DOI:
10.1039/C5AN01996F
(Paper)
Analyst, 2016,
141, 910-917
Temperature-responsive molecular recognition chromatography using phenylalanine and tryptophan derived polymer modified silica beads†
Received
28th September 2015
, Accepted 24th November 2015
First published on 25th November 2015
Abstract
Temperature-responsive polymers incorporating molecular-recognition sites were developed as stationary phases for high-performance liquid chromatography (HPLC). The grafted stationary phases consisted of functional copolymers composed of N-isopropylacrylamide (NIPAAm) and N-acryloyl aromatic amino acid methyl esters, i.e., phenylalanine and tryptophan methyl esters (Phe-OMe and Trp-OMe). Three novel temperature-responsive polymers, P(NIPAAm-co-Phe-OMe5), P(NIPAAm-co-Phe-OMe10), and P(NIPAAm-co-Trp-OMe5), were synthesized. These copolymers exhibited a reversible hydrophilic/hydrophobic phase transition at their lower critical solution temperatures (LCSTs). The polymers were grafted onto aminopropyl silica using an activated ester-amine coupling method, and were packed into a stainless steel column, which was connected to an HPLC system. Temperature-responsive chromatography was conducted using water as the sole mobile phase. More hydrophobic analytes were retained longer, and the retention times of aromatic steroids and aromatic amino acids were dramatically increased. This indicated that π–π interactions occurred between the phenyl or indole moieties of phenylalanine or tryptophan, respectively, and the aromatic compounds. Furthermore, the retention times of compounds with hydrogen bond acceptors were higher with P(NIPAAm-co-Trp-OMe5), which contained indole as a hydrogen bond donor, than with P(NIPAAm-co-Phe-OMe5). This indicated that hydrogen bonding occurred between the stationary phase and the analytes. These results indicate that hydrophobic, π–π, and hydrogen bonding interactions all affected the separation mode of the temperature-responsive chromatography, and led to selective separation with molecular recognition. Both temperature-response and molecular recognition characteristics are present in the proposed separation system that utilizes a temperature-responsive polymer bearing aromatic amino acid derivatives.
Introduction
High-performance liquid chromatography (HPLC) is widely used for chemical analysis and related applications because of its ability to separate and quantify analytes.1 Reversed-phase liquid chromatography (RPLC), which uses a hydrophobic stationary phase, e.g., octadecylsilyl-modified silica, is most commonly used. The retention and selectivity in RPLC systems are controlled by varying the polarity of the mobile phase using organic solvents.
Stimuli-responsive polymers that respond to heat,2 light,3 pH,4 and ions5 have found interesting applications in chemical and biomedical fields.6 Poly(N-isopropylacrylamide) (PNIPAAm) is a widely known thermo-responsive polymer and exhibits thermally reversible solubility changes in aqueous solutions in response to temperature changes across its lower critical solution temperature (LCST, 32 °C).7 The polymer chains of PNIPAAm are hydrophilic and hydrated in water below the LCST, whereas above, they are hydrophobic and dehydrated. By exploiting this behavior, we developed temperature-responsive chromatography systems based on PNIPAAm-modified stationary phases.8 PNIPAAm-grafted silica bead surfaces exhibit thermo-responsive switchable hydrophobicity. Whereas conventional chromatography uses reverse-phase columns, in which the partitioning is controlled by the mobile phase composition, in our temperature-responsive chromatography system, the partitioning is controlled by external temperature changes and water alone is used as the mobile phase.9–11
The LCST of PNIPAAm can be adjusted to a specific temperature through its copolymer composition.12,13 The addition of butyl methacrylate (BMA) as a hydrophobic monomer lowers the LCST. Hydrophobic interactions between the solutes and copolymer-grafted surfaces of the stationary phase were increased by the inclusion of BMA, which resulted in longer retention times.14 Upon introducing a naphthylalanine derivative to obtain P(NIPAAm-co-Nap-OMe3), a π–π interaction separation mode was introduced, and the retention times of aromatic steroids were drastically increased compared with those observed using homo-PNIPAAm.15 The affinity for hydrophobic amino acids was increased upon introducing a proline derivative.16 It is possible to impart new separation modes to temperature-responsive chromatography systems by introducing functional monomers into PNIPAAm.9–11
In this study, we focused on aromatic amino acids to introduce a new separation mode featuring molecular recognition. Aromatic amino acids have unique and important properties. Phenylalanine (Phe) and tryptophan (Trp) are generally hydrophobic, but compared with simpler hydrophobic residues, such as leucine or valine, the aromatic amino acids have additional capabilities, such as π–π interactions. Trp, in particular, can form hydrogen bonds, which is an important feature. These types of non-covalent binding interactions contribute to protein secondary structures and to diverse drug–receptor interactions. A pharmacophore model is a well-known method used in drug discovery, to describe molecular recognition of a ligand by a biological macromolecule. Typical pharmacophore features include hydrophobic centroids, aromatic rings, hydrogen bond acceptors or donors, cations, and anions. In previous studies, we successfully demonstrated that hydrophobic14 and electrostatic interactions17 could be modulated by temperature changes in temperature-responsive chromatography. Therefore, the addition of further interactions, such as π–π interactions and hydrogen bonding, to the separation mode should be effective for complex molecular recognition.
