Theoretical study on σ- and π-hole carbon⋯carbon bonding interactions: implications in CFC chemistry†
Received
19th September 2016
, Accepted 1st November 2016
First published on 3rd November 2016
Abstract
In this manuscript the ability of CO2 and several CFCs to establish noncovalent carbon⋯carbon interactions (termed as noncovalent carbon⋯carbon bonding) with atmospheric gases CO, ethene and ethyne has been studied at the RI-MP2/def2-TZVPD level of theory. We have used several CFCs (CFCl3, CF3Cl, CF2Cl2 and CH3F) and the CO2 molecule as σ- and π-hole carbon bond donors (electron poor carbon atoms). As electron rich moieties we have used CO, ethene and ethyne (electron rich carbon atom bearing molecules). We have also used Bader's theory of “atoms in molecules” to further analyse and characterize the noncovalent interactions described herein. Finally, we have analyzed possible cooperativity effects between the noncovalent carbon⋯carbon bonding and hydrogen bonding interactions in the case of ethyne.
Introduction
Noncovalent interactions are recognized as key players in modern chemistry.1 Actually, supramolecular chemists rely on their proper comprehension and rationalization in order to achieve progress in fields such as, molecular recognition2,3 and materials science.4 Hydrogen bonding and, more recently, halogen bonding are examples of well-known σ-hole interactions that play an important role in many chemical and biological environments.5,6 For instance, hydrogen bonding interactions are main driving forces in enzyme chemistry and protein folding.7 Consequently, both noncovalent forces have been widely studied from theoretical and experimental perspectives.8,9 Their similarities in both strength and directionality features stimulated a series of studies using the Cambridge Structural Database (CSD) in order to shed light on the impact of these interactions in crystal structures.10,11 Related to this, σ-hole interactions involving elements of Groups IV–VI have also earned recognition among the scientific society as an important addition to the family of well-established directional non-covalent interactions.12–14 Recently, it was demonstrated that atoms of Groups IV–VI (from tetrels to chalcogens) when covalently bonded are able to establish attractive interactions with electron rich entities through their positive electrostatic potential regions, which are attributed to the anisotropies of the electronic density distribution on the extensions of their covalent bonds.15–18 It was also shown that the factors that rule the σ-hole potentials are the same across Groups IV–VI.
As a natural consequence of the scientific curiosity over the σ-hole chemistry a novel and relatively unexplored group of noncovalent interactions emerged, called π-hole bonding. A π-hole is a region of positive electrostatic potential that is perpendicular to a portion of a molecular framework.19 Two pioneering works that exemplify the impact of π-hole interactions in chemical and biological systems should be emphasized. Firstly, Bürgi and Dunitz studied the trajectory along which a nucleophile attacks the π-hole of a C
O group20–22 and, secondly, Egli and co-workers studied and rationalized the ability of guanosine to behave as a π-hole donor in the crystal structure of Z-DNA.23 More recently, the study of π-hole interactions has been extended to acyl carbons24 and other entities involving pnicogen25–29 and chalcogen bearing compounds.30,31
Haloalkanes were widely used in industry as refrigerant agents, propellants and cleaning solvents until the 1970s.32 Related to this, chlorofluorocarbons (CFCs) are a family of haloalkane compounds mostly formed by hydrocarbons, particularly alkanes, covalently bonded with halogens such as chlorine or fluorine. Nowadays, they are well known to play a key role in the depletion process of the ozone layer, which is a vital natural defense against the incoming UV radiation.33 Several theoretical studies have been devoted to analyse the interaction between CFCs and ozone, carbon dioxide and nitrogen oxide molecules, as well as sulphur containing compounds.34–36 While CFCs are considered as an important source of carbon atoms,37 it is intriguing whether they can undergo carbon bonding interactions with other atmospheric gases, such as carbon monoxide or ethene and ethyne molecules.38,39 In this regard, we propose to coin the term dicarbon bond, since both the electron donor and acceptor belong are carbon atoms, thus resembling the concept of the dihydrogen bond.40 The concept of carbon–carbon interactions involving sp and sp2 carbons has been previously studied by Remya and coworkers.41
In this study, our purpose is to investigate the ability of CFCs and CO2 moieties to establish σ- and π-hole noncovalent carbon⋯carbon bonding interactions. In order to achieve this goal, we have used several CFCs (CFCl3, CCl3F, CH3F and CF2Cl2) as σ-hole carbon bond donor entities. We have used CO, ethene and ethyne as electron rich moieties (see Fig. 1). Particularly, in CO2 π-hole complexes, we have explored two different orientations (parallel and perpendicular) between both donor and acceptor molecules. Finally, we have also used Bader's theory of “atoms in molecules” to further describe and rationalize the interactions described above.
