Emerging investigators series: silica-crosslinked graphene oxide membrane and its unique capability in removing neutral organic molecules from water
Received
10th March 2016
, Accepted 11th May 2016
First published on 12th May 2016
Abstract
This study reports the synthesis and physicochemical properties of a silica-crosslinked graphene oxide (GO) membrane, and highlights its unique capability to remove neutral organic molecules from water. The silica-crosslinked GO membrane was formed by soaking a layer-stacked GO film in saturated silica solution and remained stable under various test conditions. Comparison of the chemical compositions of the GO membrane before and after silica crosslinking indicates that silica mainly reacted with the carboxyl groups in GO. The GO membrane was negatively charged and found to remove the tested neutral organic molecules (glucose and sucrose) (∼84% to 90%) much more efficiently than the tested negatively charged ionic species (trisodium citrate/TSC) (∼22%). This unique separation behavior is quite interesting, as it is generally known that a charged nanofiltration or reverse osmosis membrane would remove charged species much better than neutral molecules of comparable sizes. Our experimental evidence suggests that neither size exclusion nor ionic strength is responsible for such unique behavior. Characterization of the interactions between the GO membrane and the tested neutral organic species indicates that the low partitioning and irreversible adsorption of neutral organic molecules into the GO membrane (much less than that of charged ionic species) is most likely responsible for their better removal by the GO membrane.
Water impact
Graphene-based membranes have recently emerged as a next generation of high-performance, energy-efficient membranes for water purification and wastewater reuse. Yet different synthesis methods as well as the resulting nanostructure and chemical compositions may significantly affect their separation performance. This research focuses on making a new graphene-based membrane with silica crosslinkers and studying its exceptional removal capability toward neutral water contaminants.
|
Introduction
Graphene oxide (GO) nanosheets, which consist of a network of aliphatic 6-membered rings decorated with epoxide, carboxyl, and hydroxyl groups,1–3 offer perfect two-dimensional building blocks to construct water filtration membranes with a layered, tunable nanostructure.4,5 Recent studies on such GO membranes have shown that water molecules can slip through the GO nanochannels at a fast rate while undesired particles are successfully rejected by size exclusion, charge repulsion, and/or intermolecular interactions.5–8
One of the major challenges in the preparation of layered GO membranes for water-related applications is the stabilization of GO layers in aqueous solution.5 So far, two major strategies exist for overcoming the potential dispersion of hydrophilic GO in an aqueous environment. The first strategy is the layer-by-layer (LbL) assembly via electrostatic interactions.9–12 At the current development stage, LbL-assembled GO membranes exhibit a water permeability in the range of 1 to 6 L m−2 h−1 bar−1,9–12 and thus they are useful as the separation layer of nanofiltration (NF) membranes10 or the surface modification layer of reverse osmosis membranes.13 Because of the dominating electrostatic interactions within the LbL-assembled layers, the GO membrane is often susceptible to swelling in high ionic-strength solutions due to the charge screening effect.14,15 Consequently, the selectivity of such membranes can be impaired by the environmental conditions such as pH and ionic strength of a feed solution.13
The other stabilization strategy is to create covalent bonding between GO nanosheets. After stronger and more stable covalent bonds are introduced, the resulting covalently crosslinked GO membranes are expected to possess improved stability and consistent separation capability under various environmental conditions, compared to the electrostatically stabilized GO membranes. Organic species such as trimesoyl chloride (TMC)5 and poly(vinyl alcohol) (PVA)16,17 have been successfully used to crosslink GO membranes. Recently, inorganic species have emerged as a more stable crosslinker due to the high-strength bonding between the oxygenated functional groups and inorganic oxides.18–20 Among various inorganic crosslinkers, silica is a particularly appealing candidate thanks to its low cost, high hydrophilicity, and good reactivity with the oxygenated functional groups in GO.21,22 For example, a silica-GO composite can be made by seeding and condensing the hydrolysis products of tetraethyl orthosilicate onto the GO plane.23 However, the resulting silica-GO nanocomposite membrane consists of agglomerated blocks rather than a layered structure that is ideal for water filtration. Today, it remains unknown whether silica can be effectively used as an inorganic crosslinker to create water-filtration GO membranes with a desirable layer-stacking structure.
Despite the abundant studies on GO membranes aiming to demonstrate their satisfactory removal of a wide array of water contaminants, relatively there is a lack of mechanistic understanding on the separation capability enabled by the unique properties of GO-formed nanochannels. For example, as a carbon-based material, GO nanosheets are able to interact with a variety of organic molecules,24–26 potentially making the GO membrane possess unique mechanisms for removing organic molecules from water. To the best of our knowledge, however, the removal of organic molecules by GO membranes and the corresponding unique separation mechanisms have not been reported in the literature.
In this study, we explored the use of silica as an effective inorganic crosslinker to synthesize a layer-stacked GO membrane. The physicochemical properties of the silica-crosslinked GO membrane were characterized using various techniques. The separation capability of the GO membrane was tested in a pressurized membrane system using representative organic species. The partitioning and adsorption of organic species into the GO membrane was investigated to understand the unique membrane separation mechanism.