Herein, we designed temperature-responsive PNIPAAm-based copolymers with N-acryloyl aromatic amino acid methyl esters, i.e., phenylalanine and tryptophan methyl esters (Phe-OMe and Trp-OMe). Three novel temperature-responsive polymers, P(NIPAAm-co-Phe-OMe5), P(NIPAAm-co-Phe-OMe10), and P(NIPAAm-co-Trp-OMe5) (where the number corresponds to the feed ratio) were synthesized and grafted onto aminopropyl silica. Temperature-responsive chromatography was conducted using only water as the mobile phase. Upon increasing the temperature, the retention time of the analytes increased. Furthermore, compounds with aromatic and/or hydrogen bond acceptor moieties were strongly retained. We evaluated the effects of the phenyl and indole moieties of phenylalanine and tryptophan, respectively, on π–π and hydrogen bonding interactions.
Experimental
Materials and chemicals
N-Isopropylacrylamide (NIPAAm), kindly provided by KJ Chemicals Co., Ltd. (Tokyo, Japan), was purified by recrystallization from n-hexane, and then dried at 25 °C under vacuum. N-Acryloyl-L-phenylalanine methyl ester and N-acryloyl-L-tryptophan methyl ester were synthesized as previously reported.18,19 2,2′-Azobisisobutyronitrile (AIBN) was purchased from Wako Pure Chemical Industries (Osaka, Japan). 3-Mercaptopropionic acid (MPA) and N,N′-dicyclohexylcarbodiimide (DCC) were purchased from Kanto Chemicals (Tokyo, Japan). N-Hydroxysuccinimide (NHS) was purchased from Merck Japan (Tokyo, Japan). Aminopropyl silica (average diameter = 3 μm) was purchased from Nishio Kogyo (Tokyo, Japan). The analytes uracil, hydrocortisone, prednisolone, dexamethasone, hydrocortisone acetate, testosterone, naphthalene, 1,5-dinitronaphthalene, fluocinonide, estriol, β-estradiol, and phenylthiohydantoin (PTH)-amino acids (Gly, Ala, Pro, Met, Leu, Thr, Phe, Tyr, and Trp) were purchased from Wako Pure Chemical Industries (Osaka, Japan). Water was passed through a Milli-Q purification system (Millipore, Bedford, MA, USA). All other reagents and solvents were of analytical grade.
Synthesis of temperature-responsive polymers containing amino acid derivatives
P(NIPAAm-co-Phe-OMe5) was prepared using radical polymerization. NIPAAm (18.0 g, 159 mmol, 95 equiv.) and N-acryloyl-L-phenylalanine methyl ester (1.96 g, 8.40 mmol, 5 equiv.) were dissolved in N,N-dimethylformamide (DMF, 45 mL). AIBN (0.116 g, 0.706 mmol, 0.4 equiv.) and MPA (0.496 g, 4.13 mmol, 2.5 equiv.), which act as the radical initiator and chain transfer agent, respectively, were added to the solution. The reaction mixture was degassed using freeze–pump–thaw cycles, and then heated to 70 °C. After reacting for 5 h, the solution was poured into diethyl ether to precipitate the polymer. The crude product was further purified by repeated precipitation from an acetone solution into diethyl ether, and then dried to yield a white solid.
P(NIPAAm-co-Phe-OMe10) and P(NIPAAm-co-Trp-OMe5) were synthesized using a similar procedure, changing the molar ratios and amino acid-derived monomer. Their reaction compositions are as follows: P(NIPAAm-co-Phe-OMe10) = NIPAAm (12.2 g, 108 mmol, 90 equiv.), N-acryloyl-L-phenylalanine methyl ester (2.80 g, 12.0 mmol, 10 equiv.), DMF (50 mL), AIBN (0.087 g, 0.530 mmol, 0.4 equiv.), and MPA (0.357 g, 2.97 mmol, 2.5 equiv.); and P(NIPAAm-co-Trp-OMe5) = NIPAAm (22.2 g, 196 mmol, 95 equiv.), N-acryloyl-L-tryptophan methyl ester (2.80 g, 10.3 mmol, 5 equiv.), DMF (80 mL), AIBN (0.145 g, 0.883 mmol, 0.4 equiv.), and MPA (0.614 g, 5.11 mmol, 2.5 equiv.).
Characterization of the temperature-responsive polymers
The 1H NMR spectra were recorded at 500 MHz on a Varian INOVA-500 spectrometer. The molecular weights of the polymers were determined through gel permeation chromatography (GPC, GPC-8020 system: column, TSK-GEL; mobile phase, DMF containing 10 mM LiCl; TOSOH, Tokyo, Japan) using poly(ethylene oxide) standards for calibration. The LCSTs of the polymers were determined by measuring their optical transmittance in aqueous solution (0.5 w/v%). The optical transmittance was measured at various temperatures at 500 nm using a UV-Vis spectrophotometer (V-630, JASCO, Tokyo, Japan). The temperature was controlled using an ETC-717 controller (JASCO) and a PT-31 Peltier system (Krüss, Hamburg, Germany) at a heating rate of 0.1 °C min−1. The LCSTs were determined as the temperature where the polymer solutions had 50% optical transmittance.