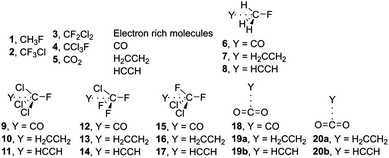 |
| Fig. 1 Compounds 1–5 and complexes 6–20 used in this study. For complexes 19 and 20 two possible orientations were considered; parallel (denoted as a) and perpendicular (denoted as b). | |
Theoretical methods
The energies of all complexes included in this study were computed at the RI-MP2/def2-TZVPD level of theory by means of the program TURBOMOLE version 7.0.42 Single point calculations at the CCSD(T)/def2-TZVP level of theory have been performed in order to give reliability to the RI-MP2 method. The MEP (molecular electrostatic potential) calculations have been performed at the MP2/def2-TZVP level of theory by means of the Gaussian09 calculation package.43 Frequency calculations have been performed at the RI-MP2/def2TZVPD level of theory and in all cases a true minima have been found. Moreover, all carbon⋯carbon complexes correspond to global minima apart from complex 8 that is a local minimum. The global minimum corresponds to an H-bonded complex CH3F⋯HCCH. Bader's “Atoms in molecules” theory has been used to study the interactions discussed herein by means of the AIMall calculation package.44 The calculations for wavefunction analyses were carried out at the MP2/def2-TZVP level of theory.
Results and discussion
MEPS study
As a preliminary study, we have computed the molecular electrostatic potential (MEP) surface of compounds 1 to 5 (see Fig. 2). As it can be observed, for compounds 1 to 4 the MEP surface showed the presence of a positive potential area located at the outermost region of the carbon atom, opposite to the C–X bond (σ-hole). Consequently, an attractive interaction with electron rich entities is expected. In addition, the MEP value of the σ-hole is more positive for compounds 1 and 2, thus expecting a stronger binding upon complexation from an electrostatic perspective. Moreover, the MEP values become less positive ongoing from compound 2 to 4, due to the difference in electronegativity between chlorine and fluorine atoms. In the case of compound 5, a positive potential region is observed on the tip of the carbon atom, perpendicularly located over the molecular plane (π-hole). The MEP value obtained is the most positive among compounds 1 to 5, thus expecting a stronger binding for π-complexes over the σ-hole set. Finally, among the electron donors, the most negative MEP value corresponds to the ethene molecule, thus expecting a slightly stronger binding over the other CO and ethyne molecules.
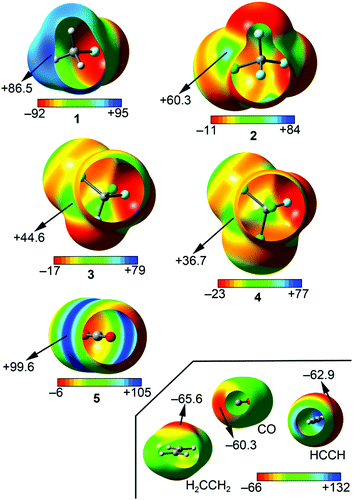 |
| Fig. 2 MEP (molecular electrostatic potential) surfaces for compounds 1 to 5. Energy values in kJ mol−1. | |
Energetic study
The interaction energies and equilibrium distances obtained for complexes 6 to 20 (see Fig. 3) studied herein are summarized in Table 1. The examination of the results indicates that the interaction energy values are weak but attractive in all cases, ranging from −11 to −4 kJ mol−1. Among the σ-hole complexes studied (6–17) the CFCl3 and CF2Cl2 ones (9–11 and 15–17, respectively) present stronger interaction energies, conversely to the MEP analysis that shows more positive values at the σ-hole of CH3F and CF3Cl molecules likely due to the greater polarizability of chlorine vs. fluorine. Moreover, CFCl3 and CF2Cl2 complexes present larger equilibrium distance values than those involving CH3F and CF3Cl molecules. On the other hand, for CH3F and CF3Cl complexes (6–8 and 12–14, respectively) comparable interaction energy values were obtained. For π-complexes involving CO2 (18 to 20b) the binding energy values are similar to those obtained for CCl3F and CF2Cl2 complexes. For some complexes we have computed the interaction energies at a higher level of theory in order to validate the computational method used herein. The values in parentheses summarized in Table 1 correspond to the interaction energies at the CCSD(T)/def2-TZVPD level of theory, which are in good agreement with the MP2 values.
