DOI:
10.1039/C6AY01450J
(Paper)
Anal. Methods, 2016,
8, 6602-6606
Sensing chlorinated hydrocarbons via miniaturized GaAs/AlGaAs thin-film waveguide flow cells coupled to quantum cascade lasers
Received
20th May 2016
, Accepted 2nd July 2016
First published on 5th July 2016
Abstract
Mid-infrared (MIR) sensors based on attenuated total reflection (ATR) spectroscopy provide robust, rapid and sensitive platforms for the detection of low levels of organic molecules and pollutants. Nowadays, MIR (3–15 μm) spectroscopy has evolved into a versatile sensing technique providing inherent molecular selectivity for the detection of organic and inorganic molecules. The excitation of vibrational and rotational transitions enables the qualitative and quantitative analysis of molecular constituents in solid, liquid, and vapor phases, which facilitates the application of MIR chem/bio sensors for on-site environmental analysis in scenarios such as trace pollutant monitoring or spill detection. This report presents the first integration of thin-film gallium arsenide/aluminum gallium arsenide (GaAs/AlGaAs) into a miniaturized liquid flow cell designed for continuous trace analysis of chlorinated hydrocarbons (CHCs) in water coupled to a broadly tunable quantum cascade laser (QCL), which facilitates in-field deployment of QCL-based sensing devices ensuring water quality and water safety.
In water quality monitoring, recent advancements in analytical technologies and methodologies have enabled the detection, identification, and quantification of an increasing number of relevant constituents at ever decreasing concentration levels.3,4 While a majority of these strategies – and especially chromatographic methods including GC-MS, GC-MS/MS, and HPLC5–7 – deliver the demanded qualitative and quantitative information, the associated instrumentation frequently remains rather bulky and confined to operation within a well-controlled laboratory environment. In addition, it is common that a series of sample processing steps (i.e., sampling, separation/extraction, etc.) prior to the actual analysis are required. These considerations lead to the associated cost, time, and potential uncertainties, in particular during the analysis of volatile organic compounds (VOCs) such as chlorinated hydrocarbons (CHCs). Based on the need for improved monitoring and sensing systems ideally requiring minimal sample preparation while facilitating compact, robust, rapidly responding, and sensitive devices providing inherent molecular selectivity, IR sensor technologies have emerged as a viable concept in the past two decades, and have in part already successfully been applied in water monitoring scenarios.8
Recent advancements in the miniaturization of MIR sensing techniques should facilitate their more ubiquitous presence in environmental monitoring situations.9,10 Correspondingly, MIR sensing techniques benefit in particular from the rapid development of state-of-the-art light source technologies. In particular, quantum cascade lasers (QCLs) and interband cascade lasers (ICLs) are nowadays available throughout almost the entire 3–15 μm spectral window providing broad wavelength tunability (up to 400 cm−1), compact dimensions, long life times, and sufficient optical power. The utility of laser light sources in IR spectroscopy and sensing has been demonstrated not only for transmission experiments, but also in particular for highly sensitive waveguide-based evanescent field absorption measurements.11–15 Even more recently, the first accessory for routinely utilizing thin-film waveguides in combination with QCLs has been reported providing a methodology complementary to conventional attenuated total reflection (ATR) crystals and sampling accessories coupled to Fourier transform infrared (FT-IR) spectrometers.15 Considering the miniaturization potential of QCLs and such matching sampling accessories, it is anticipated that MIR sensing techniques will become significantly more prevalent and utilized in environmental monitoring and in-field sensing scenarios.
Evanescent field sensing via ATR spectroscopy has evolved into the most frequently deployed sampling technique for probing opaque or strongly absorbing media, as the evanescent field penetrates only a few micrometers into the adjacent medium, thereby minimizing background absorptions of the matrix, e.g. in aqueous solutions. The penetration depth (dp) of the evanescent field at a certain wavelength λ may be approximated via
| 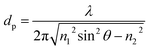 | (1) |
with the refractive index of the waveguiding structure represented as
n1, the refractive index of the adjacent medium (
i.e., usually the sample) as
n2, and the angle of incidence at the waveguide/sample interface as
θ.