Modification of aminopropyl silica with temperature-responsive polymers
P(NIPAAm-co-Phe-OMe5) (6.5 g) was dissolved in 50 mL of ethyl acetate. DCC (0.51 g, 2.5 equiv.) and NHS (0.29 g, 2.5 equiv.) were then added to the solution. The reaction mixture was stirred at 4 °C for 2 h and then at 25 °C for an additional 24 h. The precipitated dicyclohexylurea was removed by filtration, and the filtrate was poured into diethyl ether to precipitate the polymer. The resulting residue with activated terminal carboxylic acid (1.5 g) was dissolved in 50 mL of 1,4-dioxane. To this was added 3.0 g of aminopropyl silica. The reaction mixture was shaken at 25 °C for 24 h. This process was repeated three times. The resulting polymer-modified silica was washed with 500 mL of water, then 100 mL of methanol, and dried under vacuum at ambient temperature. The P(NIPAAm-co-Phe-OMe10) and P(NIPAAm-co-Trp-OMe5)-modified silica beads were prepared using similar procedures.
Temperature-responsive chromatography
The polymer-grafted silica beads were packed into a stainless-steel column (100 mm length × 2.1 mm i.d.). The column was then connected to an HPLC system. HPLC analysis was performed using a Hitachi D-7000 controller equipped with an L-7100 pump and L-74000 UV detector (wavelength = 254 nm). The temperature-responsive column was eluted with Milli-Q water at a flow rate of 0.2 mL min−1. Standard solutions of all of the analytes were prepared at concentrations of 0.1 mg mL−1. The retention factors of the analytes were calculated using the following equation: retention factor = (tR − t0)/t0, where t0 and tR are the retention times of uracil and the target analyte, respectively. The selectivity of the analytes was calculated using the following equation: selectivity (α) = (tR2 − t0)/(tR1 − t0).
Results and discussion
Temperature response of PNIPAAm copolymers
L-Phenylalanine and L-tryptophan were converted into their methyl esters using SOCl2 in MeOH,20 and their monomers were synthesized through a reaction with acryloyl chloride in the presence of triethylamine.18,19 P(NIPAAm-co-Phe-OMe5), P(NIPAAm-co-Phe-OMe10), and P(NIPAAm-co-Trp-OMe5) (Fig. 1) were synthesized from NIPAAm and their corresponding amino acid-derived acrylamides through radical polymerization using MPA. Although the number in our polymer notation corresponds to the feed content of the amino acid-derived acrylamides in the polymerization reaction, the actual introduction rates were determined by 1H NMR analysis (see the ESI†). The NIPAAm/amino acid-derived acrylamide ratios of the polymers were estimated using the integrated signals derived from the NIPAAm methine (1H, 3.9 ppm) proton and the aromatic ring of the amino acid-derived acrylamides (5H, 7.0–7.3 ppm). The monomer composition in the polymers was found to be similar to their initial feed composition. The polydispersity index (PDI) of the polymers was determined using GPC. The temperature dependence of the optical transmittance of homo-PNIPAAm, P(NIPAAm-co-Phe-OMe5), P(NIPAAm-co-Phe-OMe10), and P(NIPAAm-co-Trp-OMe5) is shown in Fig. 2. The LCSTs of the PNIPAAm-based copolymers were lower than that of homo-PNIPAAm. Therefore, the introduction of Phe-OMe or Trp-OMe increased the hydrophobicity of the polymer chain. Table 1 summarizes the polymer characterization data.
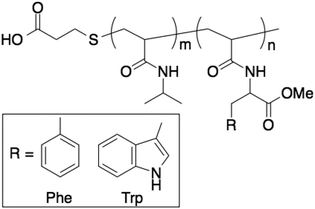 |
| Fig. 1 Structures of PNIPAAm-based temperature-responsive polymers containing aromatic amino acid derivatives. | |
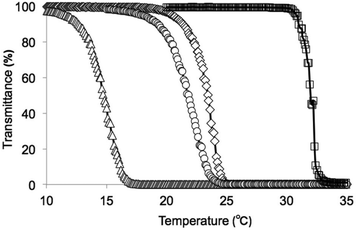 |
| Fig. 2 Phase transition profiles of (□) PNIPAAm, (◊) P(NIPAAm-co-Phe-OMe5), (△) P(NIPAAm-co-Phe-OMe10), and (○) P(NIPAAm-co-Trp-OMe5) in aqueous solution (0.5 w/v%). | |
Table 1 Physicochemical properties of PNIPAAm-based temperature-responsive polymers containing aromatic amino acid derivatives
Polymer sample code |
Composition ratios amino acid deriv.a |
M
|
M
w/Mn c |
LCST (°C) |
Determined by 1H NMR analyses.
