DOI:
10.1039/C5TB00618J
(Review Article)
J. Mater. Chem. B, 2015,
3, 4767-4779
The potential of nanoparticles for the immunization against viral infections
Received
4th April 2015
, Accepted 14th May 2015
First published on 14th May 2015
Abstract
Vaccination has a great impact on the prevention and control of infectious diseases. However, there are still many infectious diseases for which an effective vaccine is missing. Thirty years after the discovery of the AIDS-pathogen (human immunodeficiency virus, HIV) and intensive research, there is still no protective immunity against the HIV infection. Over the past decade, nanoparticulate systems such as virus-like particles, liposomes, polymers and inorganic nanoparticles have received attention as potential delivery vehicles which can be loaded or functionalized with active biomolecules (antigens and adjuvants). Here we compare the properties of different nanoparticulate systems and assess their potential for the development of new vaccines against a range of viral infections.
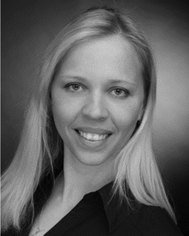
Viktoriya Sokolova
| Viktoriya Sokolova obtained a PhD degree in Inorganic Chemistry at the University of Duisburg-Essen in the group of Prof. Epple in 2006. In 2007, she received the Young Scientist Award of the Klee Family of the German Society for Biomedical Technology, the Award of the German Society for Biomaterials and she was in the final of the DSM Science & Technology Award 2007. Since 2011 she is a project leader in the group of Prof. Epple at the University of Duisburg-Essen. |
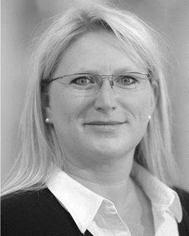
Astrid Maria Westendorf
| Astrid Westendorf studied biology at the Technical University of Braunschweig and obtained her PhD there in 2004. From 2004–2008, she worked as postdoctoral fellow in the group “Mucosal Immunology” at the Helmholtz Center for Infection Research in Braunschweig. In 2008, she became Junior Professor for Mucosal Immunology at the University of Duisburg-Essen and was awarded with the Robert-Koch Postdoc Prize for Immunology. In 2014, she became Full Professor for Infection Immunology at the Medical Faculty of the University of Duisburg-Essen. She is a member of the board of the German Society for Mucosal Immunology and Microbiom. |
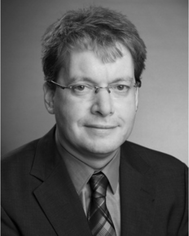
Jan Buer
| Jan Buer studied Medicine at the Medizinische Hochschule Hannover (MHH). He received his doctoral degree there in 1995. From 1996–1998 he did a postdoc with Harald von Boehmer at the Hôpital Necker in Paris. He is a board certified Medical Microbiologist. In 2006 Jan Buer received the Roche Applied Science Award “Imagining the future”. He has a strong track record in the field of immunoregulation of pathogen-specific immune responses. In 2003 he received the first joint Professorship between the Helmholtz Center for Infection Research in Braunschweig and the MHH. In 2007 Jan Buer was appointed as Director of the Institute for Medical Microbiology at the University of Duisburg-Essen. Jan Buer was Dean of Research of the Medical Faculty from 2008–2012. Since 2012 he is Dean of the Medical Faculty. He is Secretary & Board Member of German Society of Hygiene and Microbiology and elected member of the German Research Society research panel. |
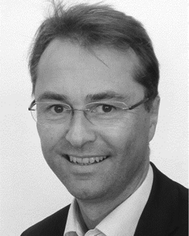
Klaus Überla
| Klaus Überla studied medicine at the University of Saarbrücken and the Free University of Berlin. After his PhD in immunology with Prof. T. Diamantstein (Free University of Berlin) he spent a postdoctorate from 1991–1993 with Prof. W. A. Haseltine at the Dana-Farber Cancer Institute at the Harvard Medical School in Boston (USA). In 1996, he obtained his Habilitation in virology at the University of Erlangen-Nürnberg with Prof. B. Fleckenstein. In 1993, he became Associate Professor of Virology at the University of Leipzig and in 2001 Full Professor of Molecular and Medical Virology at the Ruhr-University of Bochum. From 2010 to 2014 he served as Dean of the Faculty of Medicine at the Ruhr-University of Bochum. In 2015, he accepted the Chair of Virology at the University of Erlangen-Nürnberg. |
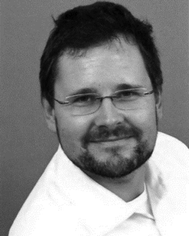
Matthias Epple
| Matthias Epple studied chemistry at the Technical University of Braunschweig and obtained bis PhD in 1992 with Prof. H. K. Cammenga. He went as a postdoctoral scientist to the University of Washington (Seattle) and finished his Habilitation in 1997 at the University of Hamburg with Prof. A. Reller. He carried out a number of research stays at the Royal Institution (London) with Prof. Sir J. M. Thomas. In 1998, he worked as Professor at the University of Augsburg and became Associate Professor at the University of Bochum in 2000. Since 2003, he is Full Professor for Inorganic Chemistry at the University of Duisburg-Essen. He was Dean of the Faculty of Chemistry from 2008–2011 and President of the German Society for Biomaterials from 2010–2013. |
Introduction
The fight against viral infections has been the subject of intense research for centuries, given the widespread prevalence of “traditional viral infections” (like polio, hepatitis, influenza) and “modern viral infections” (like HIV, bird flu, Ebola). In terms of the treatment of an infected patient, a prophylactic vaccination is clearly preferable. Therefore, studies on vaccination constitute a major goal of today's virological and immunological research.