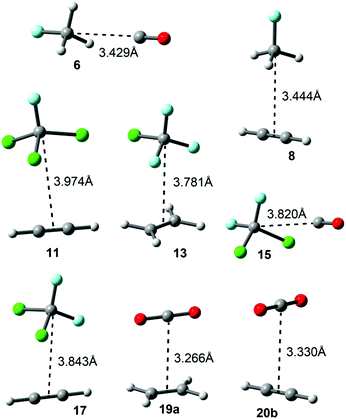 |
| Fig. 3 Optimized geometries of some representative complexes at the RI-MP2/def2-TZVPD level of theory. | |
Table 1 Interaction energies (ΔE, kJ mol−1), equilibrium distances (R, Å) and symmetry point groups used (Symm.) at the RI-MP2/def2-TZVPD level of theory for complexes 6 to 20
Complex |
ΔEa |
R
|
Symm. |
Values in parentheses correspond to the CCSD(T)/def2-TZVPD level of theory.
For complexes involving ethene and ethyne distances were measured from the C–C bond centroid.
|
6 (OC:CH3F) |
−4.3 |
3.429 |
C
3v
|
7 (H2CCH2:CH3F) |
−7.0 (−6.7) |
3.452 |
C
s
|
8 (HCCH:CH3F) |
−6.2 |
3.444 |
C
s
|
9 (OC:CCl3F) |
−4.8 (−4.3) |
3.922 |
C
3v
|
10 (H2CCH2:CCl3F) |
−10.8 |
3.937 |
C
s
|
11 (HCCH:CCl3F) |
−8.6 |
3.974 |
C
s
|
12 (OC:CClF3) |
−4.1 (−4.0) |
3.748 |
C
s
|
13 (H2CCH2:CClF3) |
−7.6 |
3.781 |
C
s
|
14 (HCCH:CClF3) |
−6.3 (−5.5) |
3.802 |
C
s
|
15 (OC:CCl2F2) |
−4.5 |
3.820 |
C
s
|
16 (H2CCH2:CCl2F2) |
−9.4 (−8.2) |
3.830 |
C
s
|
17 (HCCH:CCl2F2) |
−7.8 |
3.843 |
C
s
|
18 (OC:CO2) |
−5.4 |
3.240 |
C
2v
|
19a (H2CCH2:CO2) |
−9.2 |
3.266 |
C
2v
|
19b (H2CCH2:CO2) |
−8.8 |
3.252 |
C
2v
|
20a (HCCH:CO2) |
−9.8 |
3.210 |
C
2v
|
20b (HCCH:CO2) |
−6.1 |
3.330 |
C
2v
|
The σ-hole CFC complexes with ethene (7, 10, 13, 16) are more favourable than the complexes with ethyne, in agreement with the MEP values shown in Fig. 2, which indicate that ethene is slightly more π-basic than ethyne. In addition, complexes involving the CO molecule achieved the lowest binding energy values of the set, also in agreement with the MEP analysis. For π-hole complexes 18 to 20 the parallel orientation presents larger binding energy values than the perpendicular one, likely due to a major overlap between the π-systems of CO2 and ethene/ethyne molecules. Moreover, the CO2⋯ethene π-hole complexes 19a,b are more favourable than the ethyne ones (20a,b).
AIM analysis
We have used Bader's theory of “atoms in molecules” to characterize the noncovalent carbon⋯carbon bond complexes described above. The distribution of critical points (CPs) and bond paths for some representative complexes is shown in Fig. 4. For σ-hole complexes involving the CH3F moiety (6 to 8) the presence of a bond CP (red sphere) and bond path (dashed line) connecting both carbon atoms can be noted. In addition, in the case of complex 7 two symmetrically distributed bond CPs connect the π-system of ethene (both C atoms) to the carbon atom of the CH3F moiety, consequently a supramolecular ring is formed and a ring CP is created. On the other hand, for complexes involving the CO2 moiety (18, 19b and 20b) the interaction is characterized by the presence of a bond CP and bond path connecting the carbon atom of CO2 to either the carbon atom of CO or the bond critical point of the CC bond of ethene/ethyne. Moreover, in complex 19a where the CO2 and the ethene portions are disposed in parallel, the presence of two symmetrically distributed bond CPs connecting both C atoms of the ethene to the central C atom of CO2 can be noted, forming a supramolecular ring and its corresponding ring CP. The values of the Laplacian are all positive, as in common in closed shell calculations. Finally, additional AIM analyses are included in the ESI† (see Fig. S1). In these complexes the bond paths that characterize the interaction connect the carbon atoms of the electron rich molecules to the halogen atoms of the CFCs.