Number-averaged molecular weight determined by end group titration.
Determined by GPC.
|
P(NIPAm-co-Phe-OMe5) |
4.9% |
6500 |
2.1 |
23.3 |
P(NIPAm-co-Phe-OMe10) |
9.9% |
6400 |
2.1 |
14.8 |
P(NIPAm-co-Trp-OMe5) |
5.3% |
5900 |
1.8 |
21.7 |
Temperature-responsive chromatography for the separation of sex steroids
Temperature-responsive stationary phases were prepared by grafting the three polymers onto aminopropyl silica through the activation of the terminal carboxyl groups with NHS (Fig. S1†), after which they were packed into stainless steel HPLC columns. We evaluated the effects of column temperature on the elution behavior of sex steroids on P(NIPAAm-co-BMA5), P(NIPAAm-co-Phe-OMe5), P(NIPAAm-co-Phe-OMe10), and P(NIPAAm-co-Trp-OMe5) columns. The effect of aromatic moieties on the elution behavior of sex steroids was evaluated by comparison with P(NIPAAm-co-BMA5) having the hydrophobic group of an aliphatic moiety. Male and female steroid hormones are primarily secreted by the testes or ovaries, and contribute to the development of secondary sexual characteristics and reproductive functions. Among the sex steroid hormones, we focused on the structures of testosterone, estriol, and β-estradiol (the structures and log
P values are shown in Fig. S2†). Estriol has an aromatic ring, and its log
P value is less than that of testosterone, which has no aromatic ring. The log
P value, which is the logarithm of a compound's partition coefficient between n-octanol and water, is a well-established measure of its hydrophobicity. β-Estradiol has two hydroxyl groups, which is fewer than that found in estriol, and it has the largest log
P value among these steroid hormones. Fig. 3 shows the chromatograms of the sex steroid hormones on the P(NIPAAm-co-BMA5), P(NIPAAm-co-Phe-OMe5), P(NIPAAm-co-Phe-OMe10), and P(NIPAAm-co-Trp-OMe5) columns upon changing the column temperature from 5 to 40 °C. Recently, many commercial columns that can use water alone as the mobile phase have become available; however, these columns cannot modulate the retention and separation selectivity under aqueous conditions without the addition of organic solvent and/or salt. In contrast, in a temperature-responsive chromatographic system, the surface properties and function of the HPLC stationary phase are controlled by external temperature changes without any changes in the mobile-phase composition.
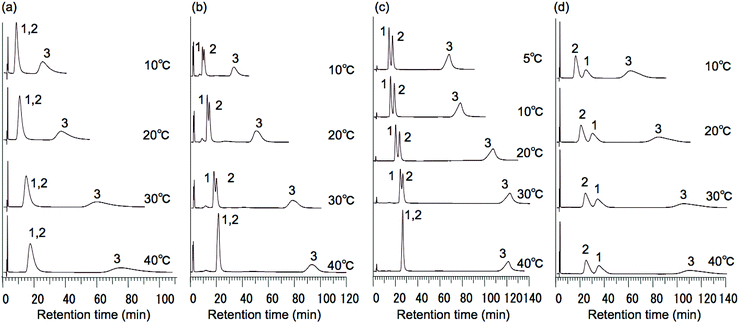 |
| Fig. 3 Temperature-dependent elution profiles of the sex steroids on (a) P(NIPAAm-co-BMA5), (b) P(NIPAAm-co-Phe-OMe5), (c) P(NIPAAm-co-Phe-OMe10), and (d) P(NIPAAm-co-Trp-OMe5) HPLC columns. Peaks (1) testosterone, (2) estriol, and (3) β-estradiol. | |
Fig. 4 shows the temperature dependence of the retention factors on each column (a magnified figure is shown in Fig. S3†). The retention factor of β-estradiol was highest on all of the columns. β-Estradiol was retained by hydrophobic interactions with the polymer chains, and its retention factor increased with increasing temperature. The results indicate that these systems could alter their partitioning using only water as the mobile phase through temperature-induced changes to the surface hydrophobicity of the stationary phase. These elution behaviors were similar to those observed in our previous work.8 Because the retention factors of testosterone and estriol on P(NIPAAm-co-BMA5) were similar, they could not be separated at any temperature. However, differences in the retention factors of testosterone and estriol were observed on P(NIPAAm-co-Phe-OMe5), P(NIPAAm-co-Phe-OMe10), and P(NIPAAm-co-Trp-OMe5).
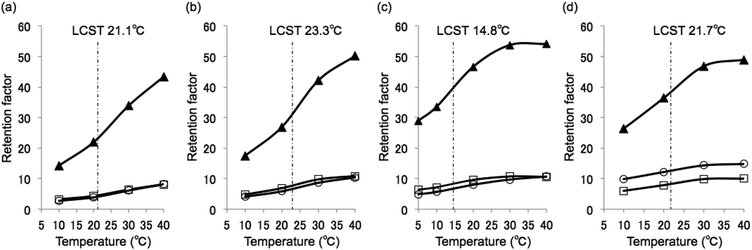 |
| Fig. 4 Temperature dependence of the retention factors of the sex steroids on (a) P(NIPAAm-co-BMA5), (b) P(NIPAAm-co-Phe-OMe5), (c) P(NIPAAm-co-Phe-OMe10), and (d) P(NIPAAm-co-Trp-OMe5) HPLC columns: (○) testosterone, (□) estriol, and (▲) β-estradiol. | |
The retention factor of estriol was larger than that of testosterone on P(NIPAAm-co-Phe-OMe5) and P(NIPAAm-co-Phe-OMe10), although the log
P value of estriol is less than that of testosterone. In addition, the α value of testosterone and estriol on P(NIPAAm-co-Phe-OMe5) and P(NIPAAm-co-Phe-OMe10) increased with decreasing temperature (Fig. S4†). These results indicate that π–π interactions between the aromatic ring of estriol and the phenyl moiety of phenylalanine occurred in addition to hydrophobic interactions. While the π–π interactions were effective at lower temperatures when the phenyl moieties were exposed and the polymer chains were hydrated, they were weakened when the phenyl moieties were hidden in the shrunken dehydrated polymer chains at higher temperatures.
The retention factor of testosterone was larger than that of estriol on P(NIPAAm-co-Trp-OMe5). Stronger non-π–π interactions were suspected between P(NIPAAm-co-Trp-OMe5) and testosterone than with estriol.
Evaluation of π–π interactions between the analytes and polymers
Naphthalene and 1,5-dinitronaphthalene were used as analytes to evaluate whether π–π interactions occur between the aromatic amino acid-derived polymers and aromatic solutes. The π-electron density of the aromatic ring of 1,5-dinitronaphthalene is low because of the strongly electron-withdrawing nitro groups, and its π–π interactions with aromatic rings are much stronger than those of naphthalene. Naphthalene (log
P = 3.3) is more hydrophobic than 1,5-dinitronaphthalene (log
P = 2.6). Therefore, in our previous work, the retention time of naphthalene was longer than that of 1,5-dinitronaphthalene on a homo-PNIPAAm column, and the elution order was similar to a conventional ODS column.15 Here, the retention times of naphthalene were shorter than those of 1,5-dinitronaphthalene on P(NIPAAm-co-Phe-OMe5), P(NIPAAm-co-Phe-OMe10), and P(NIPAAm-co-Trp-OMe5) columns; that is, the opposite elution order was observed. These results indicate that π–π interactions with the phenyl and indole moieties of phenylalanine and tryptophan, respectively, dominate the separation mode. Fig. 5(a) shows the effects of temperature on the selectivity (α) of naphthalene and 1,5-dinitronaphthalene on P(NIPAAm-co-BMA5), P(NIPAAm-co-Phe-OMe5), P(NIPAAm-co-Phe-OMe10), and P(NIPAAm-co-Trp-OMe5) columns. The α value on the P(NIPAAm-co-Phe-OMe10) column was larger than that on the P(NIPAAm-co-Phe-OMe5) column, suggesting that the π–π interactions between the stationary phase and the solute became stronger upon increasing the Phe-OMe content. As shown in Fig. 5(b), selectivity was increased at lower temperatures on the columns containing aromatic amino acids compared with the P(NIPAAm-co-BMA5) column. As shown in Fig. 5(a), the α value on the P(NIPAAm-co-Trp-OMe5) column was larger, and the peak shape of 1,5-dinitronaphthalene in the chromatogram was broader, than on the P(NIPAAm-co-Phe-OMe5) column (Fig. S5†). These results indicate that the indole moiety of tryptophan strongly interacted with 1,5-dinitronaphthalene. Notably, the α value on the P(NIPAAm-co-Trp-OMe5) column decreased with increasing temperature, which indicated that the interaction between the tryptophan indole moiety and 1,5-dinitronaphthalene weakened with increasing temperature. In general, hydrogen bonds are weakened when the temperature increases. Indole acts as a hydrogen bond donor and nitro groups act as hydrogen bond acceptors.21 Therefore, a hydrogen bond between the nitro moieties of 1,5-dinitronaphthalene and NH in the indoles on P(NIPAAm-co-Trp-OMe5) might affect the characteristic retention of 1,5-dinitronaphthalene. The results indicate that the P(NIPAAm-co-Trp-OMe5) column has different selectivities than the P(NIPAAm-co-Phe-OMe5) column.
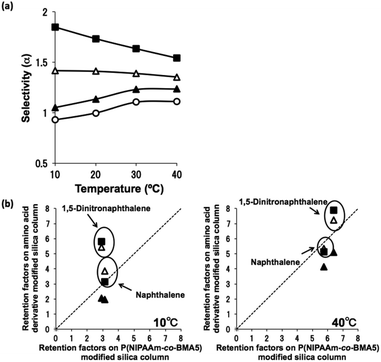 |
| Fig. 5 (a) Relationships between temperature and the selectivity for naphthalene and 1,5-dinitronaphthalene. (b) Effects of the introduction of an amino acid derivative on the selectivity at 10 and 40 °C. (○) P(NIPAAm-co-BMA5), (▲) P(NIPAAm-co-Phe-OMe5), (△) P(NIPAAm-co-Phe-OMe10), (■) P(NIPAAm-co-Trp-OMe5). | |
Evaluation of hydrogen bonding between the analytes and polymers
To evaluate the possibility of hydrogen bonding, we compared the retention behavior of fluocinonide, which has two fluorine atoms that act as hydrogen bond acceptors,22 and testosterone on each column. Fig. 6 shows the chromatograms of testosterone and fluocinonide on P(NIPAAm-co-BMA5),14 P(NIPAAm-co-Phe-OMe5), and P(NIPAAm-co-Trp-OMe5) columns upon changing the column temperature from 5 to 40 °C. Fig. 7(a) shows the temperature dependence of the retention factors of fluocinonide on P(NIPAAm-co-BMA5), P(NIPAAm-co-Phe-OMe5), and P(NIPAAm-co-Trp-OMe5). The retention factor of fluocinonide on P(NIPAAm-co-Trp-OMe5) was higher than that on the other columns at low temperatures. However, it did not significantly increase with increasing temperature when compared with the other columns. Although the retention factor on P(NIPAAm-co-BMA5) was lower than on P(NIPAAm-co-Trp-OMe5) at low temperatures, it was higher at 40 °C. The hydrophobic interactions between fluocinonide and P(NIPAAm-co-BMA5) or P(NIPAAm-co-Phe-OMe5) could simply have increased with increasing temperature. However, although the hydrophobic interaction between fluocinonide and P(NIPAAm-co-Trp-OMe5) increased, the hydrogen bonding between the two fluorine atoms in fluocinonide and the NH in the indoles on P(NIPAAm-co-Trp-OMe5) decreased with increasing temperature. The effect of temperature on the α value for fluocinonide and testosterone on P(NIPAAm-co-BMA5), P(NIPAAm-co-Phe-OMe5), and P(NIPAAm-co-Trp-OMe5) is shown in Fig. 7(b). The increase of the selectivity on P(NIPAAm-co-Trp-OMe5) with increasing temperature was small, whereas the selectivity on P(NIPAAm-co-BMA5) drastically increased with temperature. These results indicate that hydrogen bonding by the tryptophan moieties also contributed to the separation mode of the temperature-responsive chromatography.
 |
| Fig. 6 Chromatograms of (1) testosterone and (2) fluocinonide on (a) P(NIPAAm-co-BMA5), (b) P(NIPAAm-co-Phe-OMe5), and (c) P(NIPAAm-co-Trp-OMe5) HPLC columns at various temperatures. | |
 |
| Fig. 7 Effects of temperature on the (a) retention factor of fluocinonide and (b) selectivity for testosterone and fluocinonide on (○) P(NIPAAm-co-BMA5), (▲) P(NIPAAm-co-Phe-OMe5), and (■) P(NIPAAm-co-Trp-OMe5) HPLC columns. | |
Temperature-responsive chromatography for the separation of amino acids
The detection or identification of amino acids is important in biomedical and biochemical analysis for the determination of the primary structure of peptides and proteins. Commercial protein sequencing systems employing the Edman degradation method use HPLC with UV detection to analyze the PTH amino acid products that are produced from this process. First, we evaluated the effects of temperature on the elution behavior of the PTH amino acids. Fig. 8 shows the chromatograms obtained for a mixture of PTH amino acids using pure water as the mobile phase on the P(NIPAAm-co-Phe-OMe5), P(NIPAAm-co-Phe-OMe10), and P(NIPAAm-co-Trp-OMe5) columns upon changing the column temperature from 5 to 40 °C. Temperature dependent elution behavior was observed on each column, and well resolved chromatograms of nine amino acids were obtained at higher temperatures. The temperature dependence of the retention factors of nine PTH amino acids is compared on homo-PNIPAAm, P(NIPAAm-co-Phe-OMe5), P(NIPAAm-co-Trp-OMe5), and P(NIPAAm-co-Nap-OMe3) HPLC columns (Fig. 9). The retention factors of the amino acids increased with increasing temperature on all of the columns. Note that the retention of the aromatic amino acids was dramatically increased owing to π–π interactions on the P(NIPAAm-co-Phe-OMe5) and P(NIPAAm-co-Trp-OMe5)-modified silica columns compared with the PNIPAAm and P(NIPAAm-co-Nap-OMe3)-modified columns used in our previous report.15 Because of solubility, the content of naphthylalanine derivatives in PNIPAAm did not exceed 3%. In addition, the introduction of a more hydrophobic component, such as naphthylalanine, into PNIPAAm is expected to decrease the temperature-responsiveness of the polymer owing to increased hydrophobicity.
