |
178.80c |
155.00a |
170.40c |
10 mg kg−1 of animal weight |
SPS |
159.50a |
476.00f |
162.25c |
245.50c |
162.75b |
336.00d |
153.75a |
130.25b |
Pravastatin sodium |
160.60b |
191.00a |
157.40b |
113.40a |
164.75b |
138.50a |
179.75d |
157.50c |
AF |
169.50c |
342.25d |
174.25d |
255.25d |
176.00c |
342.25e |
160.00b |
417.50d |
PP |
165.25c |
254.25b |
177.25d |
301.50f |
176.75c |
412.00f |
175.50c |
506.00e |
AF F < 1 kDa |
159.75a |
363.00e |
158.00b |
199.50b |
150.25a |
291.25c |
155.25a |
153.00c |
PP F < 1 kDa |
157.00a |
318.00c |
154.00a |
268.75e |
162.50b |
146.00b |
152.50a |
123.00a |
15 mg kg−1 of animal weight |
SPS |
159.50a |
476.00e |
162.25c |
245.50f |
162.75b |
336.00e |
153.75b |
130.25c |
Pravastatin sodium |
160.60a |
191.00c |
157.40b |
113.40a |
164.75b |
138.50b |
179.75d |
157.50d |
AF |
166.00b |
237.25d |
155.74a |
183.75d |
169.75c |
190.50d |
164.00c |
600.00e |
PP |
159.75a |
146.75a |
162.50c |
210.50e |
155.75a |
146.25c |
150.00a |
131.00c |
AF F < 1 kDa |
157.75a |
155.00b |
159.75b |
129.50b |
154.25a |
78.75a |
150.00a |
119.00b |
PP F < 1 kDa |
159.25a |
143.00a |
161.75c |
163.00c |
154.75a |
149.75c |
150.75a |
109.00a |
The hypocholesterolemic effect of hydrolysates and peptide fractions at a dose of 5 mg kg−1 of animal weight is shown in Fig. 4a. In general, the serum cholesterol content remained stable throughout the study period in rats IP administered with SPS. For pravastatin sodium the hypocholesterolemic effect decays from the second week at the dose evaluated. Hydrolysates and peptide fractions exhibited a higher hypocholesterolemic effect than pravastatin sodium. The highest reductions in the serum cholesterol content were observed at the end of the third week by the IP administration of both hydrolysates, and at the end of the fourth week the serum cholesterol content of animals administered with hydrolysates increased to reach a value close to that of the first week. Only PP < 1 kDa showed a tendency to reduce the serum cholesterol content in the fourth week.
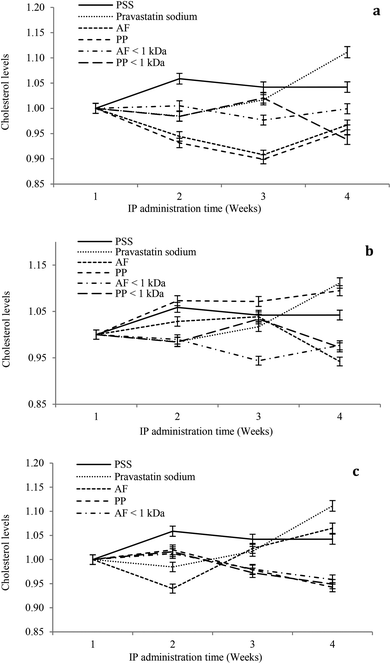 |
| Fig. 4 Serum cholesterol levels in alloxan-induced diabetic rats for four weeks of IP administration of hydrolysates and peptide fractions (a) 5 mg kg−1 of animal weight; (b) 10 mg kg−1 of animal weight; (c) 15 mg kg−1 of animal weight. Saline physiological solution (SPS); Alcalase®–Flavourzyme® hydrolysate (AF); pepsin–pancreatin hydrolysate (PP), Alcalase®–Flavourzyme® peptide fraction < 1 kDa (AF < 1 kDa); pepsin–pancreatin peptide fraction < 1 kDa (PP < 1 kDa). | |
For the dose of 10 mg kg−1 of animal weight the serum cholesterol content remained stable throughout the study period in rats IP administered with SPS. For pravastatin sodium the hypocholesterolemic effect decays from the second week at the dose evaluated (Fig. 4b). AF hydrolysates reduced the serum cholesterol content during the study period with the highest reduction at the end of the fourth week. In contrast, the PP hydrolysate showed high serum cholesterol contents during the study period with a similar content of pravastatin sodium at the end of the fourth week. From both peptide fractions only PP < 1 kDa showed a tendency to reduce the serum cholesterol content in the fourth week. At a dose of 15 mg kg−1 of animal weight the serum cholesterol content remained stable throughout the study period in rats IP administered with SPS. For pravastatin sodium the hypocholesterolemic effect decays from the second week at the dose evaluated (Fig. 4c). AF hydrolysates increased the serum cholesterol content after the second week of the study period with the highest