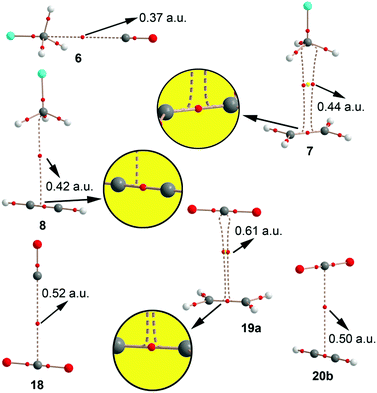 |
| Fig. 4 Distribution of critical points and bond paths in complexes 6, 7, 8, 18, 19a and 20b. Bond and ring critical points are represented by red and yellow spheres, respectively. The bond paths connecting bond critical points are also represented. The value of the density at the bond critical point (ρ × 102) is also indicated. | |
Noncovalent carbon⋯carbon bonding vs. H-bonding
This manuscript is devoted to the ability of CFCs to establish noncovalent carbon⋯carbon bonding with other atmospheric gases. It should be mentioned that the most favourable binding mode between CH3F and ethyne is the formation of a hydrogen-bonded complex by means of a HCC–H⋯FCH3 interaction. The interaction energy of the global minimum is −8.8 kJ mol−1 while the carbon⋯carbon complex 8 is −6.2 kJ mol−1. We have also analysed possible cooperativity effects between both interactions. Particularly, we have computed the binding energies of ethyne interacting with two CH3F molecules either forming two H-bonds (ΔE1, complex 21) or two noncovalent carbon⋯carbon bonds (ΔE2, complex 22), see Fig. 5. It can be observed that the H-bonding complex is approximately 5 kJ mol−1 more favourable than the noncovalent carbon⋯carbon complex. More interestingly, the H-bonded complex 21 has enhanced the ability to interact with additional CH3F molecules forming noncovalent carbon⋯carbon bonding interactions (ΔE3 = −19.8 kJ mol−1) to form complex 23. Similarly, the noncovalent carbon⋯carbon bonded complex 22 forms stronger H-bonding interactions (ΔE4 = −24.7 kJ mol−1) than ethyne alone (ΔE1 = −16.5 kJ mol−1). Therefore, strong cooperativity effects are found between both interactions in the formation of complex 23. This mutual influence between the interactions is further confirmed by the AIM analysis. The charge density at the bond CP is usually related to the interaction strength.45 It can be observed that the values of ρ(r) at the bond CPs that characterize the H-bonding and noncovalent carbon⋯carbon bonding interactions in complex 23 are greater than the corresponding values in complexes 21 and 22, thus confirming the mutual reinforcement of both interactions, in agreement with the energetic study (Fig. 5).
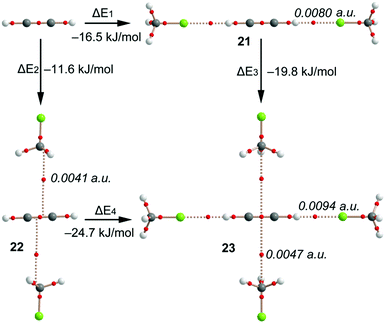 |
| Fig. 5 Distribution of critical points and bond paths in complexes 21–23, along with the binding energies. The value of the density at the bond critical point is also indicated in italics. | |
Conclusions
The results reported in this manuscript highlight the ability of CFCs and CO2 molecules to establish weak interactions (noncovalent carbon⋯carbon bonds) with CO, ethene and ethyne molecules acting as electron donors, which are present in the higher layers of the atmosphere. The results presented herein provide new insights into how these molecules interact with each other and may be important in the field of atmospheric chemistry. We have successfully used Bader's theory of “atoms in molecules” to characterize the noncovalent carbon⋯carbon bond complexes described above. Finally, favourable cooperativity effects between noncovalent carbon⋯carbon and hydrogen bonding interactions have been demonstrated energetically using the AIM theory.
Acknowledgements
A. B. and A. F. thank the DGICYT of Spain (projects CTQ2014-57393-C2-1-P and CONSOLIDER INGENIO 2010 CSD2010-00065, FEDER funds). We thank the CTI (UIB) for computational facilities.