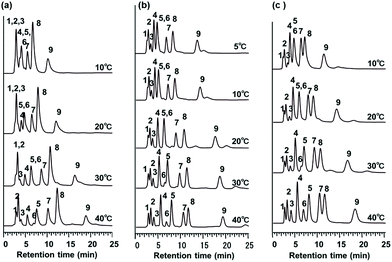 |
| Fig. 8 Chromatograms of PTH amino acids separated on (a) P(NIPAAm-co-Phe-OMe5), (b) P(NIPAAm-co-Phe-OMe10), and (c) P(NIPAAm-co-Trp-OMe5) HPLC columns. (1) PTH-Gly, (2) PTH-Ala, (3) PTH-Pro, (4) PTH-Met, (5) PTH-Leu, (6) PTH-Thr, (7) PTH-Phe, (8) PTH-Tyr, and (9) PTH-Trp. | |
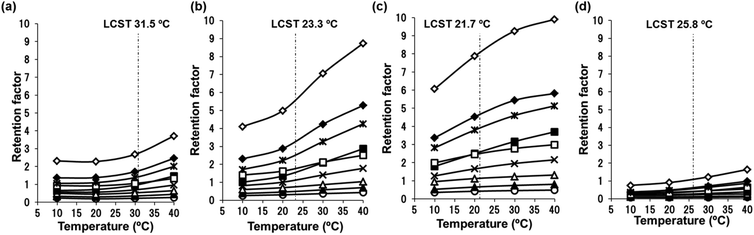 |
| Fig. 9 Temperature dependence of the retention factors of nine PTH amino acids on (a) PNIPAAm, (b) P(NIPAAm-co-Phe-OMe5), (c) P(NIPAAm-co-Trp-OMe5), and (d) P(NIPAAm-co-Nap-OMe3) HPLC columns. (○) PTH-Gly, (▲) PTH-Ala, (△) PTH-Pro, (×) PTH-Met, (■) PTH-Leu, (□) PTH-Thr, ( ) PTH-Phe, (◆) PTH-Tyr, and (◊) PTH-Trp. | |
Finally, a comparison of the separation selectivity for the PTH amino acids between PNIPAAm and the amino acid derivative-modified stationary phases was carried out. The effect of phenylalanine content in the column on the retention factors of the PTH amino acids is shown in Fig. 10(a). The retention factors of the aromatic amino acids were dramatically increased on the P(NIPAAm-co-Phe-OMe5) and P(NIPAAm-co-Phe-OMe10) columns compared with the homo-PNIPAAm column. As shown in Fig. 10(b), the amino acid derivative-modified columns showed greater selectivity for aromatic amino acids than the PNIPAAm column at 10 °C. These observations are consistent with π–π interactions between the aromatic components of the analytes and polymer. The results indicate that dual temperature-response and molecular recognition characteristics are active in the proposed separation system based on temperature-responsive polymers bearing aromatic amino acid derivatives.
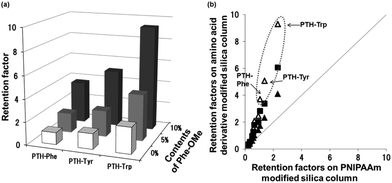 |
| Fig. 10 (a) Effects of the introduction of phenylalanine derivatives on the retention factors of PTH amino acids on PNIPAAm, P(NIPAAm-co-Phe-OMe5), and P(NIPAAm-co-Phe-OMe10) HPLC columns at 10 °C. (b) Comparison of retention factors of PTH amino acids on the amino acid derivative-modified silica columns and PNIPAAm-modified silica column at 10 °C. (▲) P(NIPAAm-co-Phe-OMe5), (△) P(NIPAAm-co-Phe-OMe10), (■) P(NIPAAm-co-Trp-OMe5). | |
Conclusions
In this study, we demonstrated an analytical method which is a novel temperature-responsive chromatographic system based on PNIPAAm containing aromatic amino acid derivatives as molecular-recognition sites, e.g. P(NIPAAm-co-Phe-OMe) and P(NIPAAm-co-Trp-OMe5)-modified silica columns. There are many reports of functional polymers synthesized by introducing amino acid derivatives to synthetic polymers23–25 and novel packing materials composed of poly(N-alanine)-grafted porous silica or poly(octadecyl acrylate-alt-N-octadecylmaleimide)-grafted silica with separation mechanisms explained by π–π interactions or shape-selectivity.26,27 However, we believe the present research is the first report to successfully demonstrate dual temperature-response and molecular recognition characteristics in a separation system utilizing temperature-responsive polymers bearing aromatic amino acid derivatives. These systems have the potential to be applied to the separation of complex and delicate peptides, proteins, and antibodies using only water as the eluent. Furthermore, the incorporation of amino acid moieties results in a chiral stationary phase that may contribute to the separation of such complex compounds.
Acknowledgements
This study was supported in part by a Grant-in-Aid for Scientific Research (B) (Grant No. 21390012 and 25293009) from the Ministry of Education, Culture, Sports, Science and Technology, Japan.
Notes and references
-
L. R. Snyder, J. J. Kirkland and J. W. Dolan, Introduction to Modern Liquid Chromatography, John Wiley & Sons, Inc., Hoboken, NJ, 2010 Search PubMed.
- K. Suwa, K. Morishita, A. Kishida and M. Akashi, J. Polym. Sci., Part A: Polym. Chem., 1997, 35, 3087–3094 CrossRef CAS.