16
Routinely used internal reflection elements (IREs) are usually fabricated at a thickness of several hundreds of μm up to the millimeter scale from a limited set of MIR transparent materials dominated by bulk crystals composed of zinc selenide (ZnSe), zinc sulfide (ZnS), silicon (Si), germanium (Ge) and diamond in a wide variety of lateral dimensions (a few centimeters to a few millimeters in length, width or diameter). Alternatively, optical fibers made from polycrystalline silver halides or chalcogenide glasses – just to name a few – have been replacing conventional IREs in a variety of sensing configurations.17,18 However, further miniaturization of the active sensing element, i.e. the development of ultra-thin sections of fiberoptic waveguides with a thickness on the order of magnitude of the propagating wavelength (few micrometers) was limited due to the mechanical stability of such core-only structures.
Nowadays, single- or few-mode propagating thin-film waveguides covering the entire MIR spectral range are readily available based on semiconductors and diamond materials, and are anticipated to largely replace conventional IREs and optical fibers as MIR transducers in combination with frequency-matched light sources such as QCLs and ICLs.19 Moreover, appropriate microfabrication processes and optical simulation techniques were established leading to waveguide designs providing exceptional sensitivity and utility, as shown for example via on-chip integrated Mach-Zehnder interferometers.19–21
Next to advances in thin-film waveguide technologies, appropriate surface modification of the transducing interface enables capturing, preselecting or enriching target molecules within the evanescent field for analytical signal amplification. In addition, hydrophobic coatings further reduce water background absorptions. In particular, enrichment membranes based on aliphatic polymers such as ethylene/propylene copolymer (EPCo) or polyisobutadiene, as well as sol–gel based coatings were studied as a convenient surface modification approach readily enriching VOCs and semi-volatile organic constituents (SVOCs), and enabling detection limits at low ppb levels in aqueous matrices including surface waters, aquifers and seawater. Deploying e.g., a conventional IR-ATR system combined with a silver halide ATR waveguide, limits of detection around 10 ppb for VOCs recently have been shown via the utilization of an EPco polymer enrichment membrane.22–31
In the present study, GaAs thin-film waveguides for analyzing organic constituents in water were introduced via the development of a miniaturized flow cell enabling efficient coupling of a miniaturized liquid phase sensing accessory to QCLs and QCL spectrometers. This MIR sensing platform was applied for the detection of chlorinated hydrocarbons in water at low ppm concentration levels.
Experimental
A stainless steel waveguide accessory was equipped with a GaAs/Al0.2Ga0.8As thin-film waveguide with dimensions of 5 × 10 mm. After optimization via finite-element (FEM) simulations, GaAs waveguide structures were epitaxially grown onto a Si-doped GaAs substrate at a thickness of 10 μm on top of a previously deposited 10 μm AlGaAs optical buffer layer. Advancing a previously described chip assembly,15 a miniaturized flow cell was designed and fabricated. As shown in Fig. 1, the flow cell was connected via PTFE tubing to a peristaltic pump. To avoid and sample carryover, the peristaltic pump was operated in reverse mode at a pump rate of 1 mL min−1 20 μL of a 1%wt EPCo solution (in n-hexane) was deposited onto the waveguide surface. After evaporation of the solvent, a uniform polymer membrane was obtained with a thickness of approximately 10 μm.23
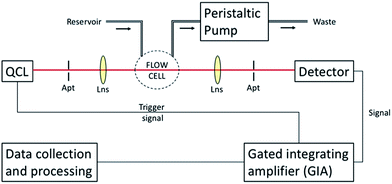 |
| Fig. 1 Schematic of the developed thin-film waveguide flow cell accessory coupled to a broadly tunable QCL. Abbreviations: Apt – pinhole aperture, Lns – convex ZnSe lens. | |
As previously described,15 the broadly tunable QCL (MIRcat, Daylight Solutions, San Diego, CA, USA) along with a mercury–cadmium–telluride (MCT) detector system was coupled to a custom-built gated-integrating amplifier (GIA) for converting laser pulses to a continuous signal. Spectra acquisition was performed via a LabView script (LabView 2014, SP1, V 14.0.1f3, National Instruments GmbH, München, Germany). A schematic of the experimental setup is shown in Fig. 1.