content at the end of the fourth week. In contrast, the PP hydrolysate and both peptide fractions reduced the serum cholesterol content after the second week of the study period with the highest reduction at the end of the fourth week. In general, the higher reductions in serum cholesterol contents were observed after the second week of the study period at the three doses (5, 10 and 15 mg kg−1 of animal weight). Only at the high dose PP hydrolysates and peptide fractions increased the hypocholesterolemic effect after the second week to the end of the study period.
The hypolipidemic effect of hydrolysates and peptide fractions at a dose of 5 mg kg−1 of animal weight is shown in Fig. 5a. In general, the serum triglyceride content remained stable throughout the study period in rats IP administered with SPS. For pravastatin sodium the hypocholesterolemic effect decays from the second week and remains stable at the end of the study. The highest reductions in the serum triglyceride content were observed at the beginning of the third week by the IP administration of both hydrolysates. At the end of the study the hypolipidemic effects of both hydrolysates decay. The ultrafiltered peptide fractions exhibited the hypolipidemic effect only at the beginning of the second week and after that their effects decay until they reach similar values as SPS at the end of the study.
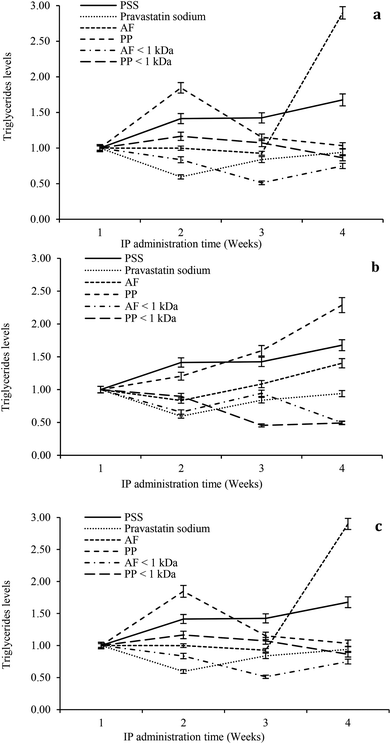 |
| Fig. 5 Serum triglycerides levels in alloxan-induced diabetic rats for four weeks of IP administration of hydrolysates and peptide fractions (a) 5 mg kg−1 of animal weight; (b) 10 mg kg−1 of animal weight; (c) 15 mg kg−1 of animal weight. Saline physiological solution (SPS); Alcalase®–Flavourzyme® hydrolysate (AF); pepsin–pancreatin hydrolysate (PP), Alcalase®–Flavourzyme® peptide fraction < 1 kDa (AF < 1 kDa); pepsin–pancreatin peptide fraction <1 kDa (PP < 1 kDa). | |
For the dose of 10 mg kg−1 of animal weight the serum triglyceride content remained stable throughout the study period in rats IP administered with SPS. For pravastatin sodium the hypocholesterolemic effect decays from the second week and remains stable at the end of the study (Fig. 5b). PP hydrolysates only exhibited a slight hypolipidemic effect at the beginning of the second week. In contrast, AF hydrolysates showed a reduction in the triglyceride content at the beginning of the second week with successive reductions in its hypolipidemic effect at weeks three and four. Both peptide fractions exhibited higher reduction of the serum triglyceride content than pravastatin sodium at the end of the study. At a dose of 15 mg kg−1 of animal weight the serum triglyceride content remained stable throughout the study period in rats IP administered with SPS. For pravastatin sodium the hypocholesterolemic effect decays from second week and remains stable at the end of the study (Fig. 5c). For three weeks the AF hypolipidemic effect remains stable and after that the effect is lost until the higher value of triglycerides is reached of all the study. PP hydrolysates reduced the serum triglyceride content after the second week and maintained a reducing trend throughout the study. Both peptide fractions reduced the serum triglyceride content after the second week of the study period with the highest reduction at the end of the fourth week. In general, the higher reductions in the serum triglyceride content were observed after the second week of the study period at the three doses (5, 10 and 15 mg kg−1 of animal weight). At a dose of 10 mg kg−1 of animal weight both peptide fractions exhibited the highest hypolipidemic effect.