- F. D. Jochum, L. Zur Borg, P. J. Roth and P. Theato, Macromolecules, 2009, 42, 7854–7862 CrossRef CAS.
- Y. Hiruta, T. Funatsu, M. Matsuura, J. Wang, E. Ayano and H. Kanazawa, Sens. Actuators, B, 2015, 207, 724–731 CrossRef CAS.
- Y. Hiruta, Y. Nagumo, Y. Suzuki, T. Funatsu, Y. Ishikawa and H. Kanazawa, Colloids Surf., B, 2015, 132, 299–304 CrossRef CAS PubMed.
- E. S. Gil and S. M. Hudson, Prog. Polym. Sci., 2004, 29, 1173–1222 CrossRef CAS.
- M. Heskins and J. E. Guillet, J. Macromol. Sci. Chem., 1968, 2, 1441–1455 CrossRef CAS.
- H. Kanazawa, K. Yamamoto, Y. Matsushima, N. Takai, A. Kikuchi, Y. Sakurai and T. Okano, Anal. Chem., 1996, 68, 100–105 CrossRef CAS PubMed.
- E. Ayano and H. Kanazawa, Anal. Sci., 2014, 30, 167–173 CrossRef CAS PubMed.
- Y. Hiruta, Y. Nagumo, A. Miki, T. Okano and H. Kanazawa, RSC Adv., 2015, 5, 73217–73224 RSC.
- M. Akimaru, K. Okubo, Y. Hiruta and H. Kanazawa, Anal. Sci., 2015, 31, 881–886 CrossRef CAS PubMed.
- Y. Hiruta, M. Shimamura, M. Matsuura, Y. Maekawa, T. Funatsu, Y. Suzuki, E. Ayano, T. Okano and H. Kanazawa, ACS Macro Lett., 2014, 3, 281–285 CrossRef CAS.
- H. Feil, Y. H. Bae, J. Feijen and S. W. Kim, Macromolecules, 1993, 26, 2496–2500 CrossRef CAS.
- H. Kanazawa, Y. Kashiwase, K. Yamamoto, Y. Matsushima, A. Kikuchi, Y. Sakurai and T. Okano, Anal. Chem., 1997, 69, 823–830 CrossRef CAS PubMed.
- T. Nishio, R. Kanazashi, A. Nojima, H. Kanazawa and T. Okano, J. Chromatogr. A, 2012, 1228, 148–154 CrossRef CAS PubMed.
- H. Kanazawa, E. Ayano, C. Sakamoto, R. Yoda, A. Kikuchi and T. Okano, J. Chromatogr. A, 2006, 1106, 152–158 CrossRef CAS PubMed.
- E. Ayano, C. Sakamoto, H. Kanazawa, A. Kikuchi and T. Okano, Anal. Sci., 2006, 22, 539–543 CrossRef CAS PubMed.
- F. Sanda, T. Abe and T. Endo, J. Polym. Sci., Part A: Polym. Chem., 1997, 35, 2619–2629 CrossRef CAS.
- B. L. Moore and R. K. O'Reilly, J. Polym. Sci., Part A: Polym. Chem., 2012, 50, 3567–3574 CrossRef CAS.
- K. Li, G. Tan, J. Huang, F. Song and J. You, Angew. Chem., Int. Ed., 2013, 52, 12942–12945 CrossRef CAS PubMed.
- R. P. Herrera, V. Sgarzani, L. Bernardi and A. Ricci, Angew. Chem., Int. Ed., 2005, 44, 6576–6579 CrossRef CAS PubMed.
- J. A. K. Howard, V. J. Hoy, D. O'Hagan and G. T. Smith, Tetrahedron, 1996, 52, 12613–12622 CrossRef CAS.
- H. Mori, K. Sutoh and T. Endo, Macromolecules, 2005, 38, 9055–9065 CrossRef CAS.
- M. Casolaro, R. Cini, B. Del Bello, M. Ferrali and E. Maellaro, Biomacromolecules, 2009, 10, 944–949 CrossRef CAS PubMed.
- X. Feng, Q. Zhang, X. Liang, J. Li, Y. Zhao and L. Chen, J. Polym. Res., 2014, 21, 1–9 CAS.
- A. Shundo, A. K. Mallik, T. Sakurai, M. Takafuji, S. Nagaoka and H. Ihara, J. Chromatogr. A, 2009, 1216, 6170–6176 CrossRef CAS PubMed.
- A. K. Mallik, T. Sawada, M. Takafuji and H. Ihara, Anal. Chem., 2010, 82, 3320–3328 CrossRef CAS PubMed.
Footnote |
† Electronic supplementary information (ESI) available: Scheme of the synthesis route for the stationary phase, chemical structures and log P values of the steroids, temperature-dependent retention factor, selectivity, chromatograms of naphthalene and 1,5-dinitronaphthalene, and 1H NMR spectra. See DOI: 10.1039/c5an01996f |
|
This journal is © The Royal Society of Chemistry 2016 |
Click here to see how this site uses Cookies. View our privacy policy here.