DOI:
10.1039/C5DT02564H
(Paper)
Dalton Trans., 2016,
45, 1904-1917
P-chiral 1-phosphanorbornenes: from asymmetric phospha-Diels–Alder reactions towards ligand design and functionalisation†‡
Received
7th July 2015
, Accepted 30th July 2015
First published on 30th July 2015
Abstract
The principle of stereotopic face differentiation was successfully applied to 2H-phospholes which undergo a very efficient and highly stereoselective Diels–Alder reaction giving phosphorus-chiral 1-phosphanorbornenes with up to 87% yield. The observed reaction pathway has been supported by theoretical calculations showing that the cycloaddition reaction between 2H-phosphole 3a and the dienophile (5R)-(−)-menthyloxy-2(5H)-furanone (8) is of normal electron demand. Optically pure phosphanes were obtained by separation of the single diastereomers and subsequent desulfurisation of the sulfur-protected phosphorus atom. Finally, divergent ligand synthesis is feasible by reduction of the chiral auxiliary, subsequent stereospecific intramolecular Michael addition, and various functionalisations of the obtained key compound 13a. Furthermore, the unique structural properties of phospanorbornenes are presented and compared to those of phosphanorbornanes.
Introduction
In the beginning of enantioselective catalysis, ligands in which a chiral coordinating phosphorus atom bears the optical information were used.1 Today, the most widely applied ligands in asymmetric transition metal catalysis carry their optical information in the chiral backbone of the ligand.2 Because of the easy access to this type of molecule, C-chiral ligands have dominated the field of enantioselective catalysis until today. Nevertheless, tremendous efforts in recent years have furnished novel synthetic pathways towards P-chiral phosphanes and triggered a comeback of this class of ligands.3 Most of these synthetic pathways are based on resolution of racemates or diastereomers, kinetic and dynamic–kinetic resolution, and desymmetrisation.3 These methods have one common feature: they start from a phosphorus atom with tetrahedral or trigonal-pyramidal coordination. This is in contrast to the main method applied in the generation of C chirality – the principle of stereotopic face differentiation – which starts from a carbon atom with trigonal-planar coordination. Differences in the applied methods are due to different stabilities and accessibilities of the starting materials. Compounds with carbon or phosphorus in a tetrahedral or trigonal-pyramidal environment are stable and readily available, as are trigonal-planar carbon compounds (alkenes, ketones, singlet carbenes), whereas the corresponding phosphorus compounds (phosphaalkenes, singlet phosphinidenes) are often not stable or difficult to synthesise.4 Therefore, kinetic (sterically demanding groups) or thermodynamic stabilisation (delocalisation or coordination to a metal atom) is necessary to obtain such low-coordinate phosphorus species. The resulting compounds are often expensive and not readily available. Furthermore, their substitution pattern is less suitable for stereoselective synthesis. As a result, there have been only two examples in which the principle of stereotopic face differentiation has been applied. In both cases a chiral substituent at the trigonal-planar phosphorus atom was necessary to obtain optical induction.5 Recently, we contributed to this hardly explored field by applying a diastereoselective Diels–Alder reaction to 2H-phospholes.6 Even though these compounds with a planar-coordinated phosphorus atom in a five-membered ring are generated in situ, they are readily accessible and their chemistry is well understood.7 Herein, we present our detailed investigations on the diastereoselective Diels–Alder reaction of 2H-phospholes and further functionalisation of the obtained 1-phosphanorbornenes for divergent ligand design.
Results and discussion
The asymmetric Diels–Alder reaction of 2H-phospholes
In a first attempt to facilitate a Diels–Alder reaction of a 2H-phosphole, phospholide 1 was protonated in situ and the 2H-phosphole 3a trapped with the chiral dienophile 4 at low temperature to ensure good stereoselectivity (Scheme 1). Usually a dienophile like crotonic acid amide 4 is applied together with a Lewis acid to activate the dienophile and connect it with the chiral auxiliary.8
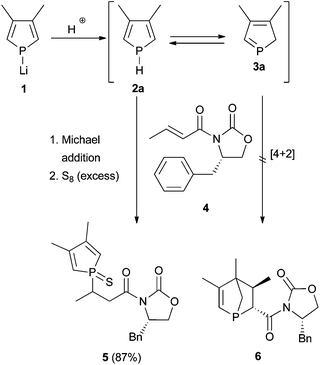 |
| Scheme 1 Protonation of phospholide 1 and subsequent reaction with 4. | |
As a Lewis acid would also interact with the phosphorus atom, deactivating the diene for cycloaddition reactions, the Diels–Alder reaction was performed without adding a Lewis acid. When acetic acid, sulfuric acid, or water were used as protonating reagent, an inseparable product mixture was obtained; using propan-2-ol gave the diastereomerically pure compound 5 (absolute configuration was not determined). Other products or isomers could not be isolated; hence, the de of the crude product could not be determined. Obviously, a Michael addition occurred, probably base-catalysed by the generated lithium isopropanolate. Thus, the synthetic route via protonation of 1 did not seem to furnish the targeted 1-phosphanorbornene 6.
We, therefore, switched to SiMe3, as this group is known to migrate in a [1,5]-sigmatropic shift at low temperatures as well.7a,931P{1H} NMR spectroscopic investigations in which the temperature was varied over time revealed that an equilibrium between monomeric 1H-phosphole (−51.5 ppm, s) 2b and only one dimer 7 exists at low temperature (Fig. 1). Monomeric 1H-phosphole 2b rearranges by a [1,5]-sigmatropic shift to 2H-phosphole 3b, which cannot be observed in NMR experiments due to its fast dimerisation to give 7. Only one isomer of the dimer (−49.6 ppm, −26.2 ppm, d, 1JP,P = 202.6 Hz) is formed, and the coupling constant suggests a P–P bond.7b,d Increasing the temperature shifts the equilibrium towards 2b. At room temperature a different isomer of the dimer is formed, and this suggests a kinetically favoured isomer at low temperatures. This system seemed to be suitable for stereoselective Diels–Alder reactions for two reasons: the fast equilibrium between 2b and 7 provides the intermediate 2H-phosphole 3b, which is then available for an additional cycloaddition reaction. Furthermore, 3b seems to react in a highly stereoselective manner in cycloaddition reactions, since only one of its dimers is formed at low temperatures. However, treating 2b/3b with 4 at −30 °C (addition of SiMe3Cl to a solution of 1 in THF at −30 °C, 30 min equilibration, addition of 4, 16 h at −30 °C) and subsequent filtration over silica gave 5 (characterised by 31P{1H} NMR spectroscopy). Therefore, direct trapping of a 2H-phosphole, generated from the corresponding protonated or silylated 1H-phosphole, with 4 did not yield the desired 1-phosphanorbornene.
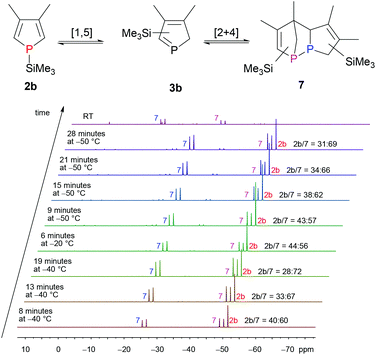 |
| Fig. 1 Temperature dependence of the equilibrium between 1H-phosphole 2b and the corresponding 2H-phosphole dimer 7 with SiMe3 as migrating group. | |
Generating 2H-phosphole 3ain situ from its corresponding dimer required a chiral dienophile that offers good stereoselectivity at the high temperatures needed for the cycloreversion of the dimer of 3a. These requirements are met by (5R)-(–)-menthyloxy-2(5H)-furanone (8). Owing to its rigid cyclic structure and fixed chiral centre, which is close to the C
C bond, it has shown high selectivities in cycloaddition reactions.10
As shown previously, this approach resulted in the desired products 9 and 10.6 The yields and selectivities were good to high and the absolute configuration of the obtained isomers was completely determined by NOE NMR spectroscopy and X-ray crystallography (Table 1). The sulfurisation of the phosphorus atom made the separation of the single isomers feasible. The molecular structure of endo-9d is shown in Fig. 2. However, the synthesis of C2-symmetric bis-phosphanes starting from bis-phospholes11 was not successful.6
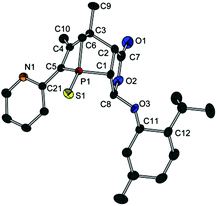 |
| Fig. 2 Molecular structure of endo-9d. Ellipsoid level at 50%. Hydrogen atoms are omitted for clarity. Selected bond lengths (in pm) and angles (in °): P(1)–S(1) 192.93(3), P(1)–C(1) 185.02(9), P(1)–C(5) 181.1(1), P(1)–C(6) 180.1(1), C(4)– C(5) 135.5(1), C(6)–P(1)–S(1) 125.85(3), C(1)–P(1)–S(1) 119.09(3), C(6)–P(1)–C(1) 91.64(5). | |
Table 1 Conditions, stereoselectivities,a and yields for compounds 9/10a,c,d.6
Furthermore, a detailed explanation of the observed selectivities was given which was supported by DFT calculations. The observed cycloaddition reaction is a Diels–Alder reaction with normal electron demand.6 A closer look at the energetic profile of the reaction (Fig. 3) gave further insight into the course of the reaction. Under both conditions (THF at 60 °C and xylenes at 140 °C), endo-11a is slightly more thermodynamically stable than exo-11a, and the activation enthalpy is smaller for the endo transition state, which is in agreement with the observed selectivities. Since the activation enthalpies are very similar under both conditions, a cycloreversion is more likely at higher temperatures.
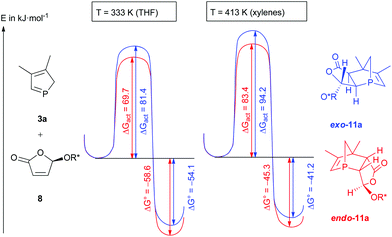 |
| Fig. 3 Energetic profile of the cycloaddition between 3a and 8. | |
Deprotection of P-chiral 1-phosphanorbornenes 9a,c,d
After an efficient method to create P-chiral 1-phosphanorbornenes in a stereoselective manner had been established, we focused on their functionalisation. In this context the application in transition metal catalysis is an important aspect. Consequently, desulfurisation of the diastereomerically pure compounds 9a,c,d to yield their trivalent derivatives 11a,c,d was performed with Raney nickel or electron-rich phosphanes like triethylphosphane, which – unlike hydridic reagents such as LiAlH4 or BH3(THF) – tolerate a number of functional groups and selectively reduce the P
S bond (Scheme 2). The 31P{1H} NMR spectra of the reaction mixture of triethylphosphane and diastereomerically pure 1-phosphanorbornenes 9a,c,d revealed a mixture of products containing endo and exo diastereomers of 11a,c,d. This suggests that a cycloreversion reaction takes place, in agreement with the computational results. In this regard, heating only diastereomerically pure 9a,c,d in xylenes at 130 °C for 16 h did not result in transformation into the other diastereomer. Thus, cycloreversion only takes place for the trivalent derivatives 11a,c,d and not for pentavalent 9a,c,d. In the case of endo-11d, only traces of the exo diastereomer were observed in the reaction mixture, and pure endo-11d was isolated in high yield. For endo-9a, endo-9c, exo-9c, and exo-9d, Raney nickel is the reagent of choice and gives the corresponding trivalent compounds 11a,c,d in moderate to good yields. However, it is important to perform the desulfurisation with Raney nickel at room temperature to avoid transformation into the other diastereomer. A transition metal complex, namely, [W(CO)5(endo-11a)], has been obtained previously.6
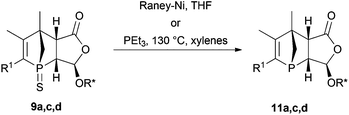 |
| Scheme 2 Desulfurisation of P-chiral 1-phosphanorbornenes; yields and conditions: endo-11a: 55%, Raney nickel; endo-11c: 75%, Raney nickel; exo-11c: 87%, Raney nickel; endo-11d: 93%, PEt3; exo-11d: 71%, Raney nickel. | |
Functionalisation of 1-phosphanorbornenes
Functionalisation of the 1-phosphanorbornenes should facilitate an efficient ligand design. As an improved synthesis compared to the reported one6 afforded endo-9a on a multigram scale, this derivative was studied first. In general, the different functional groups in endo-9a allow various modifications. However, when introduction of a second donor group is considered, functionalisation of the C
C bond employing hydroboration or epoxidation followed by ring opening would result in regioisomers with uncontrollable regioselectivity. Furthermore, introducing another donor group at the α position of the carbonyl group via the enolate would result in an anti position to the phosphorus atom and thus the resulting compound could not act as a chelating ligand. We, therefore, focused on functionalisation of the menthyloxy-furanone ring. Reductive cleavage of the chiral auxiliary gave diol 12a (Scheme 3). For further modifications, sulfur protection was necessary due to a higher tolerance of the P
S group towards various reagents (see further discussion). A selective chemical transformation of only one of the two OH groups in diol 12a seems challenging. However, treatment of 12a with a strong base resulted in a Michael addition of the OH group in the 4-position relative to the phosphorus atom. The overall yield of 13a over a two-step process is good. The intramolecular Michael addition is regio- and stereospecific, since only the OH group in 4-position to the phosphorus atom can attack the C
C bond; the attack proceeds anti to the bridge of the 1-phosphanorbornene. Consequently this transformation is restricted to the endo isomers. This simple and facile derivatisation of endo-9a prompted us to expand this synthetic strategy to endo-9c and endo-9d. The additional challenge in these two cases is the generation of a second stereogenic centre in α position to the phosphorus atom, which does not proceed in a stereospecific manner (Scheme 4). Reduction of endo-9c gives diol 12c in moderate yield due to low solubility of 12c in common organic solvents (see Exp. Section). The subsequent intramolecular Michael addition is an equilibrium reaction. Full conversion of 12c could not be observed. Furthermore, when the pure isomer (S)-13c (obtained by fractional crystallisation) was treated with KOtBu in THF, the formation of 12c and (R)-13c was observed by 31P{1H} NMR spectroscopy; after 20 h at room temperature an equilibrium was reached showing the ratio 12c
:
(R)-13c
:
(S)-13c = 2
:
33
:
66.