The main goal of a vaccination is to induce a specific immunity with immunological memory against a pathogen in the recipient. The protection should last as long as possible, and the side effects should be as low as possible. Modern vaccination began in the late 18th century with the discovery of smallpox immunization by Edward Jenner. Infection with a non-pathogenic animal pox virus which is closely related to the human smallpox virus protected against the often fatal smallpox disease.1 Wide-spread and systematic application of this approach even led to eradication of the smallpox virus. Immunization by live, but non-pathogenic viruses also proved to be an efficient way to protect from a number of other viral diseases such as measles, rubella, and polio.
In the 20th century techniques for the cultivation of viruses were developed. This allowed a large scale production of viruses. After inactivation, these virus preparations were also successfully used for vaccination against e.g. hepatitis A and influenza. The development of recombinant DNA technologies allowed the development of vaccines against the hepatitis B virus (HBV) and the human papillomavirus (HPV) which cannot be propagated in cell culture. Since HBV infection can lead to liver cancer and HPV can cause cervical cancer, these vaccinations protect not only against infectious diseases, but also against tumor diseases.
In the last years, nanoparticles have been increasingly proposed as part of nanomedicine to selectively stimulate the immune system, both for prophylactic and therapeutic vaccination.2–8 They can be prepared in various sizes and loaded with different (bio-)molecules, including targeting moieties like antibodies to specifically address different cell types.9,10 This offers new possibilities because several cargo molecules (e.g. stimulatory molecules and antigen) can be delivered at the same time to the same cell, thereby provoking a fine-tuned immune response. Here we review the progress achieved in the last years in this exciting area.
The human immune system
The adaptive immune system has two arms to control viral infections. First, antibodies produced by B cells are able to prevent viruses from entering a cell and can therefore provide protection from infection. The second arm, cytotoxic CD8+ T cells, can recognize and eliminate virus infected cells and thereby control virus spread in an infected person. To prevent autoimmunity, both arms are controlled by CD4+ T helper cells. After immunization with a viral antigen, specialized cells of the innate immune system, the dendritic cells, take up the viral antigen and instruct T helper cells that are specific for the viral antigen to provide help for antibody-producing B cells and cytotoxic T cells (Fig. 1). A fundamental difference between B and T cell responses is that B cells recognize an antigen in its three-dimensional conformation, while T cells only react with small linear fragments of the antigen. These fragments, also called epitopes, are generated by dendritic cells after uptake and processing of the viral vaccine antigen. An important aspect for long-term protection is the differentiation of B and T cells into memory B cells and long-lived plasma cells and memory T cells, respectively. Due to their central role during induction of immune responses, dendritic cells have been established as promising target cells for vaccination.11,12 Dendritic cells take up and process vaccine antigens and present them by major histocompatibility complex (MHC) class II molecules to CD4+ T cells. Alternatively, antigens in the cytosol are presented by MHC class I molecules to cytotoxic CD8+ T cells. CD4+ T cells differentiate into CD4+ T helper cells and CD8+ T cells into cytotoxic lymphocytes which eliminate the virus selectively and efficiently.
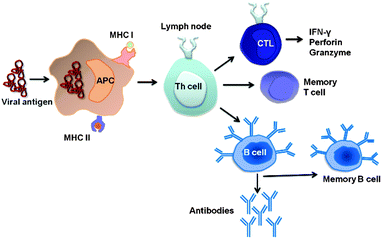 |
| Fig. 1 Schematic representation of the mechanisms of vaccination and immune defence against viruses. APC: Antigen-presenting cell (dendritic cell or B cell); MHC: Major histocompatibility complex; CTL: cytotoxic T lymphocytes (CD8+); Th: T helper cell. | |
After the decay of the primary immune response, both memory B and T cells are formed which after a new contact can immediately trigger an immune response with the viral antigen and therefore prevent the proliferation and survival of the virus in the case of a subsequent infection with the same virus (Fig. 1). The induction of a persistent memory against a virus without creating a harmful “real” infection is the main idea of vaccination.
Vaccination strategies
The main aim of today's vaccine research is to develop safe and effective strategies which may include either prophylactic (prior to infection) or therapeutic (in the case of an existing infection) vaccination to combat an infectious disease.1,13 Prophylactic vaccination prevents a possible infection after the vaccination. By prophylactic vaccination not only the disease in a given patient is prevented but also the spread of the virus in the population. This can permit a complete eradication of viruses that exclusively infect humans. This goal has almost been achieved for the polio virus, the pathogen of poliomyelitis (polio).14 Significant challenges remain with respect to the improvement of the efficacy of prophylactic vaccines including the extension of their duration or protection.