Materials and methods
Chemicals
The chemicals for membrane synthesis and testing included graphite, NaNO3, KMnO4, H2SO4, HCl, Na2SiO3, NaCl, trisodium citrate (TSC), sucrose, glucose, and poly(allylanmine hydrochloride) (PAH). All chemicals were used as received from Sigma-Aldrich (St. Louis, MO) unless noted otherwise. GO was prepared from graphite using modified Hummers method with a detailed procedure described in our earlier publication.5
Preparation of GO membranes
The GO membrane was synthesized on a commercial polyethersulfone (PES) membrane support (Sterlitech, Kent, WA), which was 120 μm thick and negatively charged due to the presence of sulfonated groups. Prior to any treatment, the PES support was thoroughly rinsed with DI water to remove any contaminants from packing and transportation. To prepare the GO membrane, the negatively charged PES membrane support was first soaked in 1 g L−1 PAH solution at pH 4 with gentle shaking to create a positively charged surface for subsequent GO deposition. After 30 min of soaking, the membrane support was taken out and rinsed thoroughly with DI water to remove extra PAH. Then, a GO film was deposited on top of the PAH-treated PES membrane support by simple vacuum filtration of a pH 3 GO solution through the membrane. The thickness of the GO membrane was controlled by varying the volume of filtered GO solution from 1 to 5 mL. The GO membrane was subsequently soaked in a saturated Na2SiO3 solution (38 wt%) for crosslinking and stabilization. After 12 h of crosslinking, the GO membrane was rinsed thoroughly with DI water to fully remove any residual silicate solution. Next, the silica-crosslinked GO membrane was transferred into a 10% H2SO4 solution for further stabilization. Finally, the fabricated GO membrane was stored in DI water at room temperature until characterization and performance testing.
Membrane permeability and rejection tests
The GO membrane was tested for its pure water permeability and rejection of different organic molecules and ionic species, using a pressurized membrane filtration system. To start the test, the GO membrane was first mounted at the bottom of an Amicon™ cell (Millipore, Billerica, MA), which was connected to a feed tank that was pressurized by a nitrogen gas cylinder to supply feed water to the membrane cell, which was equipped with a stirrer to minimize the concentration polarization. The mass of the permeate was automatically measured by a digital balance (Denver Instruments, Denver, CO) and recorded by a personal computer using data acquisition software (Winwedge, Taltech, Philadelphia, PA), which monitored the water flux of the membrane. To stabilize the membrane against any compaction effect, all membranes were pressurized under a trans-membrane pressure of 50 psi (3.4 bar) for 24 h before testing.
The removal of organic molecules by the GO membrane was evaluated using two neutral organic molecules (glucose and sucrose) and one negatively charged organic species (TSC) as representative species. Before each rejection test, the membrane was first stabilized in the system for 2 h by saturating its adsorption capacity. Both feed and permeate samples were collected to determine the removal rate. The concentration of TSC samples was determined by conductivity measurement (Accumet Excel XL30, Thermo Scientific, Marietta, OH). The concentration of neutral organic molecules was determined by a total organic carbon analyzer (TOC-5000, Shimadzu, Columbia, MD). Triplicate experiments were conducted for all rejection tests.
Quartz crystal microbalance with dissipation (QCM-D) experiment
QCM-D (E-4, Q-Sense, Sweden) was used to evaluate the partitioning and adsorption of organic molecules by the GO membrane. In order to prepare a GO-coated QCM-D sensor that would fully mimic the structure and properties of the GO membrane, the following steps were carried out. As illustrated in Fig. 1a, a gold sensor (14 mm in diameter) was firstly exposed to PAH (1 g L−1, pH 4) in an open module to convert the negative charged gold surface to a positive charged one. Then, 1 mL of a pH 3 GO suspension was vacuum filtrated on a PES membrane substrate to deposit a GO thin film. The PAH-treated gold sensor was placed on the GO film with the gold surface facing down. The membrane substrate and the gold sensor were then dried in a 60 °C oven for 15 min to enhance the bonding between the sensor and GO film. After the sensor was peeled off from the PES substrate, a GO film was transferred from the PES substrate to the sensor surface. Finally, a saturated Na2SiO3 solution was introduced to crosslink the GO film. The frequency change caused by GO deposition and silica crosslinking was modeled by Sauerbrey equation using the software package provided by Q-sense to obtain the mass of GO and silica. As shown in Fig. 1b, the deposited mass of GO and mass change caused by the crosslinking process were around 17 μg cm−2 and 5 μg cm−2, respectively.