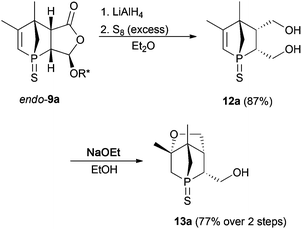 |
| Scheme 3 Reductive cleavage of the chiral auxiliary. | |
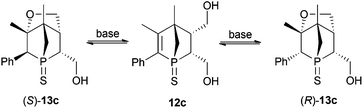 |
| Scheme 4 Intramolecular Michael addition of diol 12c. | |
M06-2X/MG3S calculations showed that the difference in energy between the two diastereomers is very small (3.5 kJ mol−1), and thus thermodynamic control of the reaction is impossible, in agreement with the observed selectivities. Varying the base or temperature gave neither full conversion of 12c nor improved stereoselectivity, so that kinetic control of the reaction is impossible as well. Therefore, functionalisation by reduction and subsequent Michael addition is only applicable to endo-9a.
Crystals of both diols (12a and 12c) and all three alcohols (13a, (R)-13c and (S)-13c) suitable for crystal structure analysis were obtained, and thus the absolute configurations of (R)-13c and (S)-13c could be deduced (Fig. 4 and 5). Comparing the molecular structures of 12a and 13a reveals the unique structural features of a 1-phosphanorbornene in contrast to a 1-phosphanorbornane (Fig. 4). The biggest difference between the two structures is observed for the σ bonds in the six-membered ring. In 12a, the P(1)–C(5) and C(3)–C(4) bonds are shorter than in 13a; in contrast, the P(1)–C(1) and C(2)–C(3) bonds are longer. This is caused by the hyperconjugation of the π* orbital of the C(4)–C(5) double bond with σ orbitals of the P(1)–C(1) and C(2)–C(3) bonds, resulting in a delocalisation of the C
C bond over the 1-phosphanorbornene system.12 These lengthenings and shortenings of the bonds are considered to be a preliminary stage of a retro-Diels–Alder reaction.12a,13 Due to the backbonding of all three P–C σ* orbitals with the nonbonding orbitals of the sulfur atom, the P
S bond in 12a is included in the delocalisation and also shortened.
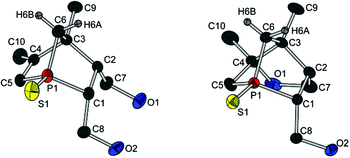 |
| Fig. 4 Molecular structures of 12a (left) and 13a (right); ellipsoid level at 50%. Hydrogen atoms except H(6A) and H(6B) are omitted. Selected bonds lengths (in pm): 12a: S(1)–P(1) 194.47(4), P(1)–C(1) 184.5(1), P(1)–C(5) 179.3(1), P(1)–C(6) 180.4(1), C(1)–C(2) 156.6(1), C(2)–C(3) 158.2(1), C(3)–C(4) 153.1(1), C(3)–C(6) 154.3(2), C(4)–C(5) 133.8(1), C(2)–C(7) 151.8(1); 13a: S(1)–P(1) 195.11(3), P(1)–C(1) 183.0(1), P(1)–C(5) 181.4(1), P(1)–C(6) 180.98(9), C(1)–C(2) 156.1(2), C(2)–C(3) 156.2(2), C(3)–C(4) 156.6(1), C(3)–C(6) 153.6(1), C(4)–C(5) 155.5 (2), C(2)–C(7) 151.4(2). | |
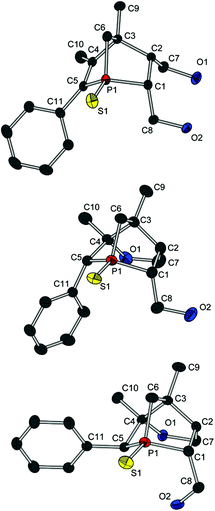 |
| Fig. 5 Molecular structures of 12c (top), (R)-13c (centre), (S)-13c (bottom); ellipsoid level at 50%. Hydrogen atoms are omitted for clarity. Selected bond lengths (in pm) and angles (in °): 12c: S(1)–P(1) 193.8(1), P(1)–C(1) 184.5(1), P(1)–C(5) 181.3(2), P(1)–C(6) 180.0(2), C(1)–C(2) 156.7(2), C(2)–C(3) 158.1(2), C(3)–C(4) 153.5(2), C(4)–C(5) 135.1(2), O(2)–C(8) 142.7(2), C(4)–C(3)–C(2) 109.4(1), C(5)–P(1)–C(1) 101.14(6); (R)-13c: S(1)–P(1) 195.08(8), P(1)–C(1) 181.4(2), P(1)–C(5) 182.6(2), P(1)–C(6) 181.0(2), C(1)–C(2) 155.4(2), C(2)–C(3) 155.7(2), C(3)–C(4) 157.0(2), C(4)–C(5) 157.8(2), O(2)–C(8) 142.9(2), C(4)–C(3)–C(2) 99.3(1), C(5)–P(1)–C(1) 105.95(7); (S)-13c: S(1)–P(1) 194.25(9), P(1)–C(1) 182.6(1), P(1)–C(5) 183.9(2), P(1)–C(6) 182.1(1), C(1)–C(2) 155.5(2), C(2)–C(3) 156.0(2), C(3)–C(4) 156.2(2), C(4)–C(5) 158.9(2), O(2)–C(8) 142.3(2); C(4)–C(3)–C(2) 99.68(9), C(5)–P(1)–C(1) 102.14(6). | |
A well-known effect in norbornene or norbornadiene systems is the strong NMR deshielding of the bridge atom. Even though it is known that the reason for this observation is the delocalisation of the double bond as well, the way in which the bridge is involved is not completely understood. Some theories suggest a direct hyperconjugation of the π orbitals with the exocyclic σ orbitals of the bridge.12,14a An interaction between the σ orbitals of the endocyclic bond of the bridge and the π* orbitals is also discussed, but seems unlikely due to the orientation of the corresponding orbitals.12b However, on looking at the torsion angles C(4)–C(3)–C(6)–H(6A) (167(1)° for 12a and 13a) und C(2)–C(3)–C(6)–H(6B) (178(1)° for 12a and 173(1)° for 13a), the fixed antiperiplanar orientation of these bonds is distinctive. Hence, an efficient hyperconjugation between these bonds and therefore indirectly with the π bond could cause a delocalisation of the double bond over the whole 1-phosphanorbornene system. The same conclusions can be drawn from the molecular structures of 12c and (R)-13c and (S)-13c (see Fig. 5).
Compound 13a represents a key molecule for divergent ligand synthesis, as the unaltered OH group in 3-position to the phosphorus atom facilitates the introduction of various functional and coordinating groups to afford chelating ligands. First, nucleophilic attack of the OH group at diarylchlorophosphanes was conducted, initially without protecting the exocyclic phosphorus atom. However, hydrolysis of the P–O bond was observed during purification of the resulting phosphinites by column chromatography (silica or alumina). An alternative purification by recrystallisation did not yield a pure product. When the second phosphorus atom was also protected with sulfur, compounds 14a,b (Scheme 5) could be easily purified by column chromatography, crystallised, and structurally characterised (Fig. 6). Unfortunately, a subsequent sulfur deprotection of 14a,b was not successful with the methods described in Scheme 2. 31P{1H} NMR spectroscopic studies showed that only the endocyclic phosphorus atom and not the exocyclic one is desulfurised.
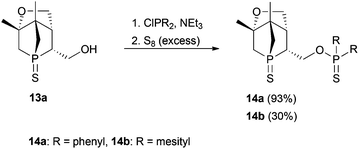 |
| Scheme 5 Synthesis of phosphinites 14. | |
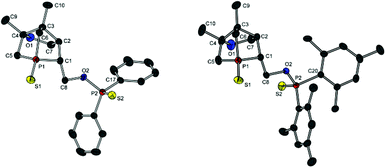 |
| Fig. 6 Molecular structures of 14a (left) and 14b (right); ellipsoid level at 50%. Hydrogen atoms are omitted for clarity. Selected bonds lengths (in pm): 14a: S(1)–P(1) 194.71(6), S(2)–P(2) 193.50(6), C(1)–C(8) 150.1(3), O(2)–C(8) 145.7(2); 14b: S(1)–P(1) 194.60(7), S(2)–P(2) 193.68(7), C(1)–C(8) 151.3(2), O(2)–C(8) 144.9(2). | |
Since preparation of the phosphane–phosphinite ligands (deprotected 14a,b) was not successful, another approach was pursued to obtain bis-phosphanes having only P–C bonds (Scheme 6). Bromination of 13a with Br2PPh3 gave bromide 15 in excellent yield. However, the following nucleophilic substitution turned out to be challenging. Treating 15 with LiPPh2 resulted in elimination of HBr giving 16; apparently, the exocyclic proton in α position is rather acidic. Nevertheless, applying the less basic lithium phospholide 1 followed by sulfur protection afforded the desired product 17 in excellent yield. The thiophosphole group of 17 can now be employed in other asymmetric Diels–Alder reactions.15 The basicity of lithium phosphanides like LiPPh2 can be reduced by coordination to borane. Accordingly, LiP(BH3)Ph2 gave access to 18 in good yield. Compound 18 was fully characterised, also by X-ray crystallography (Fig. 7). Since no reducible groups, such as the P–O bond in 14a,b are present, sulfur deprotection was performed under very mild conditions with LiAlH4. The corresponding bis-phosphane was isolated as bis-borane adduct 19 in good yield. 31P{1H} NMR studies showed that its trivalent species 20 can be generated quantitatively in situ with morpholine; however, 20 was not isolated.
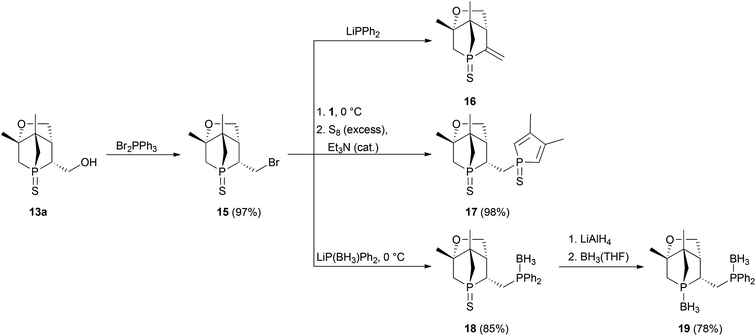 |
| Scheme 6 Bromination of 13a and subsequent nucleophilic substitution at 15. | |
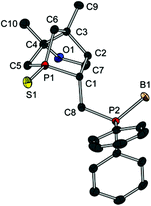 |
| Fig. 7 Molecular structure of 18; ellipsoid level at 50%. Hydrogen atoms are omitted for clarity. Bond lengths (in pm) and angles (in °): S(1)–P(1) 194.4(1), P(1)–C(1) 181.9(3), P(1)–C(5) 180.8(3), P(1)–C(6) 180.7(3), C(1)–C(2) 157.2(4), C(2)–C(3) 155.7(4), C(3)–C(4) 156.0(4), C(4)–C(5) 155.3(4), P(2)–B(1) 192.1(3); C(6)–P(1)–C(5) 93.1(2), C(6)–P(1)–S(1) 125.5(1), C(5)–P(1)–S(1) 119.9(1), C(8)–P(2)–B(1) 113.3(2), C(11)–P(2)–B(1) 112.5(2), C(11)–P(2)–C(8) 105.9(1). | |
Conclusions
A diastereoselective synthetic route towards P-chiral 1-phospha-norbornenes has been established by using the principle of stereotopic face differentiation. The observed reaction pathway was supported by theoretical calculations showing that the cycloaddition reaction between 2H-phosphole 3a and dienophile 8 is of normal electron demand. The phosphorus atom in the obtained Diels–Alder products 9a,c,d was easily desulfurised to give the corresponding trivalent species 11a,c,d. Further functionalisation yielded alcohol 13a as a key compound for divergent ligand synthesis. A subsequent electrophilic substitution at the OH group gave phosphinites which are sensitive towards hydrolysis. This problem was overcome by sulfur protection (14a,b). Finally, bromination of 13a afforded bromide 15, which underwent elimination of HBr in the presence of a strong base with formation of 16. By using less basic nucleophiles, this elimination reaction could be suppressed and the desired nucleophilic substitution products (17 and 18) were obtained. The phosphanes presented in this work are expected to be very useful as ligands in asymmetric transition metal catalysis.
