DOI:
10.1039/C5CC09069E
(Communication)
Chem. Commun., 2016,
52, 1029-1031
Planar chiral desymmetrization of a two-layered cyclophane and control of dynamic helicity through the arrangement of two nonstereogenic centers†
Received
2nd November 2015
, Accepted 14th November 2015
First published on 17th November 2015
Abstract
We designed a planar chiral two-layered cyclophane, which is inherently achiral but desymmetrized by the arrangement of two nonstereogenic centers. We demonstrate the control of dynamic helicity that is generated by the helical twisting of two-layered planes in the cyclophane, where methyl and cyclohexylmethyl groups act as directing groups.
Planar chirality is generated when a plane possesses different arrangements of atoms above and below the chiral plane.1 Planar chiral molecules undergo racemization through rotation of the chiral plane.2,3 The chiroptical properties4 of molecules with enantiomers have been investigated to enable their isolation through optical resolution5 or enantioselective synthesis,6 and such robust chiral scaffolds have been used as building blocks for the construction of chiral architectures,7 functional materials,8 and asymmetric catalysts.1c,9 For most of the reported planar chiral cyclophanes, a chain-length-dependent strategy was used to make the molecule robust and configurationally stable.3,4,5a–i,6a–e Thus, configurational stability can often be achieved by making at least part of a molecule inflexible. This would appear to pose a problem for the design of a planar chiral molecule that can exhibit a range of motion.
We report a planar chiral and configurationally stable cyclophane. The cyclophane is composed of two layers that are stacked in pairs and bridged with four terephthalamide units (Fig. 1). The arrangement of two nonstereogenic centers X and Y on each amide nitrogen in the bridge generates planar chirality (1: X ≠ Y). The two-layered scaffold is inherently achiral (2: X = Y). Since neither plane can rotate, the chirality is assured to be configurationally stable. However, regarding the conformation, the two planes can be allowed to twist in a clockwise or counterclockwise manner in cases where the molecule prefers not to adopt an eclipsed form. If interconversion between two twisted forms in each enantiomer is realized, we would consider such a molecule to be conformationally dynamic.
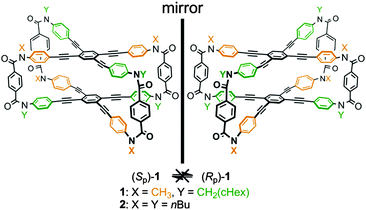 |
| Fig. 1 Chemical structures of cyclophanes 1 and 2. Planar chiral desymmetrization of 1 through the arrangement of two nonstereogenic centers X and Y (X ≠ Y). Stereochemical notation of planar chiral 1 is conformed to that reported in ref. 1c. | |
The two nonstereogenic centers X and Y, which are selected for size to be relatively small and large, can also play another role. In a helically twisted form of the two-layered cyclophane, the two nonstereogenic centers X and Y would act in concert as directing groups to determine the preferred direction of helical twisting: the smaller group should occupy a narrower space and the larger group should occupy a vacant space, which consequently would lead to a preference for a particular sense of dynamic helicity (Scheme 1). Thus, the difference in relative sizes of X and Y can control the screw-sense preference10 of dynamic helicity even without any transmission of chirality. In each configurationally stable isomer, a single pair of X and Y creates contrary preferences for (M)- or (P)-helicity. The control of dynamic helicity is a fascinating issue,11 and has been generally achieved through the transmission of some stereogenic element such as point chirality12 or planar chirality.5m,8d The generation of planar chirality and the unprecedented control of dynamic helicity through the arrangement of two nonstereogenic centers are described below.