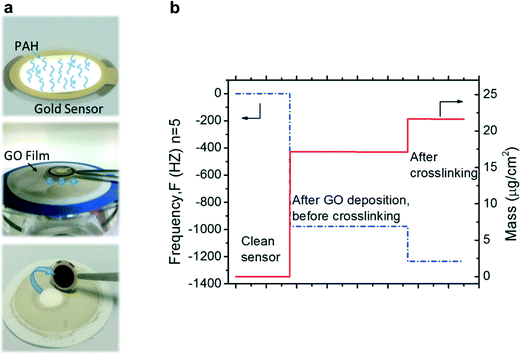 |
| Fig. 1 (a) The procedure to prepare a GO-coated QCM-D sensor. (b) Changes in the frequency and mass of a sensor during GO deposition and silica crosslinking. | |
The GO-coated sensor was installed in the QCM-D chamber to probe the partitioning and adsorption. The partitioning was measured by flowing glucose (100 mg L−1 of glucose with and without 20 mM NaCl, respectively) or TSC (3.3 mM) solution across the sensor surface. The irreversible adsorption was measured after the partitioning experiment by replacing the glucose or TSC solution with DI water.
GO and membrane characterization
Transmission electron microscopy (TEM) images were taken using a JEOL JEM 2100 LaB6 TEM (Peabody, MA) operated at 200 kV. The selected area diffraction (SAD) pattern was recorded under the diffraction mode of the same TEM. An energy-dispersive X-ray spectroscopy (EDS) system was also used to characterize the elemental composition of GO with the same TEM. The surface morphology and cross-sectional structure of the PES membrane and GO membranes were characterized by scanning electron microscopy (SEM) (SU-70, Hitachi High Technologies America, Gaithersburg, MD). Fourier transform infrared (FTIR) spectroscopy (Nicolet 6700, Thermo Scientific, Marietta, OH) was used to monitor the structural evolution during the membrane fabrication. The zeta potential of GO was measured by a Zetasizer Nano-ZSP analyzer (Malvern Instruments, UK). X-ray photoelectron spectroscopy (XPS) spectra were obtained using Kratos AXIS 165 photoelectron spectroscopy (Kratos Analytical, UK).
Results and discussion
Oxidation degree and charge of GO nanosheets
In order to understand the effects of GO nanosheet properties on the synthesis and performance of GO membranes, the oxidation degree and charge of GO nanosheets were characterized. The TEM images (Fig. 2a) of GO nanosheets exhibit single-layer carbon sheets with large areas of amorphous structure, indicating a high degree of oxidation. Nonetheless, regions with an aligned carbon lattice representing an unoxidized sp2 structure of graphene can still be observed at a higher magnification (Fig. 2b). The crystallinity of GO nanosheets was further characterized using SAD. As shown in Fig. 2c, the patterned diffraction ring confirms the presence of unoxidized carbon crystal structure, although the graphitic plane was mostly oxidized. Note that such mixed presence of oxidized and unoxidized regions is crucial for effective water filtration by a GO membrane. This is because the oxygenated functional groups help create the needed interlayer spacing for water molecules to pass through, while the unoxidized carbon lattice provides an ultrafast water path.4,27 The carbon-to-oxygen (C/O) ratio of GO was quantified by the EDS measurement using TEM. As shown in Fig. 1d, the C/O ratio was estimated as 61/39, which is consistent with results from previous studies.28,29 Owing to such a high content of oxygenated functional groups (i.e., carboxyl, hydroxyl, and epoxide),30,31 the GO nanosheets carried strong negative charge over a wide pH range (Fig. 2e).
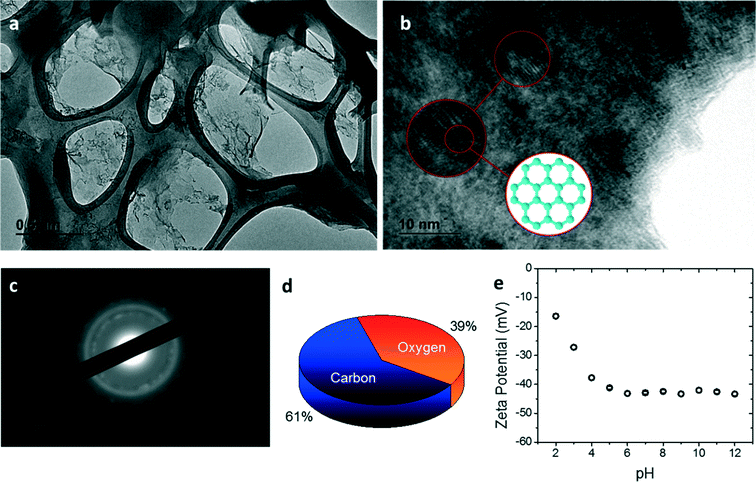 |
| Fig. 2 GO nanosheets: (a–b) transmission electron microscopy images at different magnifications, (c) selected area diffraction pattern, (d) elemental composition by energy-dispersive spectroscopy mapping, and (e) zeta potential. | |
Membrane synthesis and properties