The present study showed the hypocholesterolemic and hypolipidemic effects of Mucuna pruriens hydrolysates and ultrafiltered peptide fractions. Other studies that examined the effect of plant proteins and their hydrolysates also showed the hypolipidemic effect.40,41 The suggested mechanisms for this hypolipidemic effect are the excretion of fat by the hydrophobicity of hydrolysates itself or peptides made during the ingestion process and the changes of hepatic enzymes related to lipid metabolism. Because a high concentration of serum cholesterol and triglycerides is a key factor for cardiovascular risk, the results suggested that the hydrolysates and the ultrafiltered peptide fractions were an effective hypocholesterolemic and hypolipidemic peptide mixture, and the serum cholesterol and triglyceride lowering effect of hydrolysates and ultrafiltered peptide fractions was dosage and time dependent.
Inhibition of human platelet aggregation
The peptide fractions obtained with the AF and PP sequential system showed significant inhibition of ADP-induced aggregation of human platelets in platelet-rich plasma (Table 1). The maximum inhibitory effect of protein hydrolysates obtained with the AF and PP systems was 33.33 and 31.72% in F > 10 kDa at a concentration of 0.9269 and 1.8130 mg mL−1, respectively that corresponds to a moderate antithrombotic activity which is similar to the activity of synthetic antithrombotic compounds (aspirin and indomethacin). Probably the most active ultrafiltered peptide fraction is F > 10 kDa from hydrolysate AF because it needs less protein concentration than F > 10 kDa from hydrolysate PP to cause the same inhibition. Previous studies have identified three distinct fibrinogen-derived peptides that are inhibitors of platelet aggregation. The amino acid sequences of these peptides form RGD, which recurs twice in the fibrinogen α chain; HHLGGAKQAGDV, the carboxyl terminus of the fibrinogen γ-chain; and GPRP. RGD-containing peptides and γ-chain-related peptides appear to inhibit platelet aggregation by binding to the platelet receptor complex GPIIb/IIIa and blocking adhesive glycoprotein attachment. GPRP binds directly to the fibrinogen D domain and inhibits the ability of fibrinogen to bind to GPI1b/IIIa. GPRP is also able to inhibit fibrin gel formation via binding to the D domain of fibrinogen. Agents that interfere with fibrinogen and von Willebrand factor binding to GPIIb/IIIa or with von Willebrand factor binding to GPIb have already been proven as effective antithrombotics in in vivo studies. Using models in which platelet-dependent thrombus formation occurred after coronary artery constriction, various investigators have demonstrated inhibition of thrombosis after infusion of a monoclonal antibody that binds to GPIIb/IIIa or by peptides that contained the RGD sequence. An antibody that bound to the von Willebrand factor and prevented its attachment to activated platelets also inhibited arterial thrombus formation. Similarly, the agent aurintricarboxylic acid, which binds to the von Willebrand factor and prevents its attachment to GPIb, has been demonstrated in vivo to be a potent inhibitor of platelet-dependent thrombus formation within constricted arteries. Inhibition of platelet aggregation by direct blockade of platelet-adhesive glycoprotein interactions is a potentially effective means to prevent or arrest acute arterial thrombus formation.11
Amino acid composition
During hydrolysis, asparagine and glutamine partially converted to aspartic acid and glutamic acid, respectively; the data for asparagine and/or aspartic acid were therefore reported as Asx while those for glutamine and/or glutamic acid were reported as Glx. The results of this study suggest that Mucuna pruriens protein hydrolysates and ultrafiltered peptide fractions could contain peptides that inhibit platelet aggregation in plasma and exhibit both in vitro and in vivo hypolipidemic effects (Table 3).