References
- C. A. Hunter and J. K. M. Sanders, J. Am. Chem. Soc., 1990, 112, 5525 CrossRef CAS
.
- H. J. Schneider, Angew. Chem., Int. Ed., 2009, 48, 3924 CrossRef CAS PubMed
.
- C. A. Hunter and J. K. M. Sanders, J. Am. Chem. Soc., 1990, 112, 5525 CrossRef CAS
.
- W. J. Vickaryous, R. Herges and D. W. Jonhson, Angew. Chem., Int. Ed., 2004, 43, 5831 CrossRef CAS PubMed
.
- S. J. Grabowski, Chem. Rev., 2011, 111, 2597 CrossRef CAS PubMed
.
- P. Murrayrust and W. D. S. Motherwell, J. Am. Chem. Soc., 1979, 101, 4374 CrossRef CAS
.
- Y. Bai, T. R. Sosnick, L. Mayne and S. W. Englander, Science, 1995, 269, 192 CAS
.
- A. C. Legon and D. J. Millen, Acc. Chem. Res., 1987, 20, 39 CrossRef CAS
.
- P. Metrangolo, H. Neukirch, T. Pilati and G. Resnati, Acc. Chem. Res., 2005, 38, 386 CrossRef CAS PubMed
.
- G. A. Cavallo, P. Metrangolo, R. Milani, T. Pilati, A. Priimagi, G. Resnati and G. Terraneo, Chem. Rev., 2016, 116, 2478 CrossRef CAS PubMed
.
- T. Steiner, Angew. Chem., Int. Ed., 2002, 41, 48 CrossRef CAS
.
- P. Politzer, J. S. Murray and T. Clark, Phys. Chem. Chem. Phys., 2010, 12, 7748 RSC
.
- K. E. Riley, J. S. Murray, J. Franfrlík, J. Rezáč, R. J. Solá, M. C. Concha, F. M. Ramos and P. Politzer, J. Mol. Model., 2011, 17, 3309 CrossRef CAS PubMed
.
- A. Bauzá, T. J. Mooibroek and A. Frontera, Angew. Chem., Int. Ed., 2013, 52, 12317 CrossRef PubMed
.
- S. C. Nyburg and C. H. Faerman, Acta Crystallogr., Sect. B: Struct. Sci., 1985, 41, 274 CrossRef
.
- P. Politzer, K. E. Riley, F. A. Bulat and J. S. Murray, Comput. Theor. Chem., 2012, 998, 2 CrossRef CAS
.
- T. N. G. Row and R. Parthasarathy, J. Am. Chem. Soc., 1981, 103, 477 CrossRef CAS
.
- N. Ramasubbu and R. Parthasarathy, Phosphorus Sulfur Relat. Elem., 1987, 31, 221 CrossRef CAS
.
- J. S. Murray, P. Lane, T. Clark, K. E. Riley and P. Politzer, J. Mol. Model., 2012, 18, 541 CrossRef CAS PubMed
.
- H. B. Burgi, Inorg. Chem., 1973, 12, 2321 CrossRef
.
- H. B. Burgi, J. D. Dunitz and E. Shefter, J. Am. Chem. Soc., 1973, 95, 5065 CrossRef CAS
.
- H. B. Burgi, J. D. Dunitz, J. M. Lehn and G. Wipff, Tetrahedron, 1974, 30, 1563 CrossRef
.
- M. Egli and R. V. Gessner, Proc. Natl. Acad. Sci. U. S. A., 1995, 92, 180 CrossRef CAS
.
- P. Sjoberg and P. Politzer, J. Phys. Chem., 1990, 94, 3959 CrossRef CAS
.
- A. Bauzá, R. Ramis and A. Frontera, J. Phys. Chem. A, 2014, 118, 2827 CrossRef PubMed
.
- A. Bauzá, T. J. Mooibroek and A. Frontera, Chem. Commun., 2015, 51, 1491 RSC
.
- A. Bauzá, A. Frontera and T. J. Mooibroek, Cryst. Growth Des., 2016, 16, 5520 Search PubMed
.
- A. Bauzá, T. J. Mooibroek and A. Frontera, Chem. Commun., 2015, 51, 1491 RSC
.
- A. Bauzá, T. J. Mooibroek and A. Frontera, ChemPhysChem, 2016, 17, 1608 CrossRef PubMed
.
- L. M. Azofra, I. Alkorta and S. Scheiner, Theor. Chem. Acc., 2014, 133, 1586 CrossRef
.