The GO membrane was prepared on top of a PES membrane support that had an average pore diameter of ∼300 nm (Fig. 3a), too large to facilitate the formation of an intact polyamide-based selective layer on top but suitable for the deposition of a GO thin film.32 The PES support was first pretreated with a positively charged PAH solution to create a positively charged surface for the subsequent deposition of negatively charged GO. As shown in Fig. 3b, the PES surface was fully covered by a uniform GO thin film after filtering ∼1 mL GO solution (1 mg mL−1 at pH 3) through the substrate. Note that the relatively low pH of 3 was used to create an optimum environment for subsequent silica crosslinking, considering that silica gelation tends to form a more porous structure at a low pH.33 As illustrated in Fig. 3c, a gelation process was initiated in the GO channels, after the GO membrane was soaked in a saturated Na2SiO3 solution. After ∼12 h gelation, the silica-crosslinked GO membrane was transferred into a 10% H2SO4 solution for further stabilization. The silica crosslinking of a GO membrane is a critical step because the as-deposited GO layers are highly unstable and tend to disperse when soaked in an aqueous solution.5
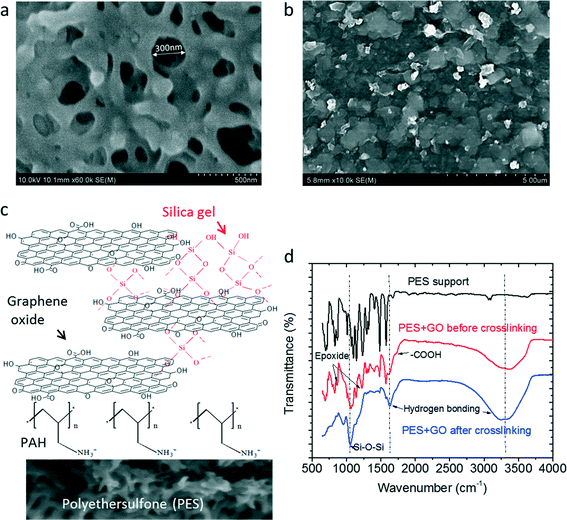 |
| Fig. 3 Silica-crosslinked GO membrane: SEM images of the membrane top surface (a) before and (b) after GO deposition, (c) schematic of reaction, and (d) FTIR spectra at different synthesis steps. | |
The chemical composition of the GO membrane at different synthesis steps was monitored by FTIR. As shown in Fig. 3d, after the deposition of GO on the PES support, new peaks appear at 1710 cm−1 for carboxyl groups, and at 856 cm−1 and 1223 cm−1 for epoxide groups,34 on top of the original peaks of PES. The subsequent silica crosslinking leads to a strong peak for the Si–O bonding at 1053 cm−1,35 which is absent from the spectra of the un-crosslinked GO membrane.
In order to understand the reaction mechanism responsible for the silica crosslinking of GO, XPS was employed to examine the GO membrane before and after crosslinking. As shown in Fig. 4a, the overall XPS spectra before crosslinking gives a C/O ratio of 68/32, which is slightly larger than the C/O ratio (61/39) of GO nanosheets obtained from EDS characterization. The increase of C/O ratio is likely due to the loss of a fraction of small, highly oxidized GO nanosheets during the vacuum filtration of GO solutions for making the membrane. The subsequent silica crosslinking increased the oxygen content as well as introduced emission peaks for Si2p at 102.9 eV and Si2s at 153.3 eV.36 Note that the relatively low silicon content (3%) after crosslinking indicates that silica mainly acted as a crosslinker between GO nanosheets rather than formed a thick gelation layer on top of GO (otherwise, the relatively shallow XPS penetration depth, normally a few nanometers, would have led to a much higher silicon content).
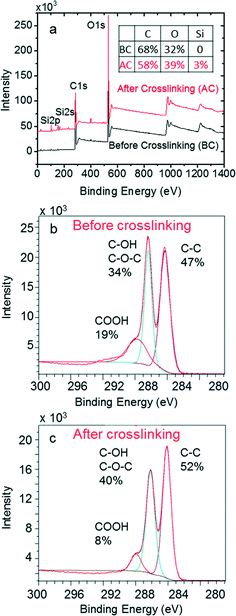 |
| Fig. 4 Properties of GO membrane surface: (a) XPS spectra before and after silica crosslinking, and chemical composition of C1s peaks (b) before and (c) after silica crosslinking. | |
We closely analyzed the C1s peaks before and after silica crosslinking. As shown in Fig. 4b, before the silica crosslinking, the C1s peak is composed of 47% unoxidized carbon (C–C), 34% single carbon–oxygen (C–O) bond (e.g., those in hydroxyl or epoxide groups), and 19% carbon–oxygen double bond (C
O) (e.g., carboxyl group), with the corresponding binding energy levels at 284.3 eV, 286.4 eV, and 288.1 eV, respectively.37 However, the silica crosslinking caused dramatic changes in the carbon peak composition, particularly a concentration decrease from 19% to 8% for the carbon–oxygen double bond and an increase from 34% to 40% for the single carbon–oxygen bond. Such changes indicate that during the crosslinking process, silica mainly reacted with the carboxyl groups in GO by most likely opening the C
O double bond in carboxyl groups and forming the C–O–Si bond between GO and the silica network.