Table 3 Amino acid composition of hydrolysates and ultrafiltered peptide fractions
Amino acid |
Alcalase®–Flavourzyme® |
Pepsin–pancreatin |
Hydrolysate |
F > 10 kDa |
F < 1 kDa |
Hydrolysate |
F > 10 kDa |
F < 1 kDa |
The data are expressed as means (n = 3). Within a line, data with different superscript letters are significantly different. |
Asp |
19.33 |
16.56 |
20.03 |
17.71 |
15.12 |
14.35 |
Glu |
10.89 |
13.50 |
12.51 |
12.87 |
13.32 |
13.77 |
Ser |
8.66 |
17.47 |
14.80 |
14.33 |
2.38 |
7.89 |
His |
10.87 |
4.85 |
5.32 |
5.22 |
9.09 |
3.47 |
Gly |
7.13 |
7.85 |
5.02 |
4.98 |
7.98 |
4.52 |
Thr |
8.20 |
7.72 |
7.89 |
8.60 |
13.28 |
5.79 |
Arg |
4.27 |
4.54 |
4.75 |
5.28 |
6.15 |
7.67 |
Ala |
2.89 |
3.10 |
3.67 |
4.09 |
4.23 |
4.89 |
Pro |
1.01 |
0.59 |
0.81 |
0.66 |
0.65 |
0.22 |
Tyr |
5.55 |
4.27 |
4.24 |
3.84 |
3.88 |
2.40 |
Val |
5.26 |
0.00 |
3.07 |
2.94 |
2.42 |
1.57 |
Met |
0.00 |
0.00 |
0.00 |
0.00 |
0.00 |
0.00 |
Cys |
3.90 |
5.11 |
4.50 |
4.30 |
3.91 |
6.91 |
Ile |
2.93 |
4.69 |
4.26 |
4.27 |
3.68 |
8.29 |
Trp |
0.67 |
0.41 |
0.54 |
0.51 |
0.29 |
0.26 |
Leu |
2.21 |
2.22 |
1.32 |
2.43 |
2.30 |
2.39 |
Phe |
1.69 |
1.02 |
0.89 |
0.80 |
2.31 |
8.06 |
Lys |
4.53 |
6.10 |
6.38 |
7.17 |
9.00 |
7.55 |
Amino acid distribution (%) |
Hydrophobic |
16.66d |
12.03a |
14.56b |
15.70c |
15.89c |
25.68e |
Hydrophilic |
52.49e |
47.31c |
47.63c |
46.06b |
51.66d |
43.78a |
Neutral |
30.85a |
40.67d |
37.81c |
38.24c |
32.45b |
30.54a |
The ability of a peptide to inhibit platelet aggregation can be linked to its amino acid composition and its primary sequence. Laudano and Doolittle42 demonstrated that peptides comprising gly-pro-arg (G-P-R) at the beginning of their sequences have the ability to bind fibrinogen and prevent fibrin monomer polymerization. Different studies related the inhibition of platelet aggregation by non-food derived peptides (i.e., snake venom) to the presence of the tripeptide arg-gly-asp (R-G-D) which blocks the binding of fibrinogen to the activated platelet receptors.43 Milk, egg and rapeseed derived peptides have been reported to possess antithrombotic properties.12 Shimizu et al.9 suggested that the antithrombotic activity is probably associated with the higher contents of Ile, Leu and Phe. Ultrafiltered peptide fractions F > 10 kDa from both hydrolysates that exhibited the highest values of inhibition of human platelet aggregation presented in their amino acid composition high amounts of amino acids reported in sequences of peptides with antithrombotic activity.