Experimental
General methods
All reactions were carried out under dry high purity nitrogen using standard Schlenk techniques. Experiments including elemental lithium were carried out under dry high purity argon. THF was degassed and distilled from potassium. The NMR spectra were recorded with a Bruker Avance DRX 400 spectrometer (1H NMR 400.13 MHz, 13C NMR 100.63 MHz, 31P NMR 161.98 MHz) or a Bruker Fourier 300 spectrometer (1H NMR 300.23 MHz, 13C NMR 75.50 MHz). 13C{1H} NMR spectra were recorded as APT spectra. Assignment of the chemical shifts and configurations was done using COSY, HMQC, HSQC, HMBC and selective NOE techniques. If not mentioned otherwise, CDCl3 was used as deuterated solvent. TMS was used as the internal standard in the 1H NMR spectra and all other nuclei spectra were referenced to TMS using the Ξ-scale.16 A detailed assignment of all NMR data for all compounds is given in the ESI. Mass spectra (ESI) were measured using a Bruker Daltonics Esquire 3000 Plus spectrometer. High-resolution mass spectra (HRMS; ESI) were measured using a Bruker Daltonics APEX II FT-ICR spectrometer. IR spectra were obtained with an FTIR spectrometer (Genesis ATI Mattson/Unicam or Perkin-Elmer Spectrum 2000 FT-IR) in the range of 400–4000 cm−1 in KBr. The melting points were determined in glass capillaries sealed under nitrogen using a Gallenkamp apparatus and are uncorrected. Column chromatography was performed using silica 60 (0.015–0.040 mm) purchased from Merck. UV light (389 nm), phosphomolybdic acid (12 g phosphomolybdic acid in 100 ml ethanol), para-anisaldehyde (140 ml ethanol, 5 ml sulfuric acid (conc.), 1.5 ml glacial acetic acid, 4 ml para-anisaldehyde) and iodine (saturated atmosphere) were used as staining reagents.
The compounds 3,4-dimethyl-1-phenylphosphole (2c),17 3,4-dimethyl-1-(2-pyridyl)phosphole (2d),184,199c,d,6 benzyldiphenylphosphane,20 and dibromotriphenylphosphane21 were synthesised according to the literature. All other compounds are commercially available.
Synthesis of 5.
Isopropanol was added at −30 °C to a 0.11 M stock solution of 1-lithium-3,4-dimethyl phosphole (see synthesis of 9a) in THF (5.0 ml, 0.55 mmol). The solution was stirred at this temperature for one hour; then crotonic acid amide 4 (0.13 g, 0.54 mmol) was added. After 72 hours at −30 °C, the solution was warmed to room temperature, sulfur (0.018 g, 0.56 mmol) and three drops of triethylamine were added and the solution was stirred for 16 hours at room temperature. Chromatographic workup (hexanes/diethyl ether = 1
:
3 to 1
:
2, v/v) of the crude product gave 5 as a white solid. Yield: 0.18 g, 87%. Rf (hexanes/diethyl ether = 1
:
3, v/v) = 0.22 – UV light, iodine. m.p.: 58–60 °C. [α]25D = +37.9° (c = 2.35 in toluene). 1H NMR (300 MHz): δ = 1.27 (3H, dd, 3JH,P = 18.7 Hz, 3JH,H = 7.1 Hz), 2.08 (6H, s), 2.60–2.84 (2H, m), 2.94–3.13 (1H, m), 3.24–3.54 (2H, m), 4.12–4.27 (2H, m), 4.62–4.75 (1H, m), 6.03 (2H, d, 2JH,P = 30.4 Hz), 7.18–7.26 (2H, m), 7.26–7.40 (3H, m) ppm. 13C{1H} NMR (76 MHz): δ = 15.2 (s), 17.4 (d, 3JC,P = 3.3 Hz), 17.6 (d, 3JC,P = 3.3 Hz) 31.1 (d, 1JC,P = 51.8 Hz), 37.1 (s), 37.9 (s), 55.3 (s), 66.4 (s), 122.1–123.5 (m), 123.1–123.6 (m), 127.4 (s), 129.0 (s), 129.4 (s), 135.1 (s), 153.3 (s), 153.8–154.5 (m), 171.0 (d, 3JC,P = 13.4 Hz) ppm. 31P{1H} NMR (162 MHz): δ = 61.5 ppm. IR (KBr):
= 3453 (s), 2963 (m, νC–H), 2345 (w), 1179 (s), 1699 (s), 1631 (m), 1543 (w), 1456 (m), 1387 (m), 1262 (m), 1217 (m), 1102 (m), 1121 (w), 802 (m), 763 (w), 685 (w), 658 (w), 633 (w), 597 (w), 502 (w), 457 (w), 442 (w), 417 (w) cm−1. MS (ESI, DCM/MeOH): m/z: calculated for C20H24NO3PS [M + Na]+: 412.1; found: 412.2. C20H24NO3PS (389.45): calculated: C 61.68%, H 6.21%; found: C 61.63%, H 6.19%.
In situ generation of 2b and 7.
A 0.11 M stock solution of 1-lithium-3,4-dimethyl phosphole (see synthesis of 9a) in THF (0.6 ml, 0.07 mmol) in an NMR tube was frozen in liquid nitrogen. Afterwards SiMe3Cl (2–3 fold excess) was added and the sample was equilibrated in the NMR machine at the given temperature. 31P{1H} NMR (162 MHz, no deuterated solvent): see Fig. 1.
Synthesis of endo-9a: endo-9a was synthesised by a modified method reported before6.
A mixture of 3,4-dimethyl-1-phenylphosphole (2b) (7.05 g, 37.5 mmol) and pieces of lithium (excess) in 200 ml THF was stirred at room temperature for 3–4 hours (conversion was monitored by TLC, with phosphomolybdic acid). The deep brown solution was filtered, cooled to −80 °C and oxygen-free water (4.05 ml, 225 mmol) was added dropwise. After complete addition, the solution was kept at −80 °C for 5 minutes and then warmed to room temperature over 30 minutes. Afterwards, the solution was dried over MgSO4, filtered and added to a flask containing (5R)-(L-menthyloxy)-2(5H)-furanone (8) (7.44 g, 31.2 mmol). The pale yellow solution was heated at 63 °C for 24 hours. Sulfur (1.25 g, 39.2 mmol) and two drops of triethylamine were added and the solution was kept at 40 °C for 18 hours. After cooling to rt, the solvent was removed in vacuum and the crude product was purified by recrystallisation (isopropanol/hexanes = 3
:
5, v/v) to give the main diastereomer endo-9a as a white solid. Compound 10 could not be isolated. Yield: 9.66 g, 81%. Rf (hexanes/diethyl ether = 1
:
1, v/v) = 0.33 – para-anisaldehyde. m.p.: 116–118 °C. [α]25D = −65.4° (c = 1.62 in toluene). 1H NMR (400 MHz): δ = 0.75 (3H, d, 3JH,H = 6.9 Hz), 0.81–0.91 (1H, m), 0.84 (3H, d, 3JH,H = 7.1 Hz), 0.91–1.04 (m, 2H), 0.93 (3H, d, 3JH,H = 6.5 Hz), 1.19–1.29 (1H, m), 1.30–1.40 (1H, m), 1.63 (3H, s), 1.61–1.68 (2H, m), 1.94 (3H, s), 1.91–1.97 (1H, m), 2.02–2.08 (2H, m), 2.11–2.18 (1H, m), 3.13–3.20 (1H, m), 3.25–3.31 (1H, m), 3.50 (1H, ddd, 3JH,H = 10.7 Hz, 3JH,H = 10.7 Hz, 3JH,H = 2.4 Hz), 5.49 (1H, d, 3JH,P = 13.0 Hz), 5.90 (1H, d, 2JH,P = 26.3 Hz) ppm. 13C{1H} NMR (101 MHz): δ = 15.7 (s), 18.6 (d, 3JC,P = 14.2 Hz), 19.3 (d, 3JC,P = 16.2 Hz), 20.9 (s), 22.2 (s), 23.1 (s), 25.4 (s), 31.5 (s), 34.2 (s), 40.0 (s), 47.5 (s), 48.6 (d, 1JC,P = 46.6 Hz), 50.9 (d, 2JC,P = 21.7 Hz), 53.5 (s), 56.5 (d, 1JC,P = 57.6 Hz), 78.7 (s), 99.5 (d, 2JC,P = 5.9 Hz), 121.1 (d, 1JC,P = 69.3 Hz), 165.5 (d, 2JC,P = 7.2 Hz, 173.6 (d, 3JC,P = 3.0 Hz) ppm. 31P{1H} NMR (162 MHz): δ = 48.7 ppm. IR (KBr):
= 3455 (m), 2953 (s, νC–H), 2925 (s, νC–H), 2870 (s, νC–H), 1766 (s, νO–C
O), 1633 (w), 1580 (m), 1456 (m), 1436 (m), 1414 (m), 1385 (m), 1371 (m), 1346 (m), 1303 (w), 1244 (m), 1231 (m), 1203 (w), 1178 (s), 1166 (s), 1119 (s), 1074 (m), 1038 (m), 1010 (m), 989 (s), 975 (m), 942 (s), 902 (m), 890 (m), 858 (m), 848 (w), 833 (m), 819 (m), 776 (m), 740 (m), 723 (s), 708 (m), 678 (s), 631 (w), 420 (s) cm−1. HRMS (ESI, MeOH): m/z: calculated for C20H31O3PS [M + Na]+: 405.16
237; found: 405.16
229. C20H31O3PS (382.50): calculated: C 62.8%, H 8.17%; found: C 63.4%, H 8.23%.
General procedure for the activation of Raney nickel
In a Schlenk flask under nitrogen atmosphere solid NaOH was added to a vigorously stirred suspension of an aluminium-nickel alloy (Raney type) in 20 ml of water portionwise in such a manner that the gas evolution was kept under control (caution: initiation time required, solution warms up!). In this process, the greyish metal alloy turned into a dark black solid. After the gas evolution had ceased, the reaction mixture was stirred at 80 °C for one hour. The clear solution was decanted and the black metal was washed with 15 ml portions of water under vigorous stirring until a pH of 8–9 was accomplished (usually 6–8 washing steps). Afterward, the Raney nickel was washed three times with 15 ml of ethanol and two times with 15 ml of THF. After desulfurisation was completed, the nickel waste was destroyed by addition of diluted HCl and subsequently concentrated HCl.
Synthesis of endo-11a.