The thickness of GO layers in the membrane was controlled by adjusting the volume of GO solution filtered onto the PES support. As seen in Fig. 5a, the color of the membrane turned from light brown to black after 1 mL, 2 mL, and 5 mL of GO suspension were deposited. The resulting GO membranes had a thickness of 70 nm, 130 nm, and 460 nm, respectively, as characterized by SEM cross-sectional imaging (Fig. 5b–d). As the GO film thickness increased, the permeability of the GO membrane decreased (Fig. 5e). The original PES membrane support had a pure water flux of 684 L m−2 h−1 bar−1, typical of an MF membrane. After the deposition of GO, the water flux dropped to 30, 19, and 6.4 L m−2 h−1 bar−1 for the 70 nm, 130 nm, and 460 nm-thick GO membranes, respectively. Such water fluxes of GO membranes are in general 2 to 3 times higher than the water flux of an NF membrane.38 The 130 nm-thick GO membrane (made with 2 mL of GO suspension) was selected for further characterization of its removal capability for organic molecules.
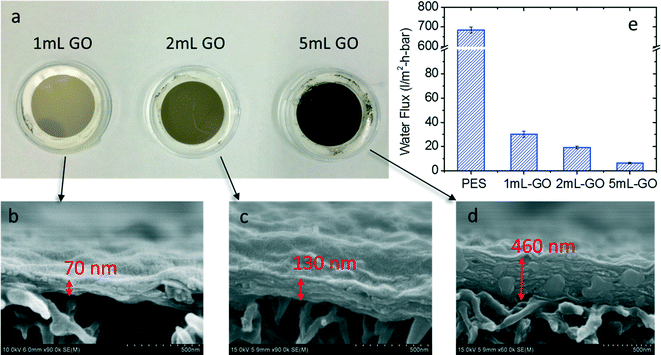 |
| Fig. 5 GO membranes made with different GO loadings: (a) surface photos, (b–d) SEM cross-sectional images, and (e) water fluxes compared to that of the PES membrane. | |
Unique removal capability for organic molecules
The GO membrane performance in removing organic molecules was tested with three organic species, namely TSC, glucose, and sucrose. All the permeation tests were conducted in a pressurized membrane system under a trans-membrane pressure of 20 psi, with the feed water at pH 7. The permeation tests were stabilized for 2 h to saturate the membrane adsorption capacity before taking feed and permeate samples, so that the removal capability due to GO adsorption was totally excluded. As listed in Fig. 6a, TSC has a molecular weight (MW) between those of glucose and sucrose. Unlike glucose and sucrose as neutral molecules, TSC is an ionic species that can dissociate into one citrate and three sodium ions.
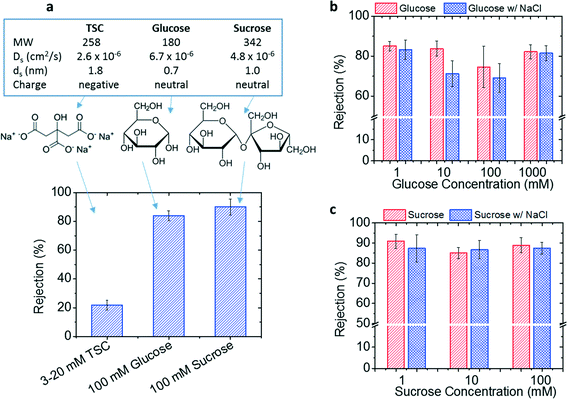 |
| Fig. 6 Removal of three organic species by the GO membrane: (a) properties and removal of the organic species, and removal of (b) glucose and (c) sucrose at various concentrations with and without 20 mM NaCl. All the permeation tests were performed in a cross-flow membrane system under a trans-membrane pressure of 20 psi and using a feed solution at pH 7. | |
The removal of the three selected organic molecules by the GO membrane is compared in Fig. 6a. There does not exist an apparent correlation between the removal rate and the molecular weight for these species—glucose, TSC, and sucrose have molecular weights of 180, 258, and 354, respectively, but their removal rates are 84%, 22%, and 90%, respectively. However, such poor correlation is not unusual because the transport properties (e.g., diffusivity) of a molecule could also affect its removal from water.38
We compared the diffusivity-derived size of the three molecules by calculating their individual Stokes diameter ds using eqn (1):
|  | (1) |
where
k is the Boltzmann constant,
T is the absolute temperature,
μ is the dynamic viscosity, and
Ds is the diffusivity in bulk solution. As listed in
Fig. 6a, TSC has the lowest diffusivity among the three species because it dissociates into charged counter ions, which hydrate with surrounding water molecules and hence diffuse very slowly.
39,40 As a result, the Stokes diameter of TSC (1.8 nm) is much larger than those of glucose (0.7 nm) and sucrose (1.0 nm). The fact that TSC has the largest diameter but the lowest removal rate indicates that the removal of organic species by the GO membrane cannot be well explained by diffusivity or size.