Notably, due to the success of vaccination, the perception of infectious diseases as a serious threat has declined in the population which reduces the willingness to be vaccinated and endangers the success of immunization programs. The fear of potential side-effects of vaccination and invasive vaccination techniques (like intramuscular injection) contribute to an aversion against vaccination, particularly in children. The development of less invasive atraumatic routes of administration for vaccines is therefore also important to increase the general acceptance of vaccination. Taking into account the global travel activities and potential threats of bioterrorism, it is another objective of current vaccination research to reduce the development time of new vaccines against rapidly spreading pathogens. The outbreak of the Ebola virus in 2014 has impressively demonstrated this necessity.
Although prophylactic vaccination is already being widely applied, the development of therapeutic vaccines against persistent viruses is particularly difficult. Persistent viruses trigger an anti-viral immune response which is not sufficient to entirely eliminate the virus from the body.15,16 Therefore, the infection remains (sometimes lifelong). Important human pathogens, such as HIV, hepatitis B and hepatitis C virus (HBV and HCV) and herpes viruses have developed various mechanisms to escape the innate and adaptive immune response (so-called immune escape).17 A therapeutic vaccination against these virulent diseases must therefore modify an existing anti-viral immune response so that the elimination of the virus despite the viral immune escape is possible.
Table 1 lists the currently used methods for immunization against viral infections. Vaccines based on attenuated viruses still allow the replication of the virus in the vaccinated person, but do not lead to a clinically manifest disease. They mimic a natural infection, activating the specific immune system, thereby protecting against a subsequent infection with the same pathogen. Inactivated virus vaccines (for example, after the formaldehyde treatment) contain whole virus particles which are not able to proliferate but still can induce the necessary immune response. The isolation of specific subunits (proteins) from inactivated virus particles leads to subunit vaccines. Alternatively, viral proteins for vaccination can also be produced by recombinant DNA technologies.
Table 1 Comparison of different antiviral immunization strategies
Type of vaccine |
Advantages |
Disadvantages |
Attenuated virus40 |
Induces humoral and cellular responses, low doses sufficient, low costs |
Possible reversion to virulence; risky to use in immuno-compromised patients; difficult to assess the safety |
Inactivated (killed) virus41 |
Induces humoral response; can be used in immunocompromised patients |
Requires multiple booster vaccinations; does not induce a cellular response |
Virus subunit42 |
Large-scale production possible; fewer side effects |
Only moderately immunogenic; antigens may not retain their native conformation |
Virus-like particles (VLPs)18 |
Induce good humoral and cellular responses |
Complex production process |
DNA vaccines43 |
Induce good cellular responses and modest antibody responses; highly stable; modifications easily possible; may encode multiple antigens; standardized large scale production |
Invasive delivery; immunotoxicity; induction of tolerance; antibiotic resistance |
Nanoparticles44 |
Induce humoral and cellular responses; highly stable; modifications possible; can carry multiple antigens, adjuvants, targeting moieties; well-defined composition; large-scale production possible |
Possible loss of the native conformation of biomolecules during synthesis; only moderately immunogenic; extensive characterization necessary before clinical approval (e.g. particle size, particle charge, composition, amount of drug loading, biodistribution and fate after administration) |
Instead to immunize directly with proteins of the virus, either as an entire particle or a subunit protein, is also possible to deliver genes (DNA) encoding one or more viral proteins. These gene-based vaccines consist of either a purified DNA or particles that transport the gene of interest. In both cases, the gene must be taken up by cells of the vaccinated person and be translated into the viral proteins against which the specific immune response is required.
Because soluble proteins generally induce only a weak immune response, it was tried to incorporate the genetically engineered viral proteins into virus-like particles (VLPs). VLPs mimic the parental virus in its protein structure, but are not infectious because the virus genome is present only in fragments or not at all. The particulate structure of the VLPs and the multiple occurrence of the same antigen molecule on the surface of the same VLPs lead to a particularly efficient immune response.18 In analogy to VLPs, nanoparticles consisting of lipids, polymers or inorganic compounds were also prepared. Nanoparticles are typically functionalized with viral proteins or peptides for immunization and have a size up to several hundred nanometers (see the extended discussion below).