A suspension of freshly prepared Raney nickel (2.20 g, excess) and endo-9a (0.420 g, 1.10 mmol) in 15 ml of THF was stirred for 40 hours at room temperature. The solution was filtered and the black solid was washed three times with 15 ml of THF. Chromatographic workup (toluene) gave endo-11a as white solid. Yield: 0.213 g, 55%. Rf (toluene) = 0.34 – para-anisaldehyde. m.p.: 130–132 °C. [α]25D = +231° (c = 1.52 in toluene). 1H NMR (300 MHz): δ = 0.75 (3H, d, 3JH,H = 6.9 Hz), 0.87 (3H, d, 3JH,H = 7.1 Hz), 0.65–1.13 (3H, m), 0.93 (3H, d, 3JH,H = 6.5 Hz), 1.15–1.27 (1H, m), 1.28–1.46 (2H, m), 1.52 (1H, dd, J = 11.5 Hz, J = 8.9 Hz), 1.63 (3H, s), 1.57–1.70 (2H, m), 1.88 (3H, s), 1.96–2.16 (2H, m), 2.97–3.07 (2H, m), 3.42 (1H, ddd, 3JH,H = 10.7 Hz, 3JH,H = 10.7 Hz, 3JH,H = 4.2 Hz), 5.04 (1H, dd, 3JH,P = 8.1 Hz, 3JH,H = 1.4 Hz), 5.87 (1H, d, 2JH,P = 45.0 Hz) ppm. 13C{1H} NMR (76 MHz): δ = 15.7 (s), 18.3 (s), 19.2 (s), 20.9 (s), 22.2 (s), 23.2 (s), 25.4 (s), 31.4 (s), 34.3 (s), 40.0 (s), 46.3 (d, 1JC,P = 19.6 Hz), 47.7 (s), 51.8 (d, 2JC,P = 1.8 Hz), 53.2 (d, 1JC,P = 4.9 Hz), 62.9 (d, 2JC,P = 6.1 Hz), 77.2 (s), 101.9 (d, 2JC,P = 11.4 Hz), 124.0 (d, 1JC,P = 24.2 Hz), 163.8 (d, 2JC,P = 4.3 Hz), 175.5 (s) ppm. 31P{1H} NMR (162 MHz): δ = −30.0 ppm. IR (KBr):
= 3458 (s), 2950 (m, νC–H), 2867 (m, νC–H), 1760 (s, νO–C
O), 1636 (w), 1584 (w), 1564 (w), 1460 (m), 1432 (w), 1358 (w), 1262 (w), 1205 (w), 1180 (m), 1114 (s), 988 (w), 944 (s), 801 (w), 777 (w), 751 (w), 681 (w), 646 (w), 548 (w), 490 (w), 469 (w), 414 (w), 428 (w), 417 (w) cm−1. MS (ESI, DCM/MeOH): m/z: calculated for C20H31O3P [M + Na]+: 373.2; found: 373.2. C20H31O3P (350.43): calculated: C 68.6%, H 8.9%; found: C 68.5%, H 8.89%.
Synthesis of endo-11c.
A suspension of freshly prepared Raney nickel (2.02 g, excess) and endo-9c (0.310 g, 0.676 mmol) in 15 ml of THF was stirred for 30 hours at room temperature. The solution was filtered and the black solid was washed three times with 15 ml of THF. Chromatographic workup (toluene) gave endo-11c as a white solid. Yield: 0.216 g, 75%. Rf (toluene) = 0.40 – UV light, para-anisaldehyde. m.p.: 128–130 °C. [α]25D = +260° (c = 1.27 in toluene). 1H NMR (300 MHz): δ = 0.74 (3H, d, 3JH,H = 6.9 Hz), 0.64–1.03 (9H, m), 1.06–1.20 (1H, m), 1.20–1.32 (1H, m), 1.35–1.49 (1H, m), 1.50–1.74 (3H, m), 1.70 (3H, s), 1.76–1.84 (1H, m), 1.94 (3H, s), 1.99–2.13 (1H, m), 3.00–3.24 (2H, m), 3.36 (1H, ddd, 3JH,H = 10.4 Hz, 3JH,H = 10.4 Hz, 3JH,H = 4.1 Hz), 5.06 (1H, d, 3JH,P = 7.1 Hz), 7.20–7.41 (5H, m) ppm. 13C{1H} NMR (76 MHz): δ = 15.0 (s), 15.7 (s), 20.2 (s), 20.8 (s), 22.1 (s), 23.1 (s), 25.4 (s), 31.3 (s), 34.2 (s), 39.8 (s), 46.8 (d, 1JC,P = 21.3 Hz), 47.6 (s), 51.0–51.2 (m), 64.5 (d, 2JC,P = 6.3 Hz), 77.1 (s), 101.0 (d, 2JC,P = 10.6 Hz), 127.0 (s), 128.4 (s), 129.0 (d, 3JC,P = 6.8 Hz), 136.8–139.3 (m), 153.9 (s), 175.6 (s) ppm. 31P{1H} NMR (162 MHz): δ = −14.5 ppm. IR (KBr):
= 3445 (s), 2963 (s, νC–H), 2922 (s, νC–H), 2868 (m, νC–H), 1754 (m, νO–C
O), 1631 (w), 1558 (w), 1506 (w), 1489 (w), 1456 (m), 1442 (w), 1386 (m), 1379 (m), 1351 (s), 1331 (m), 1298 (w), 1262 (w), 1246 (w), 1200 (m), 1168 (s), 1129 (m), 1106 (s), 1074 (m), 1038 (w), 1007 (w), 987 (m), 973 (w), 938 (s), 879 (w), 847 (w), 813 (w), 790 (w), 754 (w), 703 (m), 671 (w), 633 (w), 611 (w), 541 (w), 514 (w), 492 (w), 475 (w), 448 (w), 439 (w), 417 (w), 408 (w) cm−1. MS (ESI, MeOH): m/z: calculated for C26H35O3P [M + Na]+: 449.2; found: 449.3. C26H35O3P (426.53): calculated: C 73.2%, H 8.27%; found: C 73.3%, H 8.14%.
Synthesis of exo-11c.
A suspension of freshly prepared Raney nickel (2.01 g, excess) and exo-9c (0.329 g, 0.717 mmol) in 15 ml of THF was stirred for 30 hours at room temperature. The solution was filtered and the black solid was washed three times with 15 ml of THF. Chromatographic workup (toluene) gave exo-11c as a white solid. Yield: 0.267 g, 87%. Rf (toluene) = 0.42 – UV light, para-anisaldehyde. m.p.: 55–57 °C. [α]25D = −148° (c = 3.60 in toluene). 1H NMR (300 MHz): δ = 0.80 (3H, d, 3JH,H = 6.9 Hz), 0.87 (3H, d, 3JH,H = 7.0 Hz), 0.71–1.08 (3H, m), 0.94 (3H, d, 3JH,H = 6.5 Hz), 1.14–1.42 (3H, m), 1.51 (1H, dd, J = 12.9 Hz, J = 8.0 Hz), 1.60–1.73 (2H, m), 1.64 (3H, s), 1.87 (3H, s), 2.02–2.20 (2H, m), 2.57–2.66 (2H, m), 3.55 (1H, ddd, 3JH,H = 10.7 Hz, 3JH,H = 10.7 Hz, 3JH,H = 4.2 Hz), 5.51 (1H, d, 3JH,P = 8.8 Hz), 7.30–7.15 (3H, m), 7.30–7.38 (2H, m) ppm. 13C{1H} NMR (76 MHz): δ = 13.0 (s), 15.7 (s), 17.8 (s), 20.9 (s), 22.2 (s), 23.2 (s), 25.4 (s), 31.4 (s), 34.3 (s), 39.9 (s), 42.3 (d, 1JC,P = 7.1 Hz), 47.8 (s), 48.7 (d, 2JC,P = 2.4 Hz), 49.5 (d, 1JC,P = 19.0 Hz), 63.9 (d, 2JC,P = 6.4 Hz), 76.9 (s), 101.3 (d, 2JC,P = 29.2 Hz), 126.9 (s), 128.1 (d, 3JC,P = 7.3 Hz), 128.3 (s), 137.6 (d, 2JC,P = 20.5 Hz), 142.2 (d, 1JC,P = 16.5 Hz), 154.2 (s), 174.8 (s) ppm. 31P{1H} NMR (162 MHz): δ = −10.1 ppm. IR (KBr):
= 3424 (s), 2956 (s, νC–H), 2925 (s, νC–H), 2866 (m, νC–H), 2346 (w), 1769 (s, νO–C
O), 1630 (w), 1558 (w), 1506 (w), 1491 (w), 1456 (m), 1385 (w), 1370 (w), 1346 (m), 1243 (m), 1157 (s), 1104 (s), 1049 (m), 1010 (w), 976 (m), 944 (s), 921 (m), 868 (w), 848 (w), 797 (w), 756 (m), 698 (m), 674 (w), 640 (w), 591 (w), 549 (w), 518 (w), 493 (w), 467 (w), 453 (w), 441 (w), 422 (w), 416 (w) cm−1. MS (ESI, MeOH): m/z: calculated for C26H35O3P [M + Na]+: 449.2; found: 449.3. C26H35O3P (426.53): calculated: C 73.2%, H 8.27%, found: C 73.4%, H 8.04%.
Synthesis of endo-11d.
A solution of endo-9d (0.35 g, 0.75 mmol) and PEt3 (0.25 ml, 1.7 mmol) in 5 ml of xylenes was heated at 130 °C for 5 hours. Chromatographic workup (hexanes/diethyl ether = 5
:
1 to 3
:
1, v/v) gave endo-11d as a white solid. Yield: 0.30 g, 93%. Rf (hexanes/diethyl ether = 2
:
1, v/v) = 0.35 – UV light, para-anisaldehyde. m.p.: 129–131 °C. [α]25D = −27.2° (c = 1.62 in toluene). 1H NMR (300 MHz): δ = 0.67 (3H, d, 3JH,H = 6.9 Hz), 0.80 (3H, d, 3JH,H = 7.0 Hz), 0.59–0.99 (3H, m), 0.88 (3H, d, 3JH,H = 6.5 Hz), 1.10–1.30 (2H, m), 1.32–1.44 (1H, m), 1.50–1.62 (3H, m), 1.65 (3H, s), 1.95 (3H, s), 2.00–2.09 (1H, m), 2.09–2.18 (1H, m), 2.95–3.18 (2H, m), 3.31 (1H, ddd, 3JH,H = 10.6 Hz, 3JH,H = 10.6 Hz, 3JH,H = 4.1 Hz), 5.68 (1H, d, 3JH,P = 8.3 Hz), 7.06–7.12 (1H, m), 7.16–7.27 (1H, m), 7.44–7.75 (1H, m), 8.43–8.60 (1H, m) ppm. 13C{1H} NMR (76 MHz): δ = 15.0 (s), 15.8 (s), 20.1 (s), 20.9 (s), 22.4 (s), 23.2 (s), 25.5 (s), 31.5 (s), 34.4 (s), 40.1 (s), 46.3 (d, 1JC,P = 21.8 Hz), 47.6 (s), 50.9 (d, 1JC,P = 5.6 Hz), 52.2 (s), 65.1 (d, 2JC,P = 6.7 Hz), 77.5 (s), 102.7 (d, 2JC,P = 10.0 Hz), 121.3 (s), 123.1 (d, 3JC,P = 4.1 Hz), 135.8 (s), 140.6 (d, 1JC,P = 18.1 Hz), 149.4 (s), 155.8 (s), 157.2 (d, 2JC,P = 17.3 Hz), 175.5 (s) ppm. 31P{1H} NMR (162 MHz): δ = −13.6 ppm. IR (KBr):
= 3424 (s), 2949 (s, νC–H), 2864 (m, νC–H), 1759 s (νO–C
O), 1603 (m), 1584 (s), 1563 (w), 1460 (s), 1433 (m), 1411 (w), 1386 (m), 1357 (m), 1281 (w), 1262 (w), 1245 (w), 1205 (m), 1180 (s), 1156 (m), 1128 (s), 1114 (s), 1040 (w), 1011 (m), 987 (m), 970 (w), 944 (s), 916 (m), 879 (w), 843 (w), 798 (w), 777 (m), 751 (w), 719 (w), 704 (w), 681 (m), 645 (w), 612 (w), 541 (w), 492 (w), 469 (w), 448 (w), 440 (w), 423 (w) cm−1. MS (ESI, MeOH): m/z: calculated for C25H34NO3P [M + Na]+: 450.2; found: 450.3. C25H34NO3P (427.52): calculated: C 70.2%, H 8.02%; found: C 70.1%, H 8.08%.
Synthesis of exo-11d.