Hydrophobicity plays a major role in the hypocholesterolemic activity of peptides especially in binding bile acids. The hydrophobic amino acids of the hypocholesterolemic peptides and proteins are thought to interact with bile acids by hydrophobic interactions leading to the formation of insoluble complexes for fecal removal.28 Kongo-Dia-Moukala et al.44 suggest that hydrophobic amino acids contained in hydrolysates favor its immersion in the lipid micelles. Other factors such as amino acid sequences of peptides may also contribute to cholesterol-lowering activity. Kritchevsky et al.45 considered that a high arginine–lysine ratio in food proteins might contribute to an increased cholesterol-lowering ability. This hypothesis was supported by the hypocholesterolemic activity reported for fish protein hydrolysates, which had a high arginine–lysine ratio when compared to casein.31 Kwon et al.46 isolated a tetrapeptide with the amino acid sequence (Leu-Pro-Tyr-Pro) from soy glycinin. They reported that the hypocholesterolemic effect of this peptide was related to a Leu residue at the N terminus. In this study hydrolysates and their peptide fractions contain high amounts of hydrophobic amino acids but did not show a significant in vitro inhibition of cholesterol micellar solubility. However hydrolysates and peptide fractions exhibited hypocholesterolemic and hypolipidemic effects in alloxan induced diabetes. In this sense the amino acid composition could be more related to the biological activity than hydrophobicity.
Conclusions
The in vitro inhibition of human platelet aggregation and cholesterol micellar solubility of the ultrafiltered peptide fractions was significantly higher than those of the non-fractionated hydrolysates. Intraperitoneal administration of PP and the ultrafiltered peptide fractions AF < 1 kDa and PP < 1 kDa at a dose of 15 mg kg−1 of animal weight decreases the serum cholesterol content in alloxan-induced diabetic rats during a period of four weeks. For triglycerides, intraperitoneal administration of ultrafiltered peptide fractions AF < 1 kDa and PP < 1 kDa at a dose of 10 mg kg−1 of animal weight decreases the serum triglyceride content in alloxan-induced diabetic rats during a period of four weeks. The findings from the present study suggest that hydrolysis of Mucuna pruriens proteins with sequential enzymatic systems combined to ultrafiltration fractionation of hydrolysates could provide new opportunities for the development of health-promoting ingredients. Although it is necessary to evaluate the antithrombotic in vivo effect of the hydrolysates and their ultrafiltered peptide fractions and determine the sequences of the peptides present in the ultrafiltered fractions, the results of this study may provide a model by which to develop new antithrombotic, hypocholesterolemic and hypolipidemic strategies such as the development of physiologically functional foods or therapeutic agents aimed at preventing and/or treatment of thrombosis and dyslipidemias.
Acknowledgements
This research forms part of Project 154307 “Investigación científica dirigida al desarrollo de derivados proteínicos de Mucuna pruriens con potencial actividad biológica para la prevención y/o tratamiento de enfermedades crónicas asociadas al sobrepeso y la obesidad”, financed by te Consejo Nacional de Ciencia y Tecnología (CONACYT).
References
- B. Fletcher, K. Berra, P. Ades, L. T. Braun, L. E. Burke, J. L. Durstine, J. M. Fair, G. F. Fletcher, D. Goff, L. L. Hayman, W. R. Hiatt, N. H. Miller, R. Krauss, P. Kris-Etherton, N. Stone, J. Wilterdink and M. Winston, Managing abnormal blood lipids: a collaborative approach, Circulation, 2005, 112(20), 3184–3209 CrossRef PubMed.
- C. Kirana, P. F. Rogers, L. E. Bennett, M. Y. Abeywardena and G. S. Patten, Naturally derived micelles for rapid in vitro screening of potential cholesterol-lowering bioactives, J. Agric. Food Chem., 2005, 5, 4623–4627 CrossRef PubMed.