Despite substantial progress in understanding the interaction of pathogens with the immune system, there is still an acute need to develop effective and safe vaccines against important viral diseases including AIDS, Ebola and Dengue fever.19 Because of the lack of treatment options and funding for existing therapies and medical care in developing countries, new inexpensive immunization procedures are of particular urgency. For instance, the unsatisfactory therapeutic approaches to chronic viral hepatitis and to recurrent herpes virus infections in immunosuppressed persons require new immunization methods. For the prophylaxis and therapy of infectious viral and bacterial diarrhea which remains a major cause of mortality in infancy in developing countries, there are no convincing vaccination strategies today.20
Adjuvants
An adjuvant is defined as an additive that improves the adaptive immune response or stimulates the innate immune system in such a way that the desired effectors or mediators are efficiently induced (Table 2).19,21 Thereby they enhance the immune response and lead to a successful vaccination. Despite the need for new adjuvants, only very few compounds that have been selected in pre-clinical vaccine studies have been licensed for human use.22Table 2 shows a compilation of these vaccine adjuvants.
Table 2 Some examples of adjuvants that are currently approved or under clinical development
Adjuvant |
Composition |
Stage of development |
Activated immune response |
Indications |
Alum45 |
Al(OH)3 |
Licensed |
Cellular (Th2) and humoral |
HAV, HBV, HPV, DT, HIB, pneumococcal conjugate |
ASO330,46 |
O/W emulsion + vitamin E |
Licensed |
Humoral |
Pandemic Influenza
|
ASO447 |
Monophosphoryl lipid A from Salmonella minnesota + Al(OH)3 |
Licensed |
Cellular (Th1) and humoral |
HBV, HPV |
MF5929,48,49 |
O/W emulsion |
Licensed |
Cellular (Th2) |
Influenza, malaria, HBV, HIV |
CpG50–52 |
Oligonucleotide |
Under development |
Cellular and humoral |
HBV, malaria, HVC and cancer |
Flagellin53–56 |
Flagellin, linked to an antigen |
Under development |
Cellular and humoral |
Influenza |
IC3134,35,57 |
Peptide–oligonucleotide conjugate |
Under development |
Cellular and humoral |
Tuberculosis |
Poly(I:C)36,37,58 |
dsRNA |
Under development |
Cellular and humoral |
HBV, malaria, HCV, and cancer |
Saponin (QS21)59,60 |
Triterpene glycosides, isolated from plants |
Under development |
Cellular (Th1) and humoral |
Malaria, influenza and cancer |
Aluminium hydroxide (“Alum”) is the most widely used adjuvant which has been used for more than 70 years. It is applied in vaccines against the hepatitis A virus (HAV), the hepatitis B virus (HBV), the human papillomavirus (HPV), diphtheria and tetanus (DT), haemophilus influenza type B (HIB), and pneumococcal conjugates. Alum increases the antigen uptake and stability at the site of injection.23–25 A disadvantage of alum is a poor induction of a protective Th-1-associated immune response which is crucial for the development of vaccines against intracellular pathogens. Li et al. showed that by reducing the particle size of the traditional aluminum hydroxide adjuvant from micrometer to nanometer scale, the nanoparticles induced a stronger antigen-specific antibody response.26 Sun et al. demonstrated that shape, crystallinity and hydroxide content of aluminum oxyhydroxide (AlOOH) nanoparticles play an important role in NLRP3 inflammasome activation. They showed that the AlOOH nanorods with the highest hydroxide content and the lowest crystallinity were the most efficient ones in comparison to commercial Alum.27 Wang et al. developed phospholipid bilayer-coated aluminum nanoparticles which induced a mixed Th1/Th2 immunoresponse to establish humoral, cellular and even mucosal immunity to the corresponding antigen in mice.28
The oil–water emulsion MF59 has been licensed in Europe in 1997 as an adjuvant for its capacity to increase the immunogenicity of influenza vaccines in elderly persons.29 An oil-in-water emulsion called AS03 has been licensed in Europe for pandemic flu vaccination.30 A Toll-like receptor 4 (TLR4) agonist (monophosphoryl lipid A from Salmonella minnesota + Al(OH)3) known as AS04 is currently approved for use against human papilloma virus and hepatitis B.31,32 Calcium phosphate has also been proposed as an adjuvant.33 A TLR9 agonist-based adjuvant called IC31 consists of a cationic peptide and the immunoactive oligonucleotide.34 IC31 is currently undergoing human clinical trials in a vaccine against tuberculosis (TB).35
Poly(I:C) is a mimic of viral double-stranded (ds)RNA and a promising adjuvant for vaccines directed against viral pathogens. Poly(I:C) is a ligand for TLR3 which is located in the membrane of the endosomal compartments of most antigen presenting cells and also stimulates RIG-I-like-receptors.36 We have recently compared the effects of poly(I:C) and the oligonucleotide CpG (TLR9 ligand) in nanoparticulate formulations.37
Saponins are glycosylated natural products isolated from plants which are used as adjuvants in vaccines. Saponins are embedded into ISCOMs (immune stimulating complexes) which are used for viral envelope proteins of herpes simplex virus type 1, HBV and influenza. ISCOMs are supramolecular aggregates of cholesterol, phospholipids and saponin.38
Adjuvants allow to reduce the necessary amount of vaccine antigen by increasing the immune reaction of the body. This accelerates the development of vaccines against pandemic pathogens and can decrease the cost of vaccine production. Also, an adjuvant can improve the efficiency of vaccines by influencing the type of immune response. However, there are indications that with a particular combination of an adjuvant and a vaccine antigen under a certain genetic disposition, autoimmune side effects may occur.39 This depends on the antigen–adjuvants combination, therefore each combination must be assessed separately in a risk-benefit analysis.39
Administration routes for vaccination
Potential advantages and disadvantages of different routes of vaccine administration are given in Table 3. The typical vaccination methods include an intramuscular or a subcutaneous injection which triggers a systemic immune response. Mucosal administration of vaccines (via the mucous membranes of the respiratory tract, the gastrointestinal tract or the genital tract) is a comparatively new approach to induce a local and systemic immunity.61–63 These local immune responses in the mucous membranes should efficiently prevent the penetration of the pathogen. Examples include the local protection in the mucous membranes of the lungs against the TBC pathogen or a specific protection against an HIV infection in the genital mucosa.64,65 The administration of vaccines via the mucous membranes also has the advantage that the vaccines are taken up atraumatically (i.e. without injection).