Chemicals
Trichloroethylene (TCE), tetrachloroethylene (perchloroethylene, PCE) and ethylene/propylene copolymer (60
:
40) (EPCo) were purchased from Sigma Aldrich (Sigma Andrich Chemie GmbH, Steinheim, Germany). n-Hexane was purchased from Merck (Merck KGaA, Darmstadt, Germany). All commercially available chemicals were used without further purification.
Results and discussion
Sensor optimization
The quality of the polymer membrane is crucial to ensure reproducible hydrocarbon enrichment. Therefore, the coating of an EPCo layer onto a GaAs thin-film waveguide surface was optimized via a series of 10, 15, 20, and 25 μL of EPCo solution applied onto the waveguide surface. After tuning the QCL emission wavelength to 1640 cm−1 (i.e. the H–O–H bending vibration of water), water was pumped across the waveguide surface and the associated IR signal was recorded for 1 h. For a membrane deposited from 10 and 15 μL of EPCo solution, a significant decrease in the IR signal (i.e., damping) was evident, which indicates that the reconstituted polymer membrane was not of sufficient thickness and homogeneity for preventing the emergence of a significant water background signal recorded via the evanescent field. For membranes established from 20 and 25 μL solutions, a significantly reduced damping of the IR signal at 1640 cm−1 resulted from water, which results from equilibration of the outermost layers of the polymer coating in agreement with previous observations.32 Hence, during subsequent studies polymer membranes were reconstituted from 20 μL EPCo solution aliquots yielding a coating thickness of approximately 10 μm.
A typical membrane preconditioning experiment is shown in Fig. 2a. During equilibration of the membrane, the IR signal at 1640 cm−1 decreases (within the first 10 min), and then increases until equilibration of the coating is achieved, and the signal remains constant.
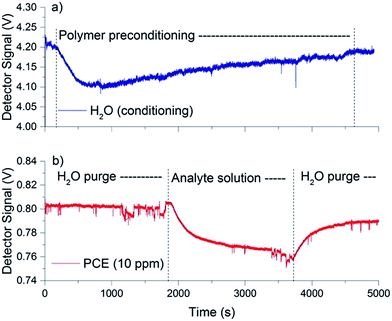 |
| Fig. 2 Typical sensor response of a 10 μm thick EPCo membrane coated onto a GaAs thin-film waveguide during a preconditioning cycle (1 h) prior to analyte application; IR signal damping at 1640 cm−1 resulting from the presence of H2O was recorded (a). Preconcentration behaviour visualized by increased damping of the IR signal at 910 cm−1 due the increasing presence of PCE within the EPCo membrane, and thus, within the penetration depth of the evanescent field. The PCE concentration in the water sample was 10 ppm. Final purging is not shown focusing on the preconcentration step (b). | |
Measurement procedure
For establishing the measurement routine, a diluted perchloroethylene (PCE) solution with a concentration of 10 ppm was prepared in deionized (DI) water. PCE was used as a model analyte for chlorinated hydrocarbons providing discriminatory absorption features within the tuning range of the applied QCL light source (i.e. @910 cm−1). Therefore, single wavelength experiments were performed tuning the QCL to emit at 910 cm−1 (i.e. 10.99 μm) operating in pulsed mode at a current of 1100 mA, which resulted in an emission power of approx. 300 mW. After aligning the thin-film waveguide such that maximal energy throughput was achieved, the flow cell was purged with DI water for 30 min for establishing a stable baseline. Subsequently, the purging solution was switched to an analyte solution containing CHCs, again 30 min until an enrichment equilibrium for PCE between the polymer membrane and PCE in solution was reached.33 After each measurement, the polymer membrane was regenerated via purging with DI water for at least 30 min until the initial IR signal was recovered. A typical measurement cycle is illustrated in Fig. 2b. The long-term stability of the polymer membrane was confirmed via numerous measurement cycles executed during the present study.