As we ruled out the effects of molecular weight and size on the different removal rates, the charge effect was examined next as another possible factor, which can introduce the Donan exclusion between the membrane surface and organic molecules and hence often plays a very important role in an NF membrane process.38 Among the three tested species, TSC is the only charged species, with each molecule dissociating into three positively charged sodium ions and one negatively charged citrate ion. The relatively large citrate ion carries three carboxylate groups with pKas at 3.1, 4.8, and 6.4, respectively, indicating that the majority of citrate ions have three negative charges at pH ∼ 7. It is expected that the removal of TSC is dominated by the removal of the relatively large, negatively charged citrate ions, so TSC is only labeled as a negatively charged species in Fig. 6a. The much lower removal rate for the negatively charged TSC (22%) than those for the two neutral molecules (glucose 84% and sucrose 90%) may suggest a possible charge effect. Typically, the charge effect in an NF membrane process enhances the removal of ionic species, which carry the same charge as that on the membrane surface, but not so for neutral molecules.38,41 However, this statement is inconsistent with the separation phenomenon we observed for the present GO membrane, which removed neutral molecules more effectively than ionic species.
We then investigated the role of ionic strength in governing the unique capability of the GO membrane to remove charged vs. neutral molecules. Research has shown that GO membranes could swell under high ionic strength and thus have larger sized transport channels, thereby potentially lowering the solute removal rate. Because a neutral molecule solution does not yield an ionic strength, we intentionally added NaCl into the glucose and sucrose solutions to create the same ionic strength as that of the TSC solution. As shown in Fig. 6b and c, the presence of an ionic strength did not appreciably decrease the removal rate for neutral molecules, indicating that the ionic strength and thus the associated membrane swelling may not be responsible for the better removal of neutral molecules than ionic species.
So far, the potential effects of size, charge, and ionic strength were all excluded, we hypothesize that the high removal rate for neutral molecules is attributed to the unique interaction between the organic molecules and the GO membrane, hindering the passage of organic molecules through the membrane. To test this hypothesis, we used QCM-D to quantitatively compare the partitioning and irreversible adsorption of glucose and TSC into the GO membrane. As shown in Fig. 7, after the GO-coated sensor was soaked in glucose and TSC solutions, respectively, the overall partitioning of glucose (less than 15 μg glucose per mg GO), with or without NaCl, into the GO membrane was much lower than that of TSC (around 600 μg TSC per mg GO). Then, we replaced the glucose or TSC solution by DI water, the residual (i.e., irreversible) adsorption of glucose or TSC was also measured in the GO sensor and is reported in Fig. 7. The irreversible adsorption of glucose (less than 5 μg glucose per mg GO) was again much lower than that of TSC (∼300 μg TSC per mg GO).
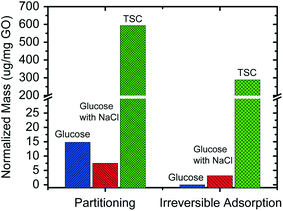 |
| Fig. 7 The partitioning and irreversible adsorption of glucose and TSC in the GO membrane measured by QCM-D. The partitioning of organic species from the solution into the GO membrane was measured by flowing glucose (100 mg L−1 of glucose with and without 20 mM NaCl) or TSC (3.3 mM) solution across the sensor surface. The irreversible adsorption was measured after the partitioning experiment by replacing the glucose or TSC solution with DI water. The deposited mass of organic species is normalized by the mass of the GO film on the sensor surface. | |
Such high partitioning and adsorption of TSC into the GO membrane is quite interesting because normally it is the repulsion instead of attraction that should exist between the negatively charged TSC molecules and the negatively charged GO membrane. There are two possible reasons to explain this phenomenon. First, TSC may enter the spaces between GO nanosheets and subsequently cause the GO membrane to swell under the resulting electrostatic repulsion. Second, the carboxyl groups in TSC may strongly bind to GO nanosheets or silica via hydrogen bonding. Research is ongoing to experimentally characterize the partitioning process in order to clearly understand the governing mechanism for the high partitioning of TSC. Additional organic molecules will also be tested to thoroughly study the effects of molecular size, shape, functional group, and charge on the partitioning of organic molecules into GO membranes.
Conclusions
We have successfully fabricated a silica-crosslinked graphene oxide (GO) membrane for water purification. We have reasonably attributed its high-efficient removal of neutral organic molecules to the low partitioning and irreversible adsorption of neutral organic molecules into the silica-crosslinked GO membrane. In comparison, the low removal rate for negatively charged organic species is mainly due to their high partitioning into the GO membrane.
Acknowledgements
This material is based upon work supported by the National Science Foundation under Grant no. CBET-1565452. The opinions expressed herein are those of the authors and do not necessarily reflect those of the sponsors.
References
- H. He, J. Klinowski, M. Forster and A. Lerf, A new structural model for graphite oxide, Chem. Phys. Lett., 1998, 287(1), 53–56 CrossRef CAS.
- A. Lerf, H. He, M. Forster and J. Klinowski, Structure of graphite oxide revisited, J. Phys. Chem. B, 1998, 102(23), 4477–4482 CrossRef CAS.
- Y. Zhu, S. Murali, W. Cai, X. Li, J. W. Suk, J. R. Potts and R. S. Ruoff, Graphene and graphene oxide: Synthesis, properties, and applications, Adv. Mater., 2010, 22(35), 3906–3924 CrossRef CAS PubMed.
- B. Mi, Graphene oxide membranes for ionic and molecular sieving, Science, 2014, 343(6172), 740–742 CrossRef CAS PubMed.