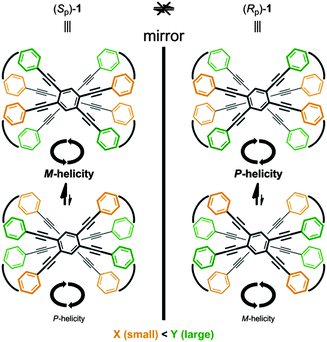 |
| Scheme 1 Control of dynamic helicity through the transmission of a difference in size between two nonstereogenic centers X (small) and Y (large) on each amide nitrogen in the bridge (terephthalamide unit), which is depicted as an arc for clarity. | |
We synthesized a two-layered cyclophane 1 [X = CH3, Y = CH2(cHex)] with a four-fold bridge. Two of the four bridges were used to form an intermediary macrocycle, which was sequentially coupled to aniline derivatives with an X or Y group to give rac-1 as a racemate through a double-ring-closing condensation (Scheme S1, ESI†).13 Both (−)-1 and (+)-1 were obtained by HPLC separation with a chiral stationary column ([α]D = −359 for the first fraction and [α]D = +365 for the second fraction). We also synthesized an achiral model 2 [X = Y = nBu] as a single species using a similar strategy (Scheme S2, ESI†).
A single-crystal X-ray analysis of 2 revealed that the two planes were forced to adopt an eclipsed form with a slight deformation of the planes to infill the cavity14 (Fig. 2). Alternatively, a conformational search for model 2′ [X = Y = Me] found a helically twisted form as the most energy-minimized structure, in which a deformation as seen in the crystal seemed to be resolved14 (Fig. 3). Such a helically twisted form led us to expect the realization of a control of helicity through arrangement of the two nonstereogenic centers X (small) and Y (large) (Scheme 1). There were no signs of an eclipsed form even when the energy window was extended up to 60 kJ mol−1.
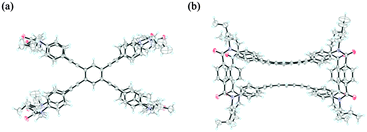 |
| Fig. 2 X-ray structure of 2 [X = Y = nBu]: (a) top view and (b) side view. Solvents are omitted for clarity. | |
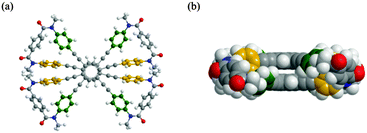 |
| Fig. 3 The most energy-minimized structure for 2′ [X = Y = Me]: (a) top view (ball and stick representation) and (b) side view (space-filling representation), obtained by a conformational search using MacroModel software (v9.9 OPLS_2005, Monte Carlo Multiple Minimum method, non-solvated, 50 000 steps). Only one enantiomeric form with (M)-helicity is depicted. | |
Next, we investigated the dynamic structure of 1 in solution. The 1H and 13C NMR spectra of rac-1, measured in chloroform-d at room temperature, showed a single set of averaged resonances (Fig. S1, ESI†), which can be explained if we consider a dynamic interconversion(s) among species involving two diastereomeric forms with (M)- or (P)-helicity. This was also supported by VT measurements which showed changes in the chemical shift of averaged resonances with temperature (Fig. S2, ESI†). Notably, a single set of average resonances emerged even when the temperature was lowered to 223 K.
The UV-vis spectrum of two-layered rac-1, measured in dichloromethane at room temperature, showed that two bands [λmax/nm (log
ε) 312 (5.16) and 360 (sh. 4.82)] characteristic of 1,2,4,5-tetrakis(phenylethynyl)benzene [316 (5.14) and 350 (4.68)]15 were mostly retained in appearance, although the intensity was markedly attenuated and the shape was broadened (Fig. 4b). A slight hypsochromic shift of the former band even with the attachment of an auxochrome and global attenuation of the spectral intensity might be attributed to the reduction of coplanarity due to twisting of the phenylethynyl groups.12b In these absorption regions, we found compositive Cotton effects in the CD spectra of (−)-1 and (+)-1, which were completely mirror-imaged (Fig. 4a). This chiroptical observation indicated that a particular sense of dynamic helicity was preferred in each enantiomer due to the arrangement of methyl and cyclohexylmethyl groups. These Cotton effects were enhanced with a decrease in temperature (Fig. S3, ESI†), which supported the contribution of a dynamic interconversion between two diastereomeric forms with (M)- or (P)-helicity.
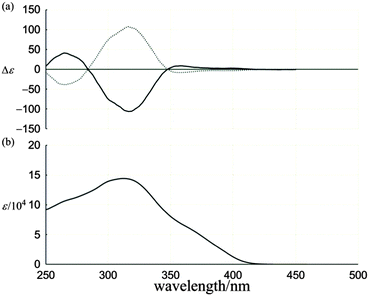 |
| Fig. 4 (a) CD spectra of (−)-1 (solid line) and (+)-1 (dashed line), and (b) UV-vis spectrum of rac-1. All spectra were measured in CH2Cl2 at room temperature. | |
In conclusion, we have demonstrated a successful control of dynamic helicity using two nonstereogenic centers based on a two-layered cyclophane, which is inherently achiral but desymmetrized by the arrangement of two nonstereogenic centers X (small) and Y (large). We used terephthalamide as a four-fold bridging unit, which induced the cyclophane to adopt helically twisted forms with (M)- or (P)-helicity, and thus dynamic helicity was generated in the configurationally stable cyclophane. Helical twisting of the two planes in the cyclophane created two diastereomeric forms due to the difference in size between X and Y, where methyl and cyclohexylmethyl groups acted as directing groups to determine a preferred sense of dynamic helicity, even though they are not stereogens.
Notes and references
-
(a) G. L. Lemière and F. C. Alderweireldt, J. Org. Chem., 1980, 45, 4175 CrossRef;
(b) B. Testa, Helv. Chim. Acta, 2013, 96, 351 CrossRef CAS;
(c) S. E. Gibson and J. D. Knight, Org. Biomol. Chem., 2003, 1, 1256 RSC.
- F. Mamiya, N. Ousaka and E. Yashima, Angew. Chem., Int. Ed., 2015, 54, 1 CrossRef CAS; H. Goto, M. Sudoh, K. Kawamoto, H. Sugimoto and S. Inoue, Chirality, 2012, 24, 867 CrossRef PubMed; K. Tomooka, T. Ezawa, H. Inoue, K. Uehara and K. Igawa, J. Am. Chem. Soc., 2011, 133, 1754 CrossRef PubMed; T. Ogoshi, R. Shiga, T. Yamagishi and Y. Nakamoto, J. Org. Chem., 2011, 76, 618 CrossRef PubMed; Y. Matsuoka, Y. Ishida, D. Sasaki and K. Saigo, Chem. – Eur. J., 2008, 14, 9215 CrossRef PubMed; T. Olszewska, M. Gdaniec and T. Połoński, J. Org. Chem., 2004, 69, 1248 CrossRef PubMed; D. H. Hochmuth and W. A. König, Tetrahedron: Asymmetry, 1999, 10, 1089 CrossRef.
- K. Takaishi, A. Muranaka, M. Kawamoto and M. Uchiyama, J. Org. Chem., 2011, 76, 7623 CrossRef CAS PubMed; E. V. D. Berge, J. Pospíšil, T. Trieu-Van, L. Collard and R. Robiette, Eur. J. Org. Chem., 2011, 6649 CrossRef.
- T. Mori and Y. Inoue, Top. Curr. Chem., 2011, 298, 99 CrossRef CAS PubMed.
-
(a) K. Akagawa, N. Nishi, I. Yoshikawa and K. Kudo, Eur. J. Org. Chem., 2015, 5055 CrossRef CAS;
(b) K. Isaac, J. Stemper, V. Servajean, P. Retailleau, J. Pastor, G. Frison, K. Kaupmees, I. Leito, J.-F. Betzer and A. Marinetti, J. Org. Chem., 2014, 79, 9639 CrossRef CAS PubMed;
(c) G. Meyer-Eppler, R. Sure, A. Schneider, G. Schnakenburg, S. Grimme and A. Lützen, J. Org. Chem., 2014, 79, 6679 CrossRef CAS PubMed;
(d) Y. Morisaki, M. Gon, T. Sasamori, N. Tokitoh and Y. Chujo, J. Am. Chem. Soc., 2014, 136, 3350 CrossRef CAS PubMed;
(e) Y. Yang, M. R. Mannion, L. N. Dawe, C. M. Kraml, R. A. Pascal, Jr. and G. J. Bodwell, J. Org. Chem., 2012, 77, 57 CrossRef CAS PubMed;
(f) Y. Ishida, E. Iwasa, Y. Matsuoka, H. Miyauchi and K. Saigo, Chem. Commun., 2009, 3401 RSC;
(g) S. Gabutti, S. Schaffner, M. Neuburger, M. Fischer, G. Schäfer and M. Mayor, Org. Biomol. Chem., 2009, 7, 3222 RSC;
(h) T. Furo, T. Mori, T. Wada and Y. Inoue, J. Am. Chem. Soc., 2005, 127, 8242 CrossRef CAS PubMed;
(i) U. Wörsdörfer, F. Vögtle, M. Nieger, M. Waletzke, S. Grimme, F. Glorius and A. Pfaltz, Synthesis, 1999, 597 CrossRef;
(j) R. Yamakado, S. Matsuoka, M. Suzuki, D. Takeuchi, H. Masu, I. Azumaya and K. Takagi, Chem. Commun., 2015, 51, 5710 RSC;
(k) J. V. Prata, A. I. Costa, G. Pescitelli and C. M. Teixeira, Tetrahedron: Asymmetry, 2014, 25, 547 CrossRef CAS;
(l) T. Ogoshi, D. Yamafuji, T. Akutsu, M. Naito and T. Yamagishi, Chem. Commun., 2013, 49, 8782 RSC;
(m) R. Yamakado, K. Mikami, K. Takagi, I. Azumaya, S. Sugimoto, S. Matsuoka, M. Suzuki, K. Katagiri, M. Uchiyama and A. Muranaka, Chem. – Eur. J., 2013, 19, 11853 CrossRef CAS PubMed;
(n) K. Tomooka, C. Iso, K. Uehara, M. Suzuki, R. Nishikawa-Shimono and K. Igawa, Angew. Chem., Int. Ed., 2012, 51, 10355 CrossRef CAS PubMed;
(o) J. Kalisiak, P. Skowronek, J. Gawroński and J. Jurczak, Chem. – Eur. J., 2006, 12, 4397 CrossRef CAS PubMed;
(p) D. Carmichael, J. Klankermayer, L. Ricard and N. Seeboth, Chem. Commun., 2004, 1144 RSC.
-
(a) T. Araki, K. Noguchi and K. Tanaka, Angew. Chem., Int. Ed., 2013, 52, 5617 CrossRef PubMed;
(b) M. Blangetti, H. Müller-Bunz and D. O'Shea, Chem. Commun., 2013, 49, 6125 RSC;
(c) N. Dendele, F. Bisaro, A.-C. Gaumont, S. Perrio and C. J. Richards, Chem. Commun., 2012, 48, 1991 RSC;
(d) K. Kanda, R. Hamanaka, K. Endo and T. Shibata, Tetrahedron, 2012, 68, 1407 CrossRef CAS;
(e) K. Kanda, T. Koike, K. Endo and T. Shibata, Chem. Commun., 2009, 1870 RSC;
(f) K. Igawa, N. Ichikawa, Y. Ano, K. Katanoda, M. Ito, T. Akiyama and K. Tomooka, J. Am. Chem. Soc., 2015, 137, 7294 CrossRef CAS PubMed;
(g) O. Riant, O. Samuel and H. B. Kagan, J. Am. Chem. Soc., 1993, 115, 5835 CrossRef CAS.
- H. Hopf, Tetrahedron, 2008, 64, 11504 CrossRef CAS; M. Cakici, Z.-G. Gu, M. Nieger, J. Bürck, L. Heinke and S. Bräse, Chem. Commun., 2015, 51, 4796 RSC; Y. Morisaki, K. Inoshita and Y. Chujo, Chem. – Eur. J., 2014, 20, 8386 CrossRef PubMed; N. L. Strutt, H. Zhang and J. F. Stoddart, Chem. Commun., 2014, 50, 7455 RSC; X. Liu, Y. Ma, W. Duan, F. He, L. Zhao and C. Song, J. Org. Chem., 2011, 76, 1953 CrossRef PubMed.
-
(a) M. Gon, Y. Morisaki and Y. Chujo, J. Mater. Chem. C, 2015, 3, 521 RSC;
(b) A. Mulas, Y. Willener, J. Carr-Smith, K. M. Joly, L. Male, C. J. Moody, S. L. Horswell, H. V. Nguyen and J. H. R. Tucker, Dalton Trans., 2015, 7268 RSC;
(c) K. Kobayakawa, M. Hasegawa, H. Sasaki, J. Endo, H. Matsuzawa, K. Sako, J. Yoshida and Y. Mazaki, Chem. – Asian J., 2014, 9, 2751 CrossRef CAS PubMed;
(d) R. Thomas, Y. Yoshida, T. Akasaka and N. Tamaoki, Chem. – Eur. J., 2012, 18, 12337 CrossRef CAS PubMed;
(e) R. Maeda, T. Wada, T. Mori, S. Kono, N. Kanomata and Y. Inoue, J. Am. Chem. Soc., 2011, 133, 10379 CrossRef CAS PubMed.
- C. T. Check, K. P. Jang, C. B. Schwamb, A. S. Wong, M. H. Wang and K. A. Scheidt, Angew. Chem., Int. Ed., 2015, 54, 4264 CrossRef CAS PubMed; C. Beemelmanns, R. Husmann, D. K. Whelligan, S. Özubuku and C. Bolm, Eur. J. Org. Chem., 2012, 3373 CrossRef; F. L. Lam, F. Y. Kwong and A. S. C. Chan, Chem. Commun., 2010, 46, 4649 RSC.
- J. Solà, M. Helliwell and J. Clayden, J. Am. Chem. Soc., 2010, 132, 4548 CrossRef CAS PubMed; Y. Inai, Y. Kurokawa and N. Kojima, J. Chem. Soc., Perkin Trans. 2, 2002, 1850 RSC.
- J. Clayden, Chem. Soc. Rev., 2009, 38, 817 RSC; J. Crassous, Chem. Soc. Rev., 2009, 38, 830 RSC; D. Pijper and B. L. Feringa, Soft Matter, 2008, 4, 1349 RSC.
-
(a) R. Katoono, K. Fujiwara and T. Suzuki, Chem. Commun., 2014, 50, 5438 RSC;
(b) R. Katoono, H. Kawai, M. Ohkita, K. Fujiwara and T. Suzuki, Chem. Commun., 2013, 49, 10352 RSC.
- During the condensation, an undesired product 3 was formed along with rac-1 in a ratio of 58
:
42 (rac-1
:
3) (Scheme S1, ESI†), and they were separated by HPLC, prior to optical resolution of rac-1.
- The shortest distance between two carbons in each plane was 3.562(5) Å. The corresponding value estimated for a helically twisted form of model 2′ was 3.642 Å.
- K. Kondo, S. Yasuda, T. Sakaguchi and M. Miya, J. Chem. Soc., Chem. Commun., 1995, 55 RSC; J. A. Marsden, J. J. Miller, L. D. Shirtcliff and M. M. Haley, J. Am. Chem. Soc., 2005, 127, 2464 CrossRef CAS PubMed.
Footnote |
† Electronic supplementary information (ESI) available: NMR and CD spectroscopic data, and experimental details of new compound preparation. CCDC 1433856. For ESI and crystallographic data in CIF or other electronic format see DOI: 10.1039/c5cc09069e |
|
This journal is © The Royal Society of Chemistry 2016 |