Table 3 Possible administration routes for vaccines
Administration route |
Advantages |
Disadvantages |
Injection |
Intramuscular (into the muscles) |
Clinically relevant; induces systemic immunity |
Pain; trained staff required |
Subcutaneous (into the skin) |
Clinically relevant; induces systemic immunity |
Pain; trained staff required |
Intravenous (into the vein) |
Rapid vaccine distribution in the body; clinical relevance possible |
Pain; trained staff required; probably unsuitable for vaccination |
Intraperitoneal (into the abdominal cavity) |
Clinical relevance doubtful |
Pain, trained staff required; high infection risk |
Oral |
Under the tongue |
Convenient; inexpensive mass vaccination possible; induces mucosal immunity |
Stability of the vaccine in the gastro-intestinal tract often not given |
Inhalation |
Intranasal/pulmonal |
Induces mucosal and lung immunity |
Risk of respiratory distress in the case of inflammatory reaction |
Microneedle |
Transcutaneous plaster |
Non-invasive; rapid; pain-free administration |
Under development |
The very high patient compliancy of oral vaccination against polio is a good example for this convenient method. Needle-free transcutaneous methods such as patches, coated with a vaccine and used by the patient himself are recent developments (Fig. 2)66 which may increase the acceptance of vaccination by patients in the future. However, efficiency, stability, dosage and possible side effects, must all be quantitatively investigated before these routes of vaccination can be clinically applied on a routine basis. Therefore, there is a need for new vaccines that can be administered in different ways and that show a prolonged release of antigen at the site of administration.
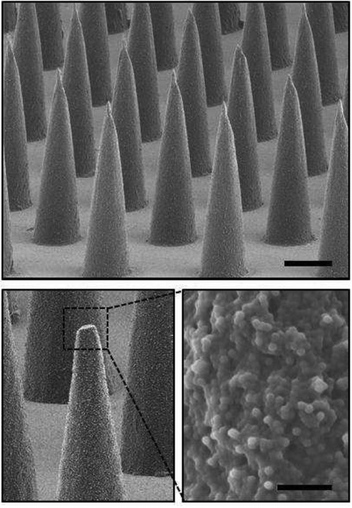 |
| Fig. 2 Drug-loaded microparticles are deposited onto microneedles and injected subcutaneously by a patch. Scanning electron micrographs of the resulting microparticle-encapsulating microneedle array (scale bar 200 μm) and high magnification image of the composite needle interior of a fractured microneedle (scale bar 10 μm). Reprinted with permission from Elsevier66 Copyright 2013. | |
It should also be pointed out that not all vaccination methods which are applied in experimental animal studies will be finally applicable to patients (e.g. an intraperitoneal injection).
In general, the route of vaccine administration is a critical factor for success of the immunization, as active biomolecules have to be transported from the site of entry to the part of the body where their action should take place.67
Nanoparticulate systems for vaccination
Nanoscopic systems have a large potential in biomedicine and in immunology.22,44,68,69Fig. 3 compares the sizes of the units that are relevant for vaccination: An influenza virus has a diameter of approximately 100 nm, calcium phosphate nanoparticles have a diameter in the range of about 150 nm, antigen-presenting cells of the immune system (for example, a dendritic cell) and specific T cells have a diameter of a few micrometers. This comparison illustrates very nicely that relatively small viruses are able to attack large cells, but also that nanoparticles are small enough to be efficiently taken up by cells (typically by endocytosis and related processes).70–74 It must be noted, however, that the calcium phosphate nanoparticles shown here are comparatively large. Gold, magnetite and silica nanoparticles, as frequently used in nanomedicine, are often smaller than 50 nm.4,5,8,75–77
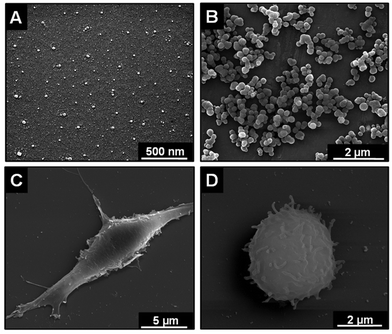 |
| Fig. 3 Scanning electron micrographs of influenza virus (A), of functionalized calcium phosphate nanoparticles (B), of primary murine dendritic cells (C), and of primary murine T cells (D). After the preparation the viruses appear to be smaller than in the natural state which is due to the fixation and drying processes. | |
Instead of the administration of whole viruses (as in conventional vaccines), nanoparticles can be selectively loaded and functionalized with different biomolecules, and also with several biomolecules on the same nanoparticle.2,3,6–8,77,78 Typical membrane antigens such as polysaccharides, proteins, lipoproteins and glycoproteins are often less immunogenic than whole viruses and therefore require an adjuvant to enhance the immune response. Thus, it is favourable that antigens and molecular adjuvants can be administered together with the same nanoparticle. Particulate delivery systems such as emulsions, liposomes, nanoparticles, and polymers can simultaneously transport the biomolecules and protect both antigen and immunostimulating biomolecules against biodegradation.79–81 Nanoparticles are able to enter the cells by endocytosis or phagocytosis and to address intracellular receptors.73,74,82,83Table 4 summarizes the currently used nanoparticulate systems.