- M. Hu and B. Mi, Enabling graphene oxide nanosheets as water separation membranes, Environ. Sci. Technol., 2013, 47(8), 3715–3723 CrossRef CAS PubMed.
- R. K. Joshi, P. Carbone, F. C. Wang, V. G. Kravets, Y. Su, I. V. Grigorieva, H. A. Wu, A. K. Geim and R. R. Nair, Precise and ultrafast molecular sieving through graphene oxide membranes, Science, 2014, 343(6172), 752–754 CrossRef CAS PubMed.
- K. Huang, G. P. Liu, Y. Y. Lou, Z. Y. Dong, J. Shen and W. Q. Jin, A graphene oxide membrane with highly selective molecular separation of aqueous organic solution, Angew Chem., Int. Ed., 2014, 53(27), 6929–6932 CrossRef CAS PubMed.
- D. W. Boukhvalov, M. I. Katsnelson and Y. W. Son, Origin of anomalous water permeation through graphene oxide membrane, Nano Lett., 2013, 13(8), 3930–3935 CrossRef CAS PubMed.
- D. A. Dikin, S. Stankovich, E. J. Zimney, R. D. Piner, G. H. Dommett, G. Evmenenko, S. T. Nguyen and R. S. Ruoff, Preparation and characterization of graphene oxide paper, Nature, 2007, 448(7152), 457–460 CrossRef CAS PubMed.
- M. Hu and B. Mi, Layer-by-layer assembly of graphene oxide membranes via electrostatic interaction, J. Membr. Sci., 2014, 469, 80–87 CrossRef CAS.
- D. Li, M. B. Mueller, S. Gilje, R. B. Kaner and G. G. Wallace, Processable aqueous dispersions of graphene nanosheets, Nat. Nanotechnol., 2008, 3(2), 101–105 CrossRef CAS PubMed.
- Y. Gao, M. Hu and B. Mi, Membrane surface modification with tio2-graphene oxide for enhanced photocatalytic performance, J. Membr. Sci., 2014, 455, 349–356 CrossRef CAS.
- W. Choi, J. Choi, J. Bang and J. H. Lee, Layer-by-layer assembly of graphene oxide nanosheets on polyamide membranes for durable reverse-osmosis applications, ACS Appl. Mater. Interfaces, 2013, 5(23), 12510–12519 CAS.
- J. de Grooth, R. Oborný, J. Potreck, K. Nijmeijer and W. M. de Vos, The role of ionic strength and odd–even effects on the properties of polyelectrolyte multilayer nanofiltration membranes, J. Membr. Sci., 2015, 475, 311–319 CrossRef CAS.
- N. Schonbeck, K. Kvale, T. Demarcy, J. Giermanska, J.-P. Chapel and J.-F. Berret, Surfactant-triggered disassembly of electrostatic complexes probed at optical and quartz crystal microbalance length scales, Langmuir, 2014, 30(19), 5620–5627 CrossRef CAS PubMed.
- Y. Xu, W. Hong, H. Bai, C. Li and G. Shi, Strong and ductile poly (vinyl alcohol)/graphene oxide composite films with a layered structure, Carbon, 2009, 47(15), 3538–3543 CrossRef CAS.
- X. Zhao, Q. Zhang, Y. Hao, Y. Li, Y. Fang and D. Chen, Alternate multilayer films of poly (vinyl alcohol) and exfoliated graphene oxide fabricated via a facial layer-by-layer assembly, Macromolecules, 2010, 43(22), 9411–9416 CrossRef CAS.
- Z. An, O. C. Compton, K. W. Putz, L. C. Brinson and S. T. Nguyen, Bio-lnspired borate cross-linking in ultra-stiff graphene oxide thin films, Adv. Mater., 2011, 23(33), 3842–3846 CAS.
- K. I. Hsu, C. W. Lien, C. H. Lin, H. T. Chang and C. C. Huang, Immobilization of iron hydroxide/oxide on reduced graphene oxide: Peroxidase-like activity and selective detection of sulfide ions, RSC Adv., 2014, 4(71), 37705–37713 RSC.
- M. A. Worsley, T. Y. Olson, J. R. I. Lee, T. M. Willey, M. H. Nielsen, S. K. Roberts, P. J. Pauzauskie, J. Biener, J. H. Satcher and T. F. Baumann, High surface area, sp(2)-cross-linked three-dimensional graphene monoliths, J. Phys. Chem. Lett., 2011, 2(8), 921–925 CrossRef CAS PubMed.
- H. Jian, Z. Kaisong, W. Kun, X. Zongli, B. Ladewig and W. Huanting, Fabrication of polyethersulfone-mesoporous silica nanocomposite ultrafiltration membranes with antifouling properties, J. Membr. Sci., 2012, 423–424, 362–370 Search PubMed.
- J. Q. Dalagan and E. P. Enriquez, One-step synthesis of mesoporous silica-graphene composites by simultaneous hydrothermal coupling and reduction of graphene oxide, Bull. Mater. Sci., 2014, 37(3), 589–595 CrossRef CAS.
- H. Wu, B. Tang and P. Wu, Development of novel sio 2–go nanohybrid/polysulfone membrane with enhanced performance, J. Membr. Sci., 2014, 451, 94–102 CrossRef CAS.