Sensor calibration
To calibrate the sensor, PCE calibration solutions were prepared at concentrations of 5, 10, 15, and 20 ppm in DI water. For each concentration, three independent measurement cycles were recorded and individually evaluated following A = −log(I/I0), as shown in Fig. 3. The detector signal obtained during water purging was used as I0, while the analyte signal at equilibrium between the solution and polymer was applied as I. Based on DIN 32645, the limit of detection (LOD) was calculated to be 5 ppm. This rather high LOD is mainly attributed to the low optical emission power of the applied QCL at 910 cm−1. It is anticipated that QCLs specifically tailored to a desired wavelength window will provide emission powers significantly exceeding the levels currently provided by generic devices. It should also be noted that in comparison to IR sensors demonstrating enrichment-based analysis of CHCs significantly larger waveguide/sample interface areas have been applied, while the current device based on thin-film waveguide technology evidently achieves respectable sensitivities using only a few square millimeters of the sensing surface given the dimensions of the GaAs chip (10 × 5 mm with an actively available surface after mounting of approx. 8 × 3 mm).
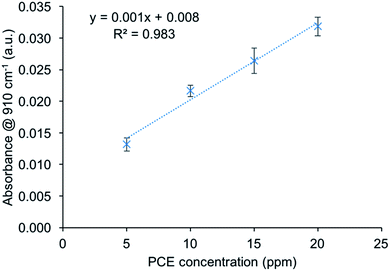 |
| Fig. 3 Single wavelength calibration for PCE at 910 cm−1 with N = 3 for each concentration using aqueous calibration samples containing 5, 10, 15 and 20 ppm of PCE. | |
Broadband detection via broadly tunable QCLs
In order to demonstrate the utility of the developed QCL-based infrared sensor concept for the simultaneous determination of several analytes, a mixture of PCE and trichloroethylene (TCE) was investigated. TCE has a characteristic absorption band at 930 cm−1, and may thus be discriminated from PCE. The flow cell was purged as described before; however, the QCL was now continuously tuned between 950 and 890 cm−1 while the detector signal was recorded. Again, QCL operation was in pulsed mode at a current of 1100 mA, which resulted in an output power between 100 and 300 mW and a laser linewidth < 1 cm−1 corresponding to the emission characteristics of the used QCL diode. Absorption spectra were again calculated via A = −log(I/I0) using the scan during water purging as the background spectrum, and the scan after signal equilibrium has been achieved as the sample scan. An exemplary spectrum comprising both analytes after a single scan from 950 and 890 cm−1 with a spectral resolution of 0.5 cm−1 is shown in Fig. 4 after smoothing with a low-pass FFT filter. The spectrum was recorded at a scan rate of 2 cm−1 s−1, whereby the spectrum was acquired within 30 s with a signal-to-noise ratio of approx. 3 dB. Faster scan rates were limited by the custom made GIA system. The applied QCL would allow scan rates up to 50 cm−1 s−1, however, at the expense of spectral resolution, which indicates that a balance between detector and light source performance has to be found. The spectral features of PCE appear well separated from TCE ensuring that both target analytes may be individually evaluated. In contrast to single wavelength experiments, baseline drifts are prevalent, which may be readily taken into account via multivariate data treatment routines utilizing spectral segments without analyte features taken into account for more robust calibration algorithms. While miniaturization of conventional FT-IR spectrometers directly affects the analytical figures-of-merit such as the signal-to-noise ratio (SNR), the intensity of the laser-based signal or the achievable scan rate in such miniaturized devices remains high due to the minute dimensions of the laser chip providing emitted beam diameters of a few tens of micrometers, thus efficiently coupling into thin-film transducers. Hence, further increasing the output power, the laser stability, and the tuning range of QCLs will directly and positively affect the achievable SNR while maintaining a small device footprint. Clearly, conventional IR-based chem/bio sensing schemes have demonstrated the fundamental feasibility of this optical sensing concept for VOC and SVOC analysis. Yet, QCL-based sensors in combination with highly efficient thin-film waveguides and appropriately miniaturized sampling accessories such as the flow cell shown herein will be instrumental in enabling performance characteristics suitable for analytical measurement scenarios demanding for particularly rapid and sensitive in situ measurements under in-field conditions.
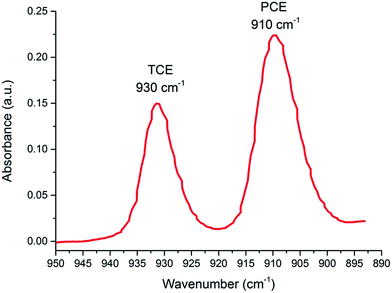 |
| Fig. 4 IR single-scan spectrum of TCE and PCE simultaneously preconcentrated via an EPCo membrane coated onto a GaAs thin-film waveguide from an aqueous sample matrix using a broadly tunable QCL covering the wavelength window of 950–890 cm−1. The spectrum was smoothed via a low-pass FFT filter. | |
Conclusions
The first prototype of an IR sensing system comprising broadly tunable QCLs or QCL spectrometers in combination with thin-film GaAs/AlGaAs waveguides serving as evanescent field transducers mounted within a miniaturized flow cell for the detection of chlorinated hydrocarbons PCE has been shown. An EPCo polymer membrane coated onto the GaAs transducer serving as a hydrophobic enrichment membrane facilitated low ppm detection limits using a remarkably small (i.e. a few square millimeters) sampling interface. The presented sensor system is modularly designed facilitating further system miniaturization/integration and adaptability to a wide range of analytes (e.g. via tailoring or selecting appropriate enrichment membranes) and sensing scenarios. Hence, next to the analysis of trace pollutants and environmental sensing it is anticipated that a variety of point-of-care scenarios may likewise benefit from this sensing platform.
Acknowledgements
This study has in part been funded by the European Union's Seventh Framework Programme managed by the REA (Research Executive Agency) http://www.ec.europa.eu/rea (FP7/2007-2013) under Grant Agreement No. 314018 FP7-SME-2012-SME (MYCOSPEC). Furthermore, the authors gratefully acknowledge partial support by the Kompetenznetz Funktionelle Nanostrukturen Baden Wuerttemberg, Germany, and by the Center for Integrated Quantum Science and Technology (IQST), University of Ulm and University of Stuttgart, Germany. Furthermore, the machine shop at University of Ulm is thanked for their valuable collaboration during prototype development. Last but not least, Daylight Solutions, Inc. (San Diego, CA, U.S.A.) and MG Optical Solutions are thanked for years of fruitful collaboration on advancing the QCL-based sensor technology.
References
- B. Mizaikoff, Anal. Chem., 2003, 75, 258–267 CrossRef.
- B. Mizaikoff, Chem. Soc. Rev., 2013, 42, 8683–8699 RSC.
- A. Ballesteros-Gómez and S. Rubio, Anal. Chem., 2011, 83, 4579–4613 CrossRef PubMed.
- N. S. Chary and A. R. Fernandez-Alba, Trends Anal. Chem., 2012, 32, 60–75 CrossRef CAS.
- R. A. Ketola, V. T. Virkki, M. Ojala, V. Komppa and T. Kotiaho, Talanta, 1997, 44, 373–382 CrossRef CAS PubMed.
- A. D. Nikolaou, S. K. Golfinopoulos, M. N. Kostopoulou, G. A. Kolokythas and T. D. Lekkas, Water Res., 2002, 36, 2883–2890 CrossRef CAS PubMed.
- S. D. Richardson and T. A. Ternes, Anal. Chem., 2011, 83, 4614–4648 CrossRef CAS PubMed.
- B. Pejcic, L. Boyd, M. Myers, A. Ross, Y. Raichlin, A. Katzir, R. Lu and B. Mizaikoff, Org. Geochem., 2013, 55, 63–71 CrossRef CAS.
- T. Schädle, B. Pejcic, M. Myers and B. Mizaikoff, Anal. Chem., 2014, 86, 9512–9517 CrossRef PubMed.
- R. Stach, B. Pejcic, E. Crooke, M. Myers and B. Mizaikoff, Anal. Chem., 2015, 87, 12306–12312 CrossRef CAS PubMed.
- M. R. Alcaráz, A. Schwaighofer, C. Kristament, G. Ramer, M. Brandstetter, H. Goicoechea and B. Lendl, Anal. Chem., 2015, 87, 6980–6987 CrossRef PubMed.
-
T. Vahlsing, H. Moser, M. Grafen, K. Nalpantidis, M. Brandstetter, H. M. Heise, B. Lendl, S. Leonhardt, D. Ihrig and A. Ostendorf, Eur. Conf. Biomed. Opt., 2015, vol. 9537, p. 953713 Search PubMed.
- B. Mizaikoff and B. Lendl, Analyst, 2014, 139, 2038 RSC.
- B. Mizaikoff, Chem. Soc. Rev., 2013, 42, 8683 RSC.
- M. Sieger, J. Haas, M. Jetter, P. Michler, M. Godejohann and B. Mizaikoff, Anal. Chem., 2016, 88, 2558–2562 CrossRef CAS PubMed.
-
N. J. Harrick, Internal Reflection Spectroscopy, John Wiley & Sons Inc, New York, 1967 Search PubMed.
- S. Simhony, E. M. Kosower and A. Katzir, Appl. Phys. Lett., 1986, 49, 253–254 CrossRef CAS.
- I. D. Aggarwal and J. S. Sanghera, J. Optoelectron. Adv. Mater., 2002, 4, 665–678 CAS.
- X. Wang, S.-S. Kim, R. Rossbach, M. Jetter, P. Michler and B. Mizaikoff, Analyst, 2012, 137, 2322–2327 RSC.
- M. Sieger and B. Mizaikoff, Photonics Res., 2016, 4, 106–110 CrossRef.
- M. Sieger, F. Balluff, X. Wang, S. Kim, L. Leidner, G. Gauglitz and B. Mizaikoff, Anal. Chem., 2013, 85, 3050–3052 CrossRef CAS PubMed.
- R. Lu, B. Mizaikoff, W. W. Li, C. Qian, A. Katzir, Y. Raichlin, G. P. Sheng and H. Q. Yu, Sci. Rep., 2013, 3, 2525 Search PubMed.
- R. Lu, W.-W. Li, B. Mizaikoff, A. Katzir, Y. Raichlin, G.-P. Sheng and H.-Q. Yu, Nat. Protoc., 2016, 11, 377–386 CrossRef CAS PubMed.
- R. Lu, G. Sheng, W. Li, H. Yu, Y. Raichlin, A. Katzir and B. Mizaikoff, Angew. Chem., Int. Ed., 2013, 52, 2265–2268 CrossRef CAS PubMed.
- B. Pejcic, M. Myers and A. Ross, Sensors, 2009, 9, 6232–6253 CrossRef CAS PubMed.
- T. Schädle, B. Pejcic and B. Mizaikoff, Anal. Methods, 2016, 8, 756–762 RSC.
- Y. Luzinova, B. Zdyrko, I. Luzinov and B. Mizaikoff, Anal. Chem., 2012, 84, 1274–1280 CrossRef CAS PubMed.
- F. Rauh, M. Schwenk, B. Pejcic, M. Myers, K. B. Ho, L. Stalker and B. Mizaikoff, Talanta, 2014, 130, 527–535 CrossRef CAS PubMed.
- M. Karlowatz, M. Kraft and B. Mizaikoff, Anal. Chem., 2004, 76, 2643–2648 CrossRef CAS PubMed.
- M. Janotta, M. Karlowatz, F. Vogt and B. Mizaikoff, Anal. Chim. Acta, 2003, 496, 339–348 CrossRef CAS.
- W. Lin and Z. Li, Anal. Chem., 2010, 82, 505–515 CrossRef CAS PubMed.
- P. Hahn, M. Tacke, M. Jakusch, B. Mizaikoff, O. Spector and A. Katzir, Appl. Spectrosc., 2001, 55, 39–43 CrossRef CAS.
- C. Phillips, M. Jakusch, H. Steiner, B. Mizaikoff and A. G. Fedorov, Anal. Chem., 2003, 75, 1106–1115 CrossRef CAS PubMed.
|
This journal is © The Royal Society of Chemistry 2016 |