Table 4 Selected examples of nanoparticulate systems as vaccine carriers
Vaccine carriers |
Examples |
Size/nm |
Antigen (s) |
Administration route |
Virus-like particles (VLPs) |
Influenza VLPs |
80–120 |
Haemagglutinin (H1N1) |
Intranasal84 |
Liposomes |
MPLA; |
50–2000 |
Cholera toxin |
Oral85 |
Phospholipid S100 and cholesterol; |
|
Lipid A |
Intravenous86 |
Phosphatidylcholine and cholesterol |
|
Tetanus toxoid |
Intranasal87 |
Polymers |
Poly(lactide-co-glycolide) (PLGA) |
90–200 |
Docetaxel |
Intramuscular88 |
Poly(lactic acid) (PLA) |
|
Malaria antigen (R32NS1) |
Intramuscular81,89 |
Chitosan |
250–350 |
Ovalbumin |
Intranasal and intradermal90 |
Inorganic nanoparticles |
Calcium phosphate |
100–250 |
Haemagglutinin (fragment of the influenza virus) |
Intraperitoneal and intranasal91 |
Gold |
5–50 |
Plasmid–DNA |
Intramuscular92 |
Gold |
12 |
Matrix 2 protein (M2e) of the influenza virus |
Intranasal93 |
Silica |
200 |
Porcine circovirus type 2 ORF2-proteine (PCV2 GST-ORF2) |
Intramuscular94 |
Virus-like particles (VLPs) have a diameter in the range of 20–150 nm and, as described above, consist of a self-assembled viral envelope and/or capsid proteins of viruses.95,96 In many cases, such VLPs have structural features and an antigenicity similar to the original virus, but are not infectious because they lack its replication ability.97 Polysaccharides or small organic molecules may be chemically linked to the surface of VLPs to enhance the immune response.98–100 The advantage of VLPs is that they are able to induce both a strong humoral (antibodies) and a cellular immune response.101,102 Some prophylactic VLP-based vaccines are currently commercially available, e.g. against HBV and HPV.32 Other VLP-based vaccine candidates are currently in clinical trials, e.g. against influenza or parvoviruses.95 Evans et al. reported the production of VLPs of the L1 protein of HPV which induced strong B and T cell responses.103 Olsson et al. developed a prophylactic VLP vaccine against HPV types 6/11/16/18 L1 and showed the induction of long-term immunological memory.104 VLPs are also promising vaccine candidates against the influenza virus.105 Quan et al. developed VLPs which consist of influenza virus proteins A/PR8/34 (H1N1), hemagglutinin (HA), and matrix proteins M1 and showed that after intranasal immunization, the induction of serum and mucosal antibody titers with neutralizing activity occurred against PR8 and A/WSN/33 (H1N1) viruses.84 Pushko et al. reported a new VLP platform which contained the three hemagglutinin (HA) subtypes of H5N1, H7N2 and H2N3-influenza viruses and showed the protective properties of such nanoparticles.106 VLPs have also been proposed for vaccination against HIV.107,108
Liposomes are self-assembled structures of a surfactant bilayer (usually phospholipids) with an aqueous core. By the hydrophilic character of the inner and outer side they can easily transport water-soluble molecules in the body.109 Burke et al. showed how a liposomal formulation can be used for the transport of the diphtheria toxin and the adjuvant poly(I:C) during the immunization.110 Mishra et al. used subcutaneously administered lipid nanoparticles as carriers for the vaccination against hepatitis B.111 Baca-Estrada et al. have applied liposomes for intranasal vaccination against the plague pathogen Yersinia Pestis.112 Heurtault et al. has reviewed the application of liposomes for nasal vaccination.113
Nanoparticles made of natural and synthetic polymers are very promising for medical research as they can be very well covalently functionalized on the surface.114–117 They can be used as carriers of vaccines (inside the particle), as well as for the presentation of vaccines (on the surface). In nanomedicine, synthetic polyesters, such as polylactic acid (polylactide) (PLA),118–120 and related copolymers, such as poly (lactide-co-glycolide) (PLGA)121–124 have been used in the form of microparticles and nanoparticles for the transport and release of bioactive molecules. They have several advantages, mainly their non-toxic degradation products (lactic acid or glycolic acid), the biodegradability which can be adjusted by the polymer composition, and last but not least, the approval by the FDA as biomaterial.116,125–127