- L. M. Azofra, I. Alkorta and S. Scheiner, Phys. Chem. Chem. Phys., 2014, 16, 18974 RSC
.
- A. Chatterjee, T. Ebina, T. Iwasaki and F. Mizukami, J. Mol. Struct., 2003, 630, 233 CrossRef CAS
.
- J. E. Lovelock, R. J. Maggs and R. A. Rasmussen, Nature, 1972, 237, 452 CrossRef CAS
.
- B. G. de Oliveira, R. C. M. Ugulino de Araújo, E. S. Leite and M. N. Ramos, Int. J. Quantum Chem., 2011, 111, 111 CrossRef
.
- K. S. Diao, F. Wang and H. J. Wang, J. Mol. Struct., 2009, 913, 195 CrossRef CAS
.
- L. Ai and J. Liu, J. Mol. Model., 2014, 20, 2179 CrossRef PubMed
.
- K. S. Diao, F. Wang and H. J. Wang, Bull. Environ. Contam. Toxicol., 2010, 84, 170 CrossRef CAS PubMed
.
-
J. G. Calvert, R. Atkinson, J. A. Kerr, S. Madronich, G. K. Moortgat and T. J. Wallington, et al., The Mechanisms of Atmospheric Oxidation of the Alkenes, Oxford University Press, New York, 2000 Search PubMed
.
-
(a) M. P. Fraser, G. R. Cass and B. R. Simoneit, Environ. Sci. Technol., 1998, 32, 2051 CrossRef CAS
;
(b) A. C. Aikin, J. R. Herman, E. J. Maier and C. J. McQuillan, J. Geophys. Res., 1982, 87, 3105 CrossRef CAS
;
(c) Y. Xiaoi, D. J. Jacob and S. Turquety, J. Geophys. Res., 2007, 112, D12305 CrossRef
.
- R. Custelcean and J. E. Jackson, Chem. Rev., 2001, 101, 1963 CrossRef CAS PubMed
.
- K. Remya and C. H. Suresh, Phys. Chem. Chem. Phys., 2015, 17, 18380 RSC
.
- R. Ahlrichs, M. Bär, M. Hacer, H. Horn and C. Kömel, Chem. Phys. Lett., 1989, 162, 165 CrossRef CAS
.
-
M. J. Frisch, G. W. Trucks, H. B. Schlegel, G. E. Scuseria, M. A. Robb, J. R. Cheeseman, G. Scalmani, V. Barone, B. Mennucci, G. A. Petersson, H. Nakatsuji, M. Caricato, X. Li, H. P. Hratchian, A. F. Izmaylov, J. Bloino, G. Zheng, J. L. Sonnenberg, M. Hada, M. Ehara, K. Toyota, R. Fukuda, J. Hasegawa, M. Ishida, T. Nakajima, Y. Honda, O. Kitao, H. Nakai, T. Vreven, J. A. Montgomery, Jr., J. E. Peralta, F. Ogliaro, M. Bearpark, J. J. Heyd, E. Brothers, K. N. Kudin, V. N. Staroverov, R. Kobayashi, J. Normand, K. Raghavachari, A. Rendell, J. C. Burant, S. S. Iyengar, J. Tomasi, M. Cossi, N. Rega, J. M. Millam, M. Klene, J. E. Knox, J. B. Cross, V. Bakken, C. Adamo, J. Jaramillo, R. Gomperts, R. E. Stratmann, O. Yazyev, A. J. Austin, R. Cammi, C. Pomelli, J. W. Ochterski, R. L. Martin, K. Morokuma, V. G. Zakrzewski, G. A. Voth, P. Salvador, J. J. Dannenberg, S. Dapprich, A. D. Daniels, Ö. Farkas, J. B. Foresman, J. V. Ortiz, J. Cioslowski and D. J. Fox, Gaussian 09, Revision B.01, Gaussian, Inc., Wallingford CT, 2009 Search PubMed
.
-
T. A. Keith, AIMAll (Version 13.05.06), TK Gristmill Software, Overland Park KS, USA, 2013 Search PubMed
.
-
J. Leszczynski, in Computational Chemistry: Reviews of Current Trends, ed. J. Leszczynski, World Scientific, Singapore, 1996, vol. 4 Search PubMed
.
Footnote |
† Electronic supplementary information (ESI) available. See DOI: 10.1039/c6cp06449c |
|
This journal is © the Owner Societies 2016 |
Click here to see how this site uses Cookies. View our privacy policy here.