A suspension of freshly prepared Raney nickel (2.08 g, excess) and exo-9d (0.320 g, 0.696 mmol) in 15 ml of THF was stirred for 43 hours at room temperature. The solution was filtered and the black solid was washed three times with 10 ml of THF. Chromatographic workup (hexanes/diethyl ether = 2
:
1, v/v) gave exo-11d as a white solid. Yield: 0.211 g, 71%. Rf (hexanes/diethyl ether = 2
:
1, v/v) = 0.25 – UV light, para-anisaldehyde. m.p.: 58–60 °C. [α]25D = −170° (c = 2.27 in toluene). 1H NMR (300 MHz): δ = 0.70 (3H, d, 3JH,H = 6.9 Hz), 0.77 (3H, d, 3JH,H = 7.0 Hz), 0.57–0.99 (3H, m), 0.86 (3H, d, 3JH,H = 6.4 Hz), 1.01–1.32 (3H, m), 1.33–1.47 (1H, m), 1.48–1.64 (2H, m), 1.57 (3H, s), 1.93 (3H, s), 1.96–2.03 (1H, m), 2.03–2.11 (1H, m), 2.51–2.59 (1H, m), 2.61–2.73 (1H, m), 3.46 (1H, ddd, 3JH,H = 10.6 Hz, 3JH,H = 10.6 Hz, 3JH,H = 4.1 Hz), 5.48 (1H, d, 3JH,P = 9.0 Hz), 7.04 (1H, dd, 3JH,H = 7.7 Hz, 3JH,H = 4.8 Hz), 7.19 (1H, d, 3JH,H = 7.7 Hz), 7.57 (1H, dd, 3JH,H = 7.7 Hz, 3JH,H = 7.7 Hz), 8.51 (1H, d, 3JH,H = 4.8 Hz) ppm. 13C{1H} NMR (76 MHz): δ = 13.4 (s), 15.7 (s), 17.7 (s), 20.8 (s), 22.2 (s), 23.2 (s), 25.4 (s), 31.3 (s), 34.3 (s), 39.7 (s), 41.7 (d, 1JC,P = 7.2 Hz), 47.7 (s), 48.8 (s), 49.3 (d, 1JC,P = 19.2 Hz), 64.3 (d, 2JC,P = 6.6 Hz), 76.4 (s), 101.1 (d, 2JC,P = 29.9 Hz), 121.3 (s), 122.8 (d, 3JC,P = 6.7 Hz), 135.9 (s), 142.8 (d, 1JC,P = 14.8 Hz), 149.4 (s), 156.5 (d, 2JC,P = 19.8 Hz), 157.8 (s), 174.7 (s) ppm. 31P{1H} NMR (162 MHz): δ = −12.1 ppm. IR (KBr):
= 3440 (s), 2961 (s, νC–H), 2925 (s, νC–H) 2868 (m, νC–H), 1767 (s, νO–C
O), 1717 (w), 1608 (m), 1584 (m), 1564 (w), 1527 (w), 1506 (w), 1464 (m), 1432 (w), 1386 (w), 1348 (w), 1261 (m), 1160 (m), 1097 (s), 1050 (m), 978 (w), 943 (m), 921 (w), 868 (w), 802 (w), 747 (w), 681 (w), 647 (w), 542 (w), 521 (w), 469 (w), 440 (w), 429 (w), 409 (w) cm−1. MS (ESI, MeOH): m/z: calculated for C25H34NO3P [M + Na]+: 450.2; found: 450.3. C25H34NO3P (427.52): calculated: C 70.2%, H 8.02%; found: C 70.1%, H 8.13%.
Synthesis of 12a.
endo-9a (0.31 g, 0.81 mmol) was added to a suspension of LiAlH4 (0.080 g, 2.1 mmol) in 10 ml of diethyl ether at 0 °C. The cooling bath was removed and the solution was left to stir for 90 minutes. The reaction was quenched by the addition of 0.45 ml water, stirred for 30 minutes and MgSO4 was added. The mixture was transferred onto a D2 frit; and the solid was continuously extracted with a DCM/diethyl ether mixture (2
:
1, v/v) for 3 hours. Sulfur (0.03 g, 0.94 mmol) was added to the extract and the solution was stirred for 16 hours. After chromatographic workup (DCM/MeOH = 50
:
1 to 20
:
1, v/v) 12a was obtained as a white solid. Crystals suitable for X-ray diffraction measurements were obtained from chloroform. Yield: 0.16 g, 87%. Rf (DCM/methanol = 10
:
1, v/v) = 0.40 – iodine. m.p.: 145–147 °C. [α]25D = −59.4° (c = 2.31 in chloroform). 1H NMR (400 MHz): δ = 1.45 (3H, s), 1.88 (3H, s), 1.89–2.01 (2H, m), 2.47–2.70 (1H, m), 2.72–2.90 (1H, m), 3.36–3.48 (1H, m), 3.59–3.71 (1H, m), 3.71–3.81 (1H, m), 4.15–4.26 (1H, m), 4.81 (2H, s (br)), 5.76 (1H, d, 2JH,P = 26.3 Hz) ppm. 13C{1H} NMR (101 MHz): δ = 19.1 (d, 3JC,P = 15.0 Hz), 19.7 (d, 3JC,P = 17.0 Hz), 44.7 (d, 1JC,P = 42.4 Hz), 49.6 (d, 2JC,P = 24.2 Hz), 52.0 (s), 54.7 (d, 1JC,P = 56.3 Hz), 59.0 (d, 2JC,P = 6.2 Hz), 59.5 (d, 3JC,P = 3.4 Hz), 121.4 (d, 1JC,P = 68.8 Hz), 163.9 (d, 2JC,P = 7.4 Hz) ppm. 31P{1H} NMR (162 MHz): δ = 48.2 ppm. IR (KBr):
= 3470 (s), 2965 (m, νC–H), 2925 (m, νC–H), 2325. (w), 1625 (m), 1585 (m), 1475 (m), 1456 (m), 1433 (m), 1384 (m), 1314 (w), 1262 (w), 1159 (w), 1126 (s), 1043 (s), 1033 (s), 983 (w), 879 (w), 825 (w), 791 (m), 768 (m), 751 (w), 703 (s), 682 (s), 626 (w), 529 (w), 464 (w), 444 (w), 431 (w), 421 (w), 414 (w) cm−1. MS (ESI, DCM/MeOH): m/z: calculated for C10H17O2PS [M + Na]+: 255.1; found: 255.0. C10H17O2PS (232.28): calculated: C 51.7%, H 7.38%; found: C 51.4%, H 7.38%.
Synthesis of 13a.
A solution of endo-9a (12.91 g, 33.8 mmol) in 150 ml THF was added at 0 °C over 5 minutes to a mixture of LiAlH4 (2.56 g, 67.5 mmol) in 50 ml THF. The cooling bath was removed and the solution was left to stir for 20 hours. The reaction was quenched by slowly adding a solution of KOH (3.1 g in 10 ml degassed water) at 0 °C. The slurry was heated to 60 °C for one hour and then cooled to room temperature. The mixture was filtered and the residue was washed with 200 ml of THF. The combined filtrates were treated with sulfur (1.20 g, 37.4 mmol) and 0.50 ml of trimethylamine, and the solution was stirred at 55 °C for 16 hours. The solvent was removed under vacuum, the residue transferred to a pad of silica and washed with a mixture of hexanes/diethyl ether (1
:
1, v/v). Crude 12a was eluted by washing the silica pad with a mixture of DCM/methanol (1
:
1, v/v). The solvent was removed and the residue was dissolved in a solution of NaOEt in 170 ml of ethanol (prepared by dissolving sodium (1.60 g, 69.6 mmol) in 170 ml of ethanol). The solution was left to stir at room temperature for 4 days. The reaction was quenched by addition of glacial acid (4.00 ml, 63.4 mmol) and the solvent was removed in vacuum. The residue was taken up in a mixture of 60 ml water and 50 ml brine, and the organic phase was extracted once with 150 ml and three times with 75 ml of chloroform. After drying the combined organic phases over MgSO4, the solvent was removed and the residue was recrystallised from a mixture of isopropanol/hexanes (9
:
5, v/v) to give 13a as a white solid. Crystals suitable for X-ray diffraction measurements were obtained from DCM/hexanes at 4 °C. Yield: 6.11 g, 78%. Rf (DCM/methanol = 10
:
1, v/v) = 0.40 – iodine. m.p.: 124–126 °C. [α]25D = +17.2° (c = 2.04 in chloroform). 1H NMR (300 MHz): δ = 1.15 (3H, s), 1.21 (3H, s), 1.79–1.98 (2H, m), 1.99–2.13 (1H, m), 2.18–2.34 (1H, m), 2.39–2.67 (2H, m), 3.05 (1H, s (br), 3.71–4.24 (4H, m) ppm. 13C{1H} NMR (76 MHz): δ = 18.2 (d, 3JC,P = 16.0 Hz), 23.9 (d, 3JC,P = 7.3 Hz), 39.9 (d, 1JC,P = 51.5 Hz), 41.4 (d, 1JC,P = 44.8 Hz), 44.0 (d, 1JC,P = 47.6 Hz), 47.2 (d, 2JC,P = 1.5 Hz), 51.6 (d, 2JC,P = 19.5 Hz), 57.9 (d, JC,P = 1.9 Hz), 66.1 (s), 86.2 (s) ppm. 31P{1H} NMR (162 MHz): δ = 44.9 ppm. IR (KBr):
= 3484 (s), 2965 (m, νC–H), 2897 (m, νC–H), 2226. (w), 1631 (m), 1501 (w), 1459 (m), 1421 (m), 1385 (m), 1327 (m), 1298 (w), 1263 (m), 1204 (w), 1140 (m), 1082 (s), 1051 (m), 1043 (m), 1030 (m), 1006 (s), 959 (m), 935 (w), 889 (m), 862 (m), 788 (m), 776 (m), 751 (m), 679 (s), 654 (m), 585 (m), 557 (m), 504 (w), 444 (m), 417 (w), 407 (w) cm−1. MS (ESI, DCM/MeOH): m/z: calculated for C10H17O2PS [M + Na]+: 255.1; found: 255.0. C10H17O2PS (232.28): calculated: C 51.7%, H 7.38%; found: C 51.5%, H 7.40%.
Synthesis of 12c.
A solution of endo-12c (0.24 g, 0.62 mmol) in 5 ml diethyl ether was added to a suspension of LiAlH4 (0.049 g, 1.3 mmol) in 10 ml of diethyl ether at 0 °C. The cooling bath was removed and the solution was left to stir for 60 h. The reaction was quenched by adding 0.13 ml water and stirred for 30 min. The mixture was filtered, the solid was treated with 6 ml THF at 65 °C for 30 min and the solution was filtered again. The solvent was removed from the combined filtrates. The residue was taken up in 10 ml toluene, sulfur (0.050 g, 1.6 mmol) and three drops of triethylamine were added and the solution was stirred at 110 °C for one hour. After chromatographic workup (DCM/MeOH = 60
:
1 to 10
:
1, v/v) 12c was obtained as a white solid. Crystals suitable for X-ray diffraction measurements were obtained from DCM. Yield: 0.072 g, 45%. Rf (DCM/methanol = 40
:
1, v/v) = 0.08 – iodine. m.p.: 198–200 °C. [α]25D = −54.1° (c = 0.795 in DMSO). 1H NMR (400 MHz): δ = 1.54 (3H, s), 1.94 (3H, d, 4JH,P = 2.7 Hz), 2.00–2.10 (2H, m), 2.61–2.72 (1H, m), 2.85–2.98 (1H, m), 3.54–3.63 (1H, m), 3.73–3.82 (1H, m), 3.83–3.91 (1H, m), 4.20–4.31 (1H, m), 7.26–7.30 (2H, m), 7.34 (1H, t, 3JH,H = 7.4 Hz), 7.42 (2H, t, 3JH,H = 7.4 Hz) ppm. 13C{1H} NMR (101 MHz): δ = 16.0 (d, 3JC,P = 11.6 Hz), 20.3 (d, 3JC,P = 16.9 Hz), 45.1 (d, 1JC,P = 41.6 Hz), 48.4 (d, 2JC,P = 22.8 Hz), 52.6 (s), 53.6 (d, 1JC,P = 56.8 Hz), 59.5 (d, 2JC,P = 6.2 Hz), 59.8 (d, 3JC,P = 3.7 Hz), 127.8 (d, 5JC,P = 1.6 Hz), 128.3 (s), 129.5 (d, 3JC,P = 5.1 Hz), 132.1 (d, 2JC,P = 9.5 Hz), 132.8 (d, 1JC,P = 64.3 Hz), 154.6 (d, 2JC,P = 13.7 Hz) ppm. 31P{1H} NMR (162 MHz): δ = 56.4 ppm. IR (KBr):
= 3424 (s), 2969 (s, νC–H), 2927 (s, νC–H), 1631 (m), 1606 (s), 1485 (m), 1456 (m), 1440 (s), 1385 (w), 1372 (w), 1344 (w), 1319 (w), 1261 (m), 1223 (w), 1151 (w), 1135 (m), 1106 (m), 1176 (m), 1037 (s), 938 (w), 902 (w), 883 (w), 802 (m), 793 (m), 785 (m), 763 (m), 734 (w), 708 (s), 638 (m), 563 (w), 502 (w), 495 (w), 449 (w) cm−1. MS (ESI, DCM/MeOH): m/z: calculated for C16H21O2PS [M + Na]+: 331.1; found: 331.1. C16H21O2PS (308.38): calculated: C 62.3%, H 6.86%; found: C 61.23%, H 6.50%.
Synthesis of (R)-13c and (S)-13c.
A solution of endo-9c (1.2 g, 2.6 mmol) in 5 ml THF was added to a suspension of LiAlH4 (0.19 g, 5.0 mmol) in 10 ml THF at 0 °C. The cooling bath was removed and the solution was left to stir for 5 h. The reaction was quenched by the addition of 4 ml of an aqueous sodium hydroxide solution (32%). 15 ml of THF and MgSO4 were added, the suspension was transferred onto a frit and the white solid was continuously extracted with THF for 12 h. Sulfur (0.09 g, 2.8 mmol) and three drops of triethylamine were added and the solution was left to stir for 11 days. After chromatographic workup (DCM/MeOH = 50
:
1) a mixture of (R)-13c and (S)-13c was obtained as a white solid. Analytical amounts of the separated diastereomers as well as crystals suitable for X-ray diffraction measurements were obtained by recrystallisation from DCM/hexanes. Yield: 0.37 g, 45%.