- O. G. Apul, Q. Wang, Y. Zhou and T. Karanfil, Adsorption of aromatic organic contaminants by graphene nanosheets: Comparison with carbon nanotubes and activated carbon, Water Res., 2013, 47(4), 1648–1654 CrossRef CAS PubMed.
- Q. Peng, C. Liang, W. Ji and S. De, A first principles investigation of the mechanical properties of g-zno: The graphene-like hexagonal zinc oxide monolayer, Comput. Mater. Sci., 2013, 68, 320–324 CrossRef CAS.
- F. Wang, J. J.-H. Haftka, T. L. Sinnige, J. L. Hermens and W. Chen, Adsorption of polar, nonpolar, and substituted aromatics to colloidal graphene oxide nanoparticles, Environ. Pollut., 2014, 186, 226–233 CrossRef CAS PubMed.
- R. Joshi, P. Carbone, F. Wang, V. Kravets, Y. Su, I. Grigorieva, H. Wu, A. Geim and R. Nair, Precise and ultrafast molecular sieving through graphene oxide membranes, Science, 2014, 343(6172), 752–754 CrossRef CAS PubMed.
- O. C. Compton and S. T. Nguyen, Graphene oxide, highly reduced graphene oxide, and graphene: Versatile building blocks for carbon-based materials, Small, 2010, 6(6), 711–723 CrossRef CAS PubMed.
- H. C. Schniepp, J.-L. Li, M. J. McAllister, H. Sai, M. Herrera-Alonso, D. H. Adamson, R. K. Prud'homme, R. Car, D. A. Saville and I. A. Aksay, Functionalized single graphene sheets derived from splitting graphite oxide, J. Phys. Chem. B, 2006, 110(17), 8535–8539 CrossRef CAS PubMed.
- D. R. Dreyer, S. Park, C. W. Bielawski and R. S. Ruoff, The chemistry of graphene oxide, Chem. Soc. Rev., 2010, 39(1), 228–240 RSC.
- D. C. Marcano, D. V. Kosynkin, J. M. Berlin, A. Sinitskii, Z. Sun, A. Slesarev, L. B. Alemany, W. Lu and J. M. Tour, Improved synthesis of graphene oxide, ACS Nano, 2010, 4(8), 4806–4814 CrossRef CAS PubMed.
- M. Hu, S. Zheng and B. Mi, Organic fouling of graphene oxide membranes and its implications for membrane fouling control in engineered osmosis, Environ. Sci. Technol., 2016, 50(2), 685–693 CrossRef CAS PubMed.
- G. W. Sears Jr, Determination of specific surface area of colloidal silica by titration with sodium hydroxide, Anal. Chem., 1956, 28(12), 1981–1983 CrossRef.
- C. Chen, Q.-H. Yang, Y. Yang, W. Lv, Y. Wen, P.-X. Hou, M. Wang and H.-M. Cheng, Self-assembled free-standing graphite oxide membrane, Adv. Mater., 2009, 21(29), 3007–3011 CrossRef CAS.
- R. Al-Oweini and H. El-Rassy, Synthesis and characterization by ftir spectroscopy of silica aerogels prepared using several si (or) 4 and r′′ si (or′) 3 precursors, J. Mol. Struct., 2009, 919(1), 140–145 CrossRef CAS.
- T. L. Barr, An xps study of si as it occurs in adsorbents, catalysts, and thin films, Appl. Surf. Sci., 1983, 15(1), 1–35 CrossRef CAS.
- D. Yang, A. Velamakanni, G. Bozoklu, S. Park, M. Stoller, R. D. Piner, S. Stankovich, I. Jung, D. A. Field and C. A. Ventrice, Chemical analysis of graphene oxide films after heat and chemical treatments by x-ray photoelectron and micro-raman spectroscopy, Carbon, 2009, 47(1), 145–152 CrossRef CAS.
- B. Van der Bruggen, J. Schaep, D. Wilms and C. Vandecasteele, Influence of molecular size, polarity and charge on the retention of organic molecules by nanofiltration, J. Membr. Sci., 1999, 156(1), 29–41 CrossRef CAS.
- G. R. Ziegler, A. L. Benado and S. S. H. Rizvi, Determination of mass diffusivity of simple sugars in water by the rotating disk method, J. Food Sci., 1987, 52(2), 501–502 CrossRef CAS.
- J. Buffle, Z. Zhang and K. Startchev, Metal flux and dynamic speciation at (bio)interfaces. Part i: Critical evaluation and compilation of physicochemical parameters for complexes with simple ligands and fulvic/humic substances, Environ. Sci. Technol., 2007, 41(22), 7609–7620 CrossRef CAS PubMed.
- B. Mi, B. J. Marinas and D. G. Cahill, Rbs characterization of arsenic(iii) partitioning from aqueous phase into the active layers of thin-film composite nf/ro membranes, Environ. Sci. Technol., 2007, 41(9), 3290–3295 CrossRef CAS PubMed.
|
This journal is © The Royal Society of Chemistry 2016 |