PLGA-particles can carry all kinds of biomolecules and synthetic drugs, including proteins, peptides, DNA, and oligonucleotides.123,128–132 In immunology, PLGA particles were used as a delivery systems for several vaccines, containing a protein antigen or plasmid DNA.121,133,134 Ma et al. reported that PLGA nanoparticles loaded with tumour antigen peptides induced a strong cytotoxic T cell response (CTL) in vivo.124 Fischer et al. demonstrated the induction of the cellular immune response by PLGA particles, which were loaded with a CTL restricted peptide antigen and a CpG oligonucleotide (TLR9 Ligand), as an adjuvant. These PLGA particles induced a significant CTL immune response in mice 6 days after the immunization.123 PLGA particles were also investigated several times for immunization against HBV.122,135,136 Feng et al. synthesized PLGA particles with a recombinant HBV surface antigen (HBsAg) by a double emulsion microencapsulation process and demonstrated the induction of the immune response in vitro and in vivo.122 Grabbe et al. have recently demonstrated how covalently functionalized nanoparticles of synthetic polymers can be used to stimulate primary dendritic cells.137,138
Natural polymers like chitosan are also applied in immunization. Chitosan is a naturally occurring polysaccharide, biodegradable and non-toxic.139–142 Seferian et al. showed the immune-stimulatory effect of chitosan nanoparticles through the activation of macrophages.143 It has been also reported that antigen-loaded chitosan nanoparticles can induce a significant serum IgG response in vivo by intranasal administration and that animals were protected against the corresponding viruses. Furthermore, the intranasal administration of chitosan nanoparticles was also tested against the influenza virus in humans.90,144
Inorganic nanoparticles are “harder” than surfactant- or polymer-based systems. Inorganic nanoparticles like gold, silica, and calcium phosphate have been used for the immunization. They have several advantages compared to organic nanoparticles. Inorganic nanoparticles are usually non-toxic, not sensitive to microbial or hydrolytic degradation and therefore easy to store. They can often be conveniently produced from inorganic precursors and covalently functionalized with different molecules (Table 4). Consequently, they were used as antigen delivery systems for a variety of biomedical applications.145–147
Gold nanoparticles are non-biodegradable materials. For decades, gold nanoparticles have been studied for biomedical applications. They can be easily synthesized and also covalently functionalized (typically by conjugating thiol-functionalized molecules), therefore they can carry many types of molecules, like antibodies, nucleic acids or proteins.76,148–150 Gold nanoparticles with the size of 2–50 nm are used, for example, for the transport of biomolecules,92,151,152 for tumour thermotherapy,153 as cytostatic agent and as contrast agent.154 However, with a functionalization on the surface, the biomolecules have no protection against degradation, e.g. by hydrolysis or enzymes like nucleases or proteases. Tao et al. developed an influenza vaccine by conjugation of an extracellular region of the matrix 2-protein (M2e) of the influenza A virus to the surface of gold nanoparticles, and tested them in a mouse influenza model. They showed that intranasal immunization of mice with M2e–gold conjugates induced the production of M2e-specific IgG serum antibodies.93
Calcium phosphate nanoparticles are suitable carriers for biomolecules155–158 as they can transport many molecules across the cell membrane.159,160 If they are prepared in a multi-shell way, they can protect an encapsulated cargo of biomolecules against enzymatic degradation.161,162 Calcium phosphate dissolves at a low pH (about 5) in the lysosomes within the cell, so that the entrapped active biomolecules are released.163,164 Calcium phosphate was also proposed as adjuvant due to an immunostimulatory effect on its own.33,165 Calcium phosphate nanoparticles can be used as delivery systems for immunoactive oligonucleotides to activate dendritic cells (Fig. 4). The immunostimulatory effect of calcium phosphate nanoparticles on dendritic cells was detected by the enhanced expression of co-stimulatory molecules (MHC II) and the secretion of cytokines in vitro.166,167
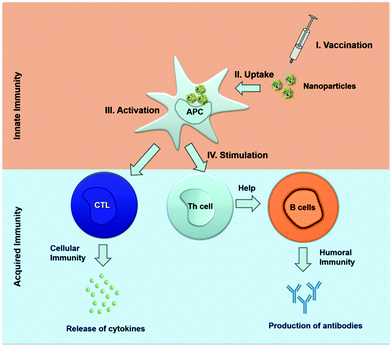 |
| Fig. 4 Schematic representation of the mechanisms of the induction of both innate and acquired immunity by activation of dendritic cells with the help of nanoparticles. APC antigen presenting cells; CTL cytotoxic T cells; Th T helper cells. | |