(S)-13c: Rf (DCM/methanol = 40
:
1, v/v) = 0.14 – iodine. m.p.: 167–169 °C. [α]25D = +113° (c = 1.86 in CHCl3). 1H NMR (300 MHz): δ = 0.85 (3H, s), 1.21 (3H, s), 2.26 (1H, ddd, 2JH,H = 13.4 Hz, 2JH,P = 9.9 Hz, 4JH,H = 2.2 Hz), 2.37 (1H, dd, 2JH,H = 13.4 Hz, 2JH,P = 6.5 Hz), 2.52–2.67 (2H, m), 2.67–2.84 (1H, m), 3.55 (1H, dd, 2JH,P = 13.9 Hz, 4JH,H = 2.2 Hz), 3.99–4.19 (3H, m), 4.21–4.37 (1H, m), 7.17–7.42 (5H, m) ppm. 13C{1H} NMR (76 MHz): δ = 18.8 (d, 3JC,P = 15.1 Hz), 20.9 (d, 3JC,P = 2.6 Hz), 40.7 (d, 1JC,P = 47.7 Hz), 45.1 (d, 1JC,P = 49.7 Hz), 46.9 (d, 2JC,P = 2.3 Hz), 51.5 (d, 2JC,P = 17.6 Hz), 55.1 (d, 1JC,P = 42.1 Hz), 58.6 (s), 66.3 (s), 92.6 (d, 2JC,P = 7.1 Hz), 127.5 (s (br)), 128.6 (s (br)), 134.2 (d, 2JC,P = 2.9 Hz) ppm. 31P{1H} NMR (162 MHz): δ = 50.5 ppm. IR (KBr):
= 3432 (s), 3092 (w, νC–H), 3027 (w, νC–H), 2998 (m, νC–H), 2986 (s, νC–H), 2967 (s, νC–H), 2932 (m, νC–H), 2891 (s, νC–H), 2822 (m, νC–H), 2758 (w, νC–H), 1950 (w), 1800 (w), 1602 (w), 1498 (m), 1469 (m), 1444 (s), 1422 (m), 1387 (m), 1380 (m), 1366 (w), 1341 (s), 1287 (w), 1259 (m), 1234 (m), 1194 (w), 1174 (w), 1144 (m), 1127 (s), 1114 (s), 1087 (s), 1061 (m), 1045 (s), 1034 (m), 1009 (s), 990 (s), 953 (s), 920 (w), 877 (s), 864 (m), 841 (s), 811 (w), 789 (m), 775 (s), 765 (s), 707 (s), 679 (m), 662 (w), 627 (m), 615 (m), 583 (m), 566 (m), 499 (m), 459 (m), 426 (w) cm−1. MS (ESI, DCM/MeOH): m/z: calculated for C16H21O2PS [M + Na]+: 331.1; found: 333.1. C16H21O2PS (308.38): calculated: C 62.3%, H 6.86%; found: C 62.2%, H 6.64%.
(R)-13c: Rf (DCM/methanol = 40
:
1, v/v) = 0.16 – iodine. m.p.: 188–190 °C. Due to the small amount of pure (R)-13b, only NMR and MS data were obtained. 1H NMR (300 MHz): δ = 1.34 (3H, s), 1.42 (3H, d, 4JH,P = 1.8 Hz), 1.94 (1H, dd, 2JH,P = 6.9 Hz, 2JH,H = 13.0 Hz), 2.14 (1H, dd, 2JH,P = 8.2 Hz, 2JH,H = 13.0 Hz), 2.14 (1H, s (br)), 2.57–2.68 (1H, m), 2.71–2.86 (1H, m), 3.17 (1H, d, 2JH,P = 19.8 Hz), 3.79–3.95 (1H, m), 3.99–4.13 (3H, m), 7.27–7.38 (3H, m), 7.63–7.74 (2H, m) ppm. 13C{1H} NMR (76 MHz): δ = 19.2 (d, 3JC,P = 14.5 Hz), 24.2 (d, 3JC,P = 8.8 Hz), 39.5 (d, 1JC,P = 54.3 Hz), 46.3 (d, 1JC,P = 43.0 Hz), 47.5 (d, 2JC,P = 2.1 Hz), 51.8 (d, 2JC,P = 17.9 Hz), 56.0 (d, 1JC,P = 42.0 Hz), 58.3 (d, 2JC,P = 3.4 Hz), 65.8 (s), 86.7 (d, 2JC,P = 3.2 Hz), 127.3 (d, 5JC,P = 2.2 Hz), 128.2 (s), 130.3 (d, 3JC,P = 6.7 Hz), 132.2 (d, 2JC,P = 4.5 Hz) ppm. 31P{1H} NMR (162 MHz): δ = 51.0 ppm. MS (ESI, DCM/MeOH): m/z: calculated for C16H21O2PS (308.38): [M + Na]+: 331.1; found: 333.1.
Synthesis of 14a.
Chlorodiphenylphosphane (0.067 g, 0.30 mmol) was added to a solution of 13a (0.048 g, 0.21 mmol) and triethylamine (0.10 ml, 0.72 mmol) in 6 ml DCM at 0 °C. After 15 min the cooling bath was removed and the solution was stirred for another 30 min at room temperature. Subsequently, sulfur (0.022 g, 0.67 mmol) was added and the solution stirred for 16 h. Then the reaction was quenched with 20 μl of isopropanol. Chromatographic workup (hexanes/diethyl ether = 1
:
1 to 1
:
3, v/v) gave 14a as a white solid. Crystals suitable for X-ray diffraction measurements were obtained from DCM/hexanes at 4 °C. Yield: 0.086 g, 93%. Rf (hexanes/diethyl ether = 1
:
3, v/v) = 0.32 – UV light, iodine. m.p.: 184–186 °C. [α]25D = −9.6° (c = 1.77 in chloroform). 1H NMR (400 MHz): δ = 1.19 (3H, s), 1.24 (3H, s), 1.82–1.98 (2H, m), 2.00–2.12 (1H, m), 2.18–2.32 (1H, m), 2.45–2.58 (1H, m), 2.77–2.93 (1H, m), 3.93 (1H, dd, 2JH,H = 10.2 Hz, 3JH,H = 5.8 Hz), 4.19 (1H, d, 2JH,H = 10.2 Hz), 4.27–4.40 (1H, m), 4.40–4.50 (1H, m), 7.38–7.58 (6H, m), 7.81–7.99 (4H, m) ppm. 13C{1H} NMR (101 MHz): δ = 18.2 (d, 3JC,P = 15.7 Hz), 23.9 (d, 3JC,P = 7.3 Hz), 40.3 (d, 1JC,P = 52.2 Hz), 41.6 (d, 1JC,P = 44.8 Hz), 43.1 (dd, 1JC,P = 46.5 Hz, 3JC,P = 9.1 Hz), 47.3 (d, 2JC,P = 2.0 Hz), 51.5 (d, 2JC,P = 18.7 Hz), 60.3 (dd, 2JC,P = 5.3 Hz, 2JC,P = 5.3 Hz), 66.2 (s), 86.3 (d, 2JC,P = 1.4 Hz), 128.6 (dd, 2JC,P = 13.5 Hz, 6JC,P = 2.5 Hz), 131.3 (dd, 3JC,P = 11.6 Hz, 7JC,P 5.7 Hz), 132.1 (d, 4JC,P = 2.4 Hz), 133.6 (dd, 1JC,P = 109.8 Hz, 5JC,P = 6.2 Hz) ppm. 31P{1H} NMR (162 MHz): δ = 44.2 (s), 83.0 (s) ppm. IR (KBr):
= 3429 (s), 3056 (m), 2974 (m, νC–H), 2887 (m, νC–H), 2346. (w), 1631 (w), 1493 (w), 1479 (m), 1437 (s), 1405 (m), 1382 (m), 1314 (w), 1263 (w), 1199 (w), 1114 (s), 1080 (s), 1014 (s), 993 (s), 961 (m), 929 (w), 888 (m), 848 (m), 814 (m), 801 (m), 780 (m), 756 (m), 728 (s), 719 (s), 695 (s), 670 (s), 638 (s), 615 (w), 562 (w), 530 (s), 503 (m), 471 (w), 458 (w), 439 (w), 421 (w), 405 (w) cm−1. MS (ESI, DCM/MeOH): m/z: calculated for C22H26O2P2S2 [M + Na]+: 471.1; found: 471.2. C22H26O2P2S2 (448.52): calculated: C 58.9%, H 5.84%; found: C 58.8%, H 5.86%.
Synthesis of 14b.
Chlorodimesitylphosphane (0.81 g, 2.61 mmol) was added to a solution of 13a (0.41 g, 1.8 mmol) and triethyl amine (0.74 ml, 5.3 mmol) in 20 ml DCM at 0 °C. After 15 min the cooling bath was removed and the solution was stirred for another 30 min at room temperature. Subsequently, sulfur (excess) was added and the solution stirred for 16 h after which the reaction was quenched with 100 μl of isopropanol. Chromatographic workup (hexanes/diethyl ether = 2
:
1 to 1
:
1, v/v) gave 14b as a white solid. Crystals suitable for X-ray diffraction measurements were obtained from DCM/hexanes at 4 °C. Yield: 0.28 g, 30%. Rf (hexanes/diethyl ether = 1
:
3, v/v) = 0.41 – UV light, iodine. m.p.: 88–90 °C. [α]25D = −18.6° (c = 1.67 in chloroform). 1H NMR (300 MHz): δ = 1.18 (3H, s), 1.23 (3H, s), 1.81–1.96 (2H, m), 1.97–2.25 (2H, m), 2.25 (6H, s), 2.18–2.44 (1H, m), 2.37 (12H, d, 4JH,P = 9.0 Hz), 2.72–2.95 (1H, m), 3.82 (1H, dd, 2JH,H = 10.2 Hz, 3JH,H = 5.8 Hz), 4.13 (1H, d, 2JH,H = 10.2 Hz), 4.13–4.26 (1H, m), 4.38–4.50 (1H, m), 6.82 (4H, s) ppm. 13C{1H} NMR (76 MHz): δ = 18.3 (d, 3JC,P = 16.1 Hz), 20.9 (s), 23.2–23.4 (m), 23.8 (d, 3JC,P = 7.1 Hz), 39.7–41.7 (m), 43.5 (dd, 1JC,P = 45.5 Hz, 3JC,P = 8.2 Hz), 47.3 (s), 51.3 (d, 2JC,P = 18.8 Hz), 58.8 (dd, 2JC,P = 11.2 Hz, 2JC,P = 4.8 Hz), 66.0 (s), 86.3 (s), 129.1–130.9 (m), 131.1–132.3 (m), 140.2–141.4 (m) ppm. 31P{1H} NMR (162 MHz): δ = 43.5 (s), 83.0 (s) ppm. IR (KBr):
= 3431 (s), 2965 (s, νC–H), 2930 (s, νC–H), 2346 (w), 2138 (w), 1605 (w), 1507 (w), 1451 (m), 1383 (m), 1262 (m), 1080 (s), 1021 (s), 888 (w), 851 (w), 802 (m), 726 (m), 687 (s), 641 (m), 556 (m), 465 (m), 439 (m), 431 (m), 422 (w), 416 (w) cm−1. MS (ESI, DCM/MeOH): m/z: calculated for C28H38O2P2S2 [M + Na]+: 555.2; found: 555.3. C28H38O2P2S2 (532.68): calculated: C 63.1%, H 7.19%; found: C 63.6%, H 7.45%.
Synthesis of 15.
Compound 13a (2.65 g, 11.4 mmol), dissolved in 40 ml DCM, was added to a solution of Br2PPh3 (7.37 g, 17.5 mmol) in 40 ml of DCM at 0 °C. The solution was allowed to warm up to room temperature overnight. The reaction was quenched by addition of 35 ml water and the phases were separated. The aqueous layer was extracted two times with 20 ml DCM, the combined organic layers were washed with brine and dried over MgSO4. After chromatographic workup (hexanes/diethyl ether = 1
:
1 to 1
:
2, v/v) 15 was obtained as a white solid. Yield: 3.27 g, 97%. Rf (hexanes/diethyl ether = 1
:
2, v/v) = 0.44 – iodine. m.p.: 159–160 °C. [α]25D = +50.0° (c = 2.50 in toluene). 1H NMR (300 MHz): δ = 1.24 (3H, s), 1.27 (3H, s), 1.83–2.26 (4H, m), 2.44–2.62 (1H, m), 2.66–2.91 (1H, m), 3.26–3.56 (1H, m), 3.89–4.15 (3H, m) ppm. 13C{1H} NMR (76 MHz): δ = 18.3 (d, 3JC,P = 16.2 Hz), 23.8 (d, 3JC,P = 7.4 Hz), 27.7 (d, 2JC,P = 6.0 Hz), 39.3–41.7 (m), 45.4 (d, 1JC,P = 41.5 Hz), 47.1 (d, 2JC,P = 2.4 Hz), 51.2 (d, 2JC,P = 18.5 Hz), 65.7 (s), 86.4 (d, 2JC,P = 1.3 Hz) ppm. 31P{1H} NMR (162 MHz): δ = 46.7 ppm. IR (KBr):
= 3430 (s), 2987 (m, νC–H), 2962 (m, νC–H), 2887 (m, νC–H), 2194 (w), 1631 (m), 1498 (w), 1436 (w), 1401 (m), 1386 (w), 1375 (w), 1314 (w), 1262 (m), 1239 (m), 1205 (w), 1185 (w), 1162 (m), 1134 (s), 1051 (s), 1017 (m), 932 (w), 912 (w), 887 (w), 873 (m), 784 (s), 740 (m), 670 (s), 624 (m), 578 (m), 538 (w), 524 (w), 496 (w), 475 (w), 460 (w), 445 (w), 414 (w) cm−1. MS (ESI, MeOH): m/z: calculated for C10H16BrOPS [M + Na]+: 317.0 (79Br), 319.0 (81Br); found: 317.0 (79Br), 319.0 (81Br). C10H16BrOPS (295.18): calculated: C 40.7%, H 5.46%; found: C 41.0%, H 5.54%.
Characterisation of the side product 16.
Product 16 was obtained as a side product in small amounts during test reactions for the nucleophilic substitution of 15. Hence, only NMR and MS data were obtained. Rf (hexanes/diethyl ether = 2
:
1, v/v) = 0.44 – iodine. 1H NMR (300 MHz): δ = 1.22 (3H, s), 1.32 (3H, s), 1.94–2.21 (4H, m), 2.82–2.95 (1H, m), 3.80 (1H, d, 2JH,H = 8.5 Hz), 4.20 (1H, dd, 2JH,H = 8.5 Hz, 3JH,H = 5.7 Hz), 5.81 (1H, d, 3JH,P = 42.0 Hz), 6.03 (1H, d, 3JH,P = 22.0 Hz) ppm. 13C{1H} NMR (76 MHz): δ = 17.9 (d, 3JC,P = 14.1 Hz), 24.0 (d, 3JC,P = 7.6 Hz), 40.5 (d, 1JC,P = 52.9 Hz), 43.4 (d, 1JC,P = 48.3 Hz), 50.5 (d, 2JC,P = 14.6 Hz), 52.7 (d, 2JC,P = 15.6 Hz), 73.0 (s), 86.6 (s), 124.0 (d, 2JC,P = 8.2 Hz), 148.5 (d, 1JC,P = 68.1 Hz) ppm. 31P{1H} NMR (162 MHz): δ = 37.8 ppm. MS (ESI, DCM/MeOH): m/z: calculated for C10H15OPS [M + Na]+: 237.1; found: 237.0.
Synthesis of 17.
A mixture of 3,4-dimethyl-1-phenylphosphole (0.21 g, 1.1 mmol) and pieces of lithium (excess) in 10 ml THF was stirred at room temperature for 3–4 hours (conversion was monitored by TLC, with phosphomolybdic acid). The deep brown solution was filtered, tert-butyl chloride (0.16 ml, 1.5 mmol) was added and the solution was stirred at 65 °C for one hour. Afterwards, a solution of 15 (0.16 g, 0.56 mmol) in 20 ml THF was added at 0 °C over five minutes and the mixture was stirred for another 10 min at room temperature. The reaction was quenched by addition of 50 μl water, stirred for ten minutes, treated with MgSO4 and filtered. Sulfur (0.054 g, 1.7 mmol) and three drops of triethylamine were added and the reaction mixture was stirred for 13 h. Chromatographic workup of the crude product (DCM/diethyl ether = 40
:
1 to 15
:
1, v/v) yielded 17 as a white solid. Yield: 0.20 g, 98%. Rf (DCM/diethyl ether = 20
:
1, v/v) = 0.25 – iodine. m.p.: 208–210 °C. [α]25D = +16.9° (c = 1.42 in toluene). 1H NMR (300 MHz): δ = 1.20–1.28 (6H, m), 1.79–2.31 (5H, m), 2.08 (6H, s), 2.62–2.75 (3H, m), 3.87–4.12 (2H, m), 5.95 (1H, d, 2JH,P = 31.0 Hz), 6.09 (1H, d, 2JH,P = 30.6 Hz) ppm. 13C{1H} NMR (76 MHz): δ = 17.2–17.7 (m), 18.5 (dd, 3JC,P = 12.3 Hz, 6JC,P = 3.4 Hz), 23.7 (d, 3JC,P = 5.5 Hz), 23.9–25.7 (m), 37.0–39.3 (m), 38.1–42.1 (m), 47.2 (s), 51.5 (dd, 2JC,P = 15.2 Hz, 5JC,P = 4.1 Hz), 66.5 (s), 86.3 (s), 122.7–125.6 (m), 153.4–154.1 (m) ppm. 31P{1H} NMR (162 MHz): δ = 50.5–51.6 (m) ppm. IR (KBr):
= 3452 (s), 2965 (s, νC–H), 2873 (m, νC–H), 2345 (w), 2086 (w), 1631 (s), 1539 (w), 1450 (m), 1402 (w), 1386 (w), 1262 (m), 1137 (m), 1085 (s), 1048 (s), 865 (w), 801 (s), 741 (m), 674 (m), 643 (m), 587 (w), 502 (w), 482 (w), 473 (w), 456 (w), 444 (w), 430 (w), 417 (w), 405 (w) cm−1. MS (ESI, DCM/MeOH): m/z: calculated for C16H24OP2S2 [M + Na]+: 381.1; found: 381.1. C16H24OP2S2 (326.37): calculated: C 53.6%, H 6.75%; found: C 53.5%, H 6.67%.
Synthesis of 18.
A 1.64 M solution of n-butyl lithium in n-hexane (0.990 ml, 1.62 mmol) was added to a solution of P(BH3)HPh2 (0.382 g, 1.92 mmol) in 8 ml of diethyl ether at 0 °C. After 5 min at this temperature, the solution was added to a suspension of 15 (0.435 g, 1.47 mmol) in 5 ml diethyl ether at 0 °C. The mixture was stirred for 18 h. Chromatographic workup of the crude product (hexanes/diethyl ether = 1
:
1, v/v) yielded 18 as a white solid. Yield: 0.520 g, 85%. Rf (hexanes/diethyl ether = 1
:
1, v/v) = 0.43 – iodine. m.p.: 216–220 °C. [α]25D = −11.3° (c = 1.16 in toluene). 1H NMR (400 MHz): δ = 1.19 (3H, s), 1.23 (3H, s), 0.30–1.50 (3H, m, B–H), 1.79–2.01 (3H, m), 2.06–2.42 (3H, m), 2.56–2.68 (1H, m), 3.11–3.26 (1H, m), 3.94 (1H, dd, 2JH,H = 10.8 Hz, 3JH,H = 5.4 Hz), 4.10 (1H, d, 2JH,H = 10.8 Hz), 7.37–7.58 (6H, m), 7.60–7.72 (2H, m), 7.86–7.98 (2H, m) ppm. 13C{1H} NMR (76 MHz): δ = 18.6 (d, 3JC,P = 18.9 Hz), 19.0 (d, 2JC,P = 35.3 Hz), 23.9 (d, 3JC,P = 7.5 Hz), 37.2 (d, 1JC,P = 46.9 Hz), 38.8 (d, 1JC,P = 51.0 Hz), 41.0 (d, 1JC,P = 44.2 Hz), 47.8 (d, 2JC,P = 2.4 Hz), 51.5 (d, 2JC,P = 19.9 Hz), 67.0 (s), 86.2 (d, 2JC,P = 2.0 Hz), 126.5 (d, 1JC,P = 55.5 Hz), 128.6–129.7 (m), 130.3 (d, 1JC,P = 55.6 Hz), 131.3–132.3 (m), 133.0 (d, J = 9.2 Hz) ppm. 31P{1H} NMR (162 MHz): δ = 15.1–16.8 (m (br)), 51.8 (d, 3JP,P = 47.3 Hz) ppm. IR (KBr):
= 3438 (m), 3061 (w), 2989 (w, νC–H), 2969 (m, νC–H), 2932 (m, νC–H), 2888 (w, νC–H), 2383 (s, νB–H), 1637 (w), 1437 (m), 1421 (w), 1411 (w), 1400 (w), 1381 (w), 1262 (w), 1198 (w), 1161 (w), 1131 (m), 1107 (m), 1064 (s), 1046 (s), 1013 (w), 984 (w), 866 (w), 783 (m), 769 (m), 751 (m), 737 (s), 719 (w), 705 (s), 695 (m), 667 (m), 655 (s), 595 (w), 501 (w), 467 (w) cm−1. MS (ESI, DCM/MeOH): m/z: calculated for C22H29BOP2S [M + Na]+: 437.1; found: 437.3. C22H29BOP2S (414.29): calculated: C 63.78%, H 7.06%; found: C 63.20%, H 7.04%.
Synthesis of 19.
LiAlH4 (0.040 g, 1.1 mmol) was added to a solution of 18 (0.055 g, 0.13 mmol) in 3 ml of THF and the suspension was stirred for 20 h at 60 °C. The reaction was cooled to 0 °C and quenched by addition of 0.3 ml of an aqueous KOH solution (20%). The mixture was diluted with 3 ml of diethyl ether, dried over MgSO4, filtered and the residue was washed three times with 3 ml diethyl ether. From the combined organic layers the solvent was removed in vacuum and the residue was dissolved in 2 ml THF. A 1 M solution of borane in THF (0.65 ml, 0.65 mmol) was added and the solution was stirred for 18 h. Chromatographic workup of the crude product (hexanes/diethyl ether = 3
:
2 v/v) yielded 19 as a white solid. Yield: 0.041 g, 78%. Rf (hexanes/diethyl ether = 3
:
2, v/v) = 0.46 – iodine. m.p.: 159–164 °C. [α]25D = −16.9° (c = 0.92 in toluene). 1H NMR (300 MHz): δ = 1.19 (3H, s), 1.24 (3H, s), 0.00–2.10 (6H, m, B–H), 1.55–1.79 (3H, m), 1.83–1.97 (1H, m), 2.10–2.42 (2H, m), 2.48–2.62 (1H, m), 2.80–3.00 (1H, m), 3.89 (1H, dd, 2JH,H = 10.8 Hz, 3JH,H = 5.0 Hz), 4.01 (1H, d, 2JH,H = 10.8 Hz), 7.37–7.68 (8H, m), 7.81–7.91 (2H, m) ppm. 13C{1H} NMR (76 MHz): δ = 18.6 (d, 3JC,P = 10.0 Hz), 20.3 (d, 1JC,P = 35.4 Hz), 24.3 (d, 3JC,P = 4.3 Hz), 32.7 (d, 1JC,P = 28.8 Hz), 34.1 (d, 1JC,P = 33.2 Hz), 35.4 (d, 1JC,P = 27.2 Hz), 47.9 (d, 2JC,P = 3.6 Hz), 56.9 (d, 2JC,P = 4.5 Hz), 66.1 (s), 86.6 (d, 2JC,P = 4.2 Hz), 126.5 (d, 1JC,P = 56.6 Hz), 129.0–129.5 (m), 130.2 (d, 1JC,P = 55.5 Hz), 131.7 (d, J = 9.4 Hz), 133.0 (d, J = 9.3 Hz) ppm. 31P{1H} NMR (162 MHz): δ = 15.7–17.8 (m (br)), 28.8–30.0 (m (br)) ppm. IR (KBr):
= 3422 (m), 3060 (w), 2967 (m, νC–H), 2384 (s, νB–H), 1637 (w), 1489 (w), 1437 (m), 1419 (w), 1263 (m), 1136 (m), 1106 (m), 1062 (s), 1046 (s), 984 (w), 928 (w), 868 (w), 794 (m), 751 (m), 737 (m), 704 (m), 660 (w), 635 (w), 593 (w), 499 (w), 469 (w), 456 (w), 430 (w), 419 (w), 410 (w) cm-1. MS (ESI, DCM/MeCN): m/z: calculated for C22H32B2OP2 [M + Na]+: 419.2; found: 419.4. C22H32B2OP2 (396.06): calculated: C 66.72%, H 8.14%; found: C 66.13%, H 8.18%.
In situ deboranation of 19.
A solution of 19 (0.010 g, 0.025 mmol) and morpholine (20 μl, 0.23 mmol) in 0.7 ml of CDCl3 was heated at 60 °C. The reaction was monitored by 31P{1H} NMR spectroscopy. A full conversion to 20 was observed after 6 h. 31P{1H} NMR (162 MHz)): δ = −36.9 (d, 3JP,P = 45.6 Hz), −18.3 (d, 3JP,P = 45.6 Hz) ppm.
Crystallographic data
The data were collected on a Gemini diffractometer (Agilent Technologies) using Mo-Kα radiation (λ = 71.073 pm), ω-scan rotation. Data reduction was performed with the CrysAlisPro (CrysAlisPro: Data collection and data reduction software package, Agilent Technologies) including the program SCALE3 ABSPACK (SCALE3 ABSPACK: Empirical absorption correction using spherical harmonics) for empirical absorption correction. The structures were solved by direct methods with SIR92.22 The refinement of all non-hydrogen atoms was performed with SHELXL-97.23 The structure figures were generated with Diamond.24 CCDC 990595 (endo-9d), 989748 (12a), 989750 (12b), 989749 (13a), 989751 ((R)-13b), 989752 ((S)-13b), 989753 (14a), 989754 (14b) and 989755 (18) contain the supplementary crystallographic data for this paper.
Theoretical methods
All calculations were carried out with the Gaussian 09 program package,25 at the M06-2X/MG3S level of theory.26 The polarisable continuum model (PCM) using the integral equation formalism variant, as implemented in GAUSSIAN 09, has been used to account for solvent effects.
Acknowledgements
This work was supported by the Saxon State Ministry of Science and the Arts (doctoral fellowship for T.M. (Landesgraduiertenstipendium)). Support from the Graduate School “Building with Molecules and Nano-objects (BuildMoNa)” and the EU-COST Action CM0802 PhoSciNet is gratefully acknowledged.
We thank Dr. P. Lönnecke (X-ray crystallography) and Dr. M. Findeisen (NMR spectroscopy) for their support.
Notes and references
-
(a) W. S. Knowles, Acc. Chem. Res., 1983, 16, 106 CrossRef CAS;
(b) W. S. Knowles, M. J. Sabacky, B. D. Vineyard and D. J. Weinkauff, J. Am. Chem. Soc., 1975, 97, 2567 CrossRef CAS;
(c) W. S. Knowles and M. J. Sabacky, Chem. Commun., 1968, 1445 RSC;
(d) B. D. Vineyard, W. S. Knowles, M. J. Sabacky, G. L. Bachman and D. J. Weinkauff, J. Am. Chem. Soc., 1977, 99, 5946 CrossRef CAS.
-
(a)
Asymmetric Synthesis – The Essentials, ed. M. Christmann and S. Bräse, Wiley-VCH, Weinheim, 2nd edn, 2007 Search PubMed;
(b)
Asymmetric Synthesis – More Methods and Applications, ed. M. Christmann and S. Bräse, Wiley-VCH, Weinheim, vol. 2, 2012 Search PubMed.
-
(a) P. Guga, A. Okruszek and W. J. Stec, Top. Curr. Chem., 2002, 220, 169 CrossRef CAS;
(b) K. V. L. Crepy and T. Imamoto, Top. Curr. Chem., 2003, 229, 1 CrossRef CAS;
(c) O. I. Kolodiazhnyi, Tetrahedron: Asymmetry, 2012, 23, 1 CrossRef CAS PubMed;
(d) A. Grabulosa, J. Granell and G. Muller, Coord. Chem. Rev., 2007, 251, 25 CrossRef CAS PubMed.
-
(a) F. Mathey, Angew. Chem., Int. Ed., 2003, 42, 1578 CrossRef CAS PubMed;
(b) M. Yoshifuji, Pure Appl. Chem., 1999, 71, 503 CrossRef CAS;
(c) M. Yoshifuji, Pure Appl. Chem., 2005, 77, 2011 CrossRef CAS;
(d) M. Yoshifuji, Bull. Chem. Soc. Jpn., 1997, 70, 2881 CrossRef CAS;
(e) M. Yoshifuji, Phosphorus, Sulfur Silicon Relat. Elem., 2002, 177, 1827 CrossRef CAS PubMed;
(f) M. Yoshifuji, J. Organomet. Chem., 2000, 611, 210 CrossRef CAS;
(g) A. Marinetti, S. Bauer, L. Ricard and F. Mathey, Organometallics, 1990, 9, 793 CrossRef CAS;
(h) F. Mathey, Acc. Chem. Res., 1992, 25, 90 CrossRef CAS;
(i) F. Mathey, A. Marinetti, S. Bauer and P. Le Floch, Pure Appl. Chem., 1991, 63, 855 CrossRef CAS;
(j) F. Mathey, Angew. Chem., Int. Ed., 2003, 115, 1616 CrossRef PubMed;
(k)
M. Regitz, O. J. Scherer and R. Appel, Multiple Bonds and Low Coordination in Phosphorus Chemistry, G. Thieme Verlag, 1990 Search PubMed;
(l)
K. B. Dillon, F. Mathey and J. F. Nixon, Phosphorus: the Carbon Copy, Wiley, 1998 Search PubMed.
-
(a) R. de Vaumas, A. Marinetti, L. Ricard and F. Mathey, J. Am. Chem. Soc., 1992, 114, 261 CrossRef CAS;
(b) X. Sava, A. Marinetti, L. Ricard and F. Mathey, Eur. J. Inorg. Chem., 2002, 1657 CrossRef CAS.
- T. Möller, M. B. Sárosi and E. Hey-Hawkins, Chem. – Eur. J., 2012, 18, 16604 CrossRef PubMed.
-
(a) F. Mathey, Acc. Chem. Res., 2004, 37, 954 CrossRef CAS PubMed;
(b) C. Charrier, H. Bonnard, G. de Lauzon and F. Mathey, J. Am. Chem. Soc., 1983, 105, 6871 CrossRef CAS;
(c) F. Mercier and F. Mathey, J. Organomet. Chem., 1984, 263, 55 CrossRef CAS;
(d) G. de Lauzon, C. Charrier, H. Bonnard and F. Mathey, Tetrahedron Lett., 1982, 23, 511 CrossRef CAS;
(e) P. le Goff, F. Mathey and L. Ricard, J. Org. Chem., 1989, 54, 4754 CrossRef CAS;
(f) F. Mathey, F. Mercier, C. Charrier, J. Fischer and A. Mitschler, J. Am. Chem. Soc., 1981, 103, 4595 CrossRef CAS;
(g) F. Mercier and F. Mathey, J. Organomet. Chem., 1993, 462, 103 CrossRef CAS;
(h) G. de Lauzon, C. Charrier, H. Bonnard, F. Mathey, J. Fischer and A. Mitschler, J. Chem. Soc., Chem. Commun., 1982, 1272 RSC.
- D. A. Evans, K. T. Chapman and J. Bisaha, J. Am. Chem. Soc., 1988, 110, 1238 CrossRef CAS.
- M. L. Sierra, N. Maigrot, C. Charrier, L. Ricard and F. Mathey, Organometallics, 1991, 10, 2835 CrossRef CAS.
-
(a) B. L. Feringa and J. C. de Jong, J. Org. Chem., 1988, 53, 1125 CrossRef CAS;
(b) J. F. G. A. Jansen and B. L. Feringa, Tetrahedron: Asymmetry, 1990, 1, 719 CrossRef CAS.
-
F. Mathey, F. Mercier, M. Spagnol, F. Robin and V. Mouries, WO9839345A1, 1998.
-
(a) S. N. Steinmann, P. Vogel, Y. Mo and C. Corminboeuf, Chem. Commun., 2011, 47, 227 RSC;
(b) M. C. Holthausen and W. Koch, J. Phys. Chem., 1993, 97, 10021 CrossRef CAS.
-
(a) B. R. Pool and J. M. White, Org. Lett., 2000, 2, 3505 CrossRef CAS PubMed;
(b) D. Birney, T. K. Lim, J. H. P. Koh, B. R. Pool and J. M. White, J. Am. Chem. Soc., 2002, 124, 5091 CrossRef CAS PubMed.
-
(a) L. Nyulászi and P. von Ragué Schleyer, J. Am. Chem. Soc., 1999, 121, 6872 CrossRef;
(b) S. N. Steinmann, D. F. Jana, J. I. C. Wu, P. von Ragué Schleyer, Y. Mo and C. Corminboeuf, Angew. Chem., Int. Ed., 2009, 48, 9828 CrossRef CAS PubMed.
- T. Möller, P. Wonneberger, N. Kretzschmar and E. Hey-Hawkins, Chem. Commun., 2014, 50, 5826 RSC.
- R. K. Harris, E. D. Becker, S. M. Cabral De Menezes, R. Goodfellow and P. Granger, Concepts Magn. Reson., 2002, 14, 326 CrossRef CAS PubMed.
- A. Breque, F. Mathey and P. Savignac, Synthesis, 1981, 983 CrossRef CAS.
-
(a) S. Holand, M. Jeanjean and F. Mathey, Angew. Chem., Int. Ed. Engl., 1997, 36, 98 CrossRef CAS PubMed;
(b) S. Holand, M. Jeanjean and F. Mathey, Angew. Chem., Int. Ed. Engl., 1997, 109, 117 CrossRef PubMed.
- D. A. Evans, K. T. Chapman and J. Bisaha, J. Am. Chem. Soc., 1988, 110, 1238 CrossRef CAS.
-
(a) S. Freitag, J. Henning, H. Schubert and L. Wesemann, Angew. Chem., Int. Ed., 2013, 52, 5640 CrossRef CAS PubMed;
(b) S. Freitag, J. Henning, H. Schubert and L. Wesemann, Angew. Chem., Int. Ed., 2013, 125, 5750 CrossRef PubMed.
- A. Hinke and W. Kuchen, Phosphorus, Sulfur Relat. Elem., 1983, 15, 93 CrossRef CAS PubMed.
- A. Altomare, G. Cascarano, C. Giacovazzo, A. Guagliardi, M. C. Burla, G. Polidori and M. Camalli, J. Appl. Crystallogr., 1994, 27, 435 Search PubMed.
- G. M. Sheldrick, Acta Crystallogr., Sect. A: Fundam. Crystallogr., 2008, A64, 112 CrossRef PubMed.
-
K. Brandenburg, Diamond 3.2i, Crystal Impact GbR, Bonn, 2012 Search PubMed.
-
M. J. Frisch, G. W. Trucks, H. B. Schlegel, G. E. Scuseria, M. A. Robb, J. R. Cheeseman, G. Scalmani, V. Barone, B. Mennucci, G. A. Petersson, H. Nakatsuji, M. Caricato, X. Li, H. P. Hratchian, A. F. Izmaylov, J. Bloino, G. Zheng, J. L. Sonnenberg, M. Hada, M. Ehara, K. Toyota, R. Fukuda, J. Hasegawa, M. Ishida, T. Nakajima, Y. Honda, O. Kitao, H. Nakai, T. Vreven, J. A. Montgomery Jr., J. E. Peralta, F. Ogliaro, M. Bearpark, J. J. Heyd, E. Brothers, K. N. Kudin, V. N. Staroverov, R. Kobayashi, J. Normand, K. Raghavachari, A. Rendell, J. C. Burant, S. S. Iyengar, J. Tomasi, M. Cossi, N. Rega, J. M. Millam, M. Klene, J. E. Knox, J. B. Cross, V. Bakken, C. Adamo, J. Jaramillo, R. Gomperts, R. E. Stratmann, O. Yazyev, A. J. Austin, R. Cammi, C. Pomelli, J. W. Ochterski, R. L. Martin, K. Morokuma, V. G. Zakrzewski, G. A. Voth, P. Salvador, J. J. Dannenberg, S. Dapprich, A. D. Daniels, O. Farkas, J. B. Foresman, J. V. Ortiz, J. Cioslowski and D. J. Fox, Gaussian 09, Revision A.02, Gaussian, Inc., Wallingford CT, 2009 Search PubMed.
-
(a) Y. Zhao and D. Truhlar, Theor. Chem. Acc., 2008, 120, 215 CrossRef CAS;
(b) Y. Zhao and D. G. Truhlar, Acc. Chem. Res., 2008, 41, 157 CrossRef CAS PubMed;
(c) P. L. Fast, M. a. L. Sánchez and D. G. Truhlar, Chem. Phys. Lett., 1999, 306, 407 CrossRef CAS.
Footnotes |
† Dedicated to Professor Manfred Scheer on the occasion of his 60th birthday. |
‡ Electronic supplementary information (ESI) available: Detailed assignment of all NMR data for all compounds. CCDC 990595 and 989748–989755. For ESI and crystallographic data in CIF or other electronic format see DOI: 10.1039/c5dt02564h |
|
This journal is © The Royal Society of Chemistry 2016 |