- A. Tirosh, A. Rudich, T. Shochat, D. Tekes-Manova, E. Isreali, Y. Henkin, I. Kochba and I. Shai, Changes in triglyceride levels and risk of coronary heart disease in young men, Ann. Intern. Med., 2007, 147, 377–385 CrossRef.
- M. R. Lovati, C. Manzoni, E. Gianazza, A. Arnoldi, E. Kurowska and K. K. Carroll, Soy protein peptides regulate cholesterol homeostasis in Hep G2 cells, J. Nutr., 2000, 130, 2543–2549 CAS.
- J. H. Oh and Y. S. Lee, Hypolipidemic effects of peptide fractions of casein on serum lipids in rats fed normal or high fat diet, J. Korean Soc. Food Sci. Nutr., 2002, 31, 263–270 CrossRef.
- F. Zhong, J. Liu, J. Ma and C. F. Shoemaker, Preparation of hypocholesterol peptides from soy protein and their hypocholesterolemic effect in mice, Food Res. Int., 2007, 40, 661–667 CrossRef CAS PubMed.
- Z. Y. Chen, R. Jiao and K. Y. Ma, Cholesterol-lowering nutraceuticals and functional foods, J. Agric. Food Chem., 2008, 56, 8761–8773 CrossRef CAS PubMed.
- C. Megías, J. Pedroche, M. M. Yust, M. Alaiz, J. Girón-Calle, F. Millán and J. Vioque, Sunflower protein hydrolysates reduce cholesterol micellar solubility, Plant Foods Hum. Nutr., 2009, 64, 86–93 CrossRef PubMed.
- M. Shimizu, N. Sawashita, F. Morimatsu, J. Ichikawa, Y. Taguchi, Y. Ijiri and J. Yamamoto, Antithrombotic papain-hydrolyzed peptides isolated from pork meat, Thromb. Res., 2009, 123, 753–757 CrossRef CAS PubMed.
- G. Piazza, A. Seddighzadeh and S. Z. Goldhaber, Heart failure in patients with deep vein thrombosis, Am. J. Cardiol., 2008, 101, 1056–1059 CrossRef PubMed.
- B. Adelman, C. Gennings, J. Strony and E. Hanners, Synergistic inhibition of platelet aggregation by fibrinogen-related peptides, Circ. Res., 1990, 67, 941–947 CrossRef CAS PubMed.
- Z. Khiari, D. Rico, C. Barry-Ryan and A. B. Martin-Diana, Structure elucidation of ACE-inhibitory and antithrombotic peptides isolated from mackerel skin gelatine hydrolysates, J. Sci. Food Agric., 2013, 94(8), 1663–1671 CrossRef PubMed.
- J. G. Ray and F. R. Rosendaal, The role of dyslipidemia and statins in venous thromboembolism, Curr. Controlled Trials Cardiovasc. Med., 2001, 2, 165–170 CrossRef CAS.
- E. Previtali, P. Bucciarelli, S. M. Passamonti and I. Martinelli, Risk factors for venous and arterial thrombosis, Blood Transfus., 2011, 9, 120–138 Search PubMed.
- I. E. Ezeagu, B. Maziya-Dixon and G. Tarawali, Seed characteristics and nutrient and antinutrient composition of 12 Mucuna accessions from Nigeria, J. Trop. Subtrop. Agroecosyst., 2003, 1, 129–140 Search PubMed.
- P. Gurumoorthi, M. Pugalenthi and K. Janardhanan, Nutritional potential of five accessions of a South Indian tribal pulse, Mucuna pruriens var utilis II. Investigations on total free phenolics, tannins, trypsin and chymotrypsin inhibitors, phytohaemagglutinins, and in vitro protein digestibility, J. Trop. Subtrop. Agroecosyst., 2003, 1, 153–158 Search PubMed.
- D. Betancur-Ancona, S. Gallegos-Tintoré and L. Chel-Guerrero, Wet fractionation of Phaseolus lunatus seeds: partial characterization of starch and protein, J. Sci. Food Agric., 2004, 84, 1193–1201 CrossRef CAS PubMed.
- J. Pedroche, M. M. Yust, J. Girón-Calle, M. Alaiz, F. Millán and J. Vioque, Utilisation of chickpea protein isolates for production of peptides with angiotensin I-converting enzyme (ACE)-inhibitory activity, J. Sci. Food Agric., 2002, 82, 960–965 CrossRef CAS PubMed.
- C. Megías, M. M. Yust, J. Pedroche, H. Lquari, J. Girón-Calle, M. Alaiz, F. Millán and J. Vioque, Purification of an ACE inhibitory peptide after hydrolysis of sunflower (Helianthus annuus L.) protein isolates, J. Agric. Food Chem., 2004, 52, 1928–1932 CrossRef PubMed.
- P. Nielsen, D. Petersen and C. Dambmann, Improved method for determining food protein degree of hydrolysis, J. Food Sci., 2001, 66, 642–646 CrossRef CAS PubMed.
- M. J. Cho, N. Unklesbay, F. Hsieh and A. D. Clarke, Hydrophobicity of bitter peptides from soy protein hydrolysates, J. Agric. Food Chem., 2004, 52(19), 5895–5901 CrossRef CAS PubMed.
- O. H. Lowry, N. J. Rosebrough, L. Farr and R. J. Randall, Protein measurement with the Folin Phenol Reagent, J. Biol. Chem., 1951, 193, 267–275 Search PubMed.
- H. Schägger, Tricine-SDS-PAGE, Nat. Protoc., 2006, 1, 16–22 CrossRef PubMed.
- B. K. Sorensen, P. Hojrup, E. Ostergard, C. S. Jørgensen, J. Enghild, L. R. Ryder and G. Houen, Silver staining of proteins on electroblotting membranes and intensification of silver staining of proteins separated by polyacrylamide gel electrophoresis, Anal. Biochem., 2002, 304, 33–41 CrossRef CAS PubMed.
- M. Miyashita, M. Akamatsu, H. Ueno, Y. Nakagawa, K. Nishimura, Y. Hayashi, Y. Sato and T. Ueno, Structure–activity relationships of RGD mimetics as fibrinogen-receptor antagonists, Biosci., Biotechnol., Biochem., 1999, 63(10), 1684–1690 CrossRef CAS PubMed.
- M. Alaiz, J. L. Navarro, J. Giron and E. Vioque, Amino acid analysis by high performance liquid chromatography after derivatization with diethylethoxymethylenemalonate, J. Chromatogr., 1992, 591, 181–186 CrossRef CAS.
-
D. C. Montgomery, Diseño y análisis de experimentos, 2da Ed. México, D. F., Editorial Limusa S. A. de C. V., 2007, pp. 21–141 Search PubMed.
-
C. C. Udenigwe and R. E. Aluko, Hypolipidemic and Hypocholesterolemic Food Proteins and Peptides, in Bioactive Food proteins and Peptides, ed. N. S. Hettiarachchy, CRC Press Taylor & Francis Group, Boca Raton, FL, 2012, p. 348 Search PubMed.
- C. A. Prieto, A. Guadix, P. González-Tello and E. M. Guadix, A cyclic membrane reactor for the hydrolysis of whey protein, J. Food Eng., 2007, 78(1), 257–265 CrossRef PubMed.
-
B. Keil, Specificity of proteolysis, Springer-Verlag, NewYork, 1992, pp. 336–569 Search PubMed.
- H. Wergedahl, B. Liaset, O. A. Gudbrandsen, E. Lied, M. Espe, Z. Muna, S. Mork and R. K. Berge, Fish protein hydrolysate reduces plasma total cholesterol, increases the proportion of HDL cholesterol, and lowers acyl-CoA: Cholesterol acyltransferase activity in liver of Zucker rats, J. Nutr., 2004, 134, 1320–1327 CAS.
- P. Bertrand, C. P. Ting, Y. Mine, L. R. Juneja, T. Okubo, S. F. Gauthier and Y. Pouliot, Comparative composition and antioxidant activity of peptide fractions obtained by ultrafiltration of egg yolk protein enzymatic hydrolysates, Membranes, 2011, 1, 149–161 CrossRef PubMed.
- E. A. Pena-Ramos, Y. L. Xiong and G. E. Arteaga, Fractionation and characterization for antioxidant activity of hydrolyzed whey protein, J. Sci. Food Agric., 2008, 84, 1908–1918 CrossRef PubMed.
- K. O. Adebowalea and O. S. Lawalb, Foaming, gelation and electrophoretic characteristics of Mucuna bean (Mucuna pruriens) protein concentrates, Food Chem., 2003, 83, 237–246 CrossRef.
- P. Park, W. Jung, K. Nam, F. Shahidi and S. Kim, Purification and characterization of antioxidative peptides from protein hydrolysate of lecithin-free egg yolk, J. Am. Oil Chem. Soc., 2001, 78(6), 651–656 CrossRef CAS.
- L. Lin and B. Li, Radical scavenging properties of protein hydrolysates from Jumbo flying squid (Dosidicus eschrichitii Steenstrup) skin gelatin, J. Sci. Food Agric., 2006, 86(14), 2290–2295 CrossRef CAS PubMed.
- S. Kim, Y. Kim, H. Byun, K. Nam, D. Joo and F. Shahidi, Isolation and characterization of antioxidative peptides from gelatin hydrolysate of Alaska pollack skin, J. Agric. Food Chem., 2001, 49(4), 1984–1989 CrossRef CAS PubMed.
- E. Mendis, N. Rajapakse, H. Byun and S. Kim, Investigation of jumbo squid (Dosidicus gigas) skin gelatin peptides for their in vitro antioxidant effects, Life Sci., 2005, 77(17), 2166–2178 CrossRef CAS PubMed.
- S. Nagaoka, Y. Futamura, K. Miwa, T. Awano, K. Yamauchi and Y. Kanamaru, Identification of novel hypocholesterol peptides derived from bovine milk β-lactoglobulin, Biochem. Biophys. Res. Commun., 2001, 281, 11–17 CrossRef CAS PubMed.
- A. A. Abd El-Baky, Clinicopathological effect of Camellia sinensis extract on streptozotocin-induced diabetes in rats, World J. Med. Sci., 2013, 8(3), 205–211 Search PubMed.
- T. Aoyama, K. Fukui, K. Takamatsu, Y. Hashimoto and T. Yamamoto, Soy protein isolate and its hydrolysate reduce body fat of dietary obese rats and genetically obese mice (yellow KK), Nutrition, 2000, 16, 349–354 CrossRef CAS.
- A. P. Laudano and R. F. Doolittle, Synthetic peptides derivatives that bind to fibrinogen and prevent the polymerization of fibrin monomers, Proceedings of the Natural Academy of Sciences USA, 1978, 75, 3085–3089 CrossRef CAS.
- J. R. Sheu, C. H. Lin, J. L. Chung, C. M. Teng and T. F. Huang, Triflavin, an Arg-Gly-Asp containing antiplatelet peptide inhibits cell-substratum adhesion and melanoma cell-induced lung colonization, Jpn. J. Cancer Res., 1992, 83, 885–893 CrossRef CAS PubMed.
- J. U. Kongo-Dia-Moukala, J. Nsor-Atindana and H. Zhang, Hypocholesterolemic activity and characterization of protein hydrolysates from defatted corn protein, Asian J. Biochem., 2011, 6, 439–449 CrossRef CAS.
- D. Kritchevsky, S. A. Tepper, S. K. Czarnecki and D. M. Klurfeld, Atherogenicity of animal and vegetable protein: Influence of the lysine to arginine ratio, Atherosclerosis, 1982, 41, 429–431 CrossRef CAS.
- D. Y. Kwon, S. W. Oh, J. S. Lee, H. J. Yang and S. H. Lee, Amino acid substitution of hypocholesterolemic peptide originated from glycinin hydrolyzate, Food Sci. Biotechnol., 2002, 11, 55–61 CAS.
|
This journal is © The Royal Society of Chemistry 2016 |