We have recently reported about a novel immunization approach with calcium phosphate nanoparticles that can serve as delivery system for a TLR9 ligand (CpG) and a viral antigen from the influenza A virus (hemagglutinin). Functionalized calcium phosphate nanoparticles were effectively taken up by dendritic cells in vivo and induced a strong immune response in immunized mice, based on high numbers of IFN-γ-producing antigen-specific CD4+ and CD8+ T cells (Fig. 5). By this, we were able to achieve a potent T cell-mediated protection against influenza.91
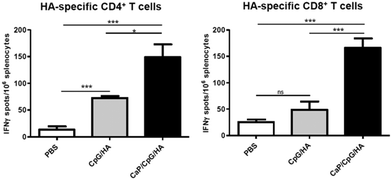 |
| Fig. 5 BALB/c mice were immunized three times intraperitoneally with calcium phosphate nanoparticles, loaded with the adjuvant CpG (TLR9 ligand) and the influenza HA antigen (CaP/CpG/HA). As controls, phosphate-buffered saline (PBS) and the dissolved biomolecules without nanoparticles in the same concentration (CpG/HA) were used. Two weeks after the final immunization, the number of HA-specific CD4+ and CD8+ T cells was strongly increased which demonstrates the effective immune response to the antigen HA. *p < 0.05; ***p < 0.001; ns = not significant. Reprinted with permission from Elsevier,91 Copyright 2013. The American Association of Immunologists, Inc. | |
In addition, we could show that calcium phosphate nanoparticles, functionalized with CpG and retroviral T cell epitopes are an excellent vaccination tool against acute and chronic retroviral infection. A one shot–shot immunization of chronically retrovirus infected mice efficiently reactivated effector T cells which led to a significant decrease in viral loads (Fig. 6).9 We identified a clear correlation between the percentage of CD8+ effector T cells and the viral loads: more CD8+ effector T cells in the spleen were correlated with lower viral loads. This demonstrates that an immunization with antigen/adjuvant-functionalized calcium phosphate nanoparticles is a very efficient method for the induction or reactivation of retrovirus-specific T cell responses, and that they represent a promising approach to improve antiretroviral vaccination. In addition, we could show that antigen-specific B cells can be targeted and activated by functionalized calcium phosphate nanoparticles.168
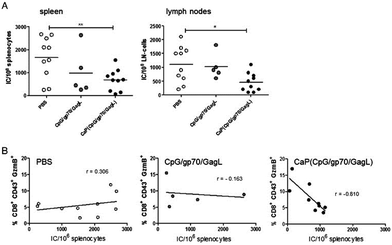 |
| Fig. 6 Therapeutic vaccination with functionalized calcium phosphate nanoparticles reduces the load of the Friend virus, a retrovirus. (A) Viral loads in the spleen and peripheral lymph nodes 7 days after the vaccination of chronically infected mice. (B) Correlation between the percentage of CD8+ effector T cells and the viral load after immunization. Reprinted with permission from Elsevier,170 Copyright 2015. | |
Sahdev et al. reported about calcium phosphate nanoparticles for transcutaneous delivery of vaccines. They showed that nanoparticles coated with sugar and ovalbumin can strongly induce the production of antibodies.169 Furthermore, an immunostimulatory effect of calcium phosphate was shown by He et al.33,165 Calcium phosphate nanoparticles can serve as vaccine delivery systems that allow the flexible dosage of adjuvants and antigens, which is important for the optimization of vaccines.
Silica nanoparticles can be easily synthesized and functionalized by the covalent attachment to silanol groups on the surface.75,171 Especially suitable are mesoporous nanoparticles which can carry active biomolecules inside the pores and release them in a desired organ or tissue.172,173 In such pores, a protein antigen can be protected from degradation so that an oral administration is possible.174,175 Kim et al. developed an approach in which host immune cells are modulated in vivo by 3D scaffolds that spontaneously assemble from mesoporous silica rods. Such mesoporous silica rods were able to enhance systemic helper T cells (Th1 and Th2), serum antibodies and cytotoxic T-cells.176
Nanoparticles can enter a cell by different mechanisms: By clathrin- or caveolin-mediated endocytosis, by unmediated endocytosis, by macropinocytosis and by phagocytosis, depending on the cell type and the nature of the nanoparticles.73,74,164,177–179
Foged et al. reported that particle size and surface charge play a significant role in the cellular uptake of nanoparticles in vitro.180 Gutierro et al. showed that the immune response after subcutaneous, oral or intranasal administration of nanoparticles directly depends on the particle size.181 Also, the shape of the nanoparticles seems to play a role.182,183 After the uptake, the nanoparticles typically are inside an endosome, which further merges with a lysosome, where the nanoparticles and their cargo are exposed to an acidic pH (4–5) and degrading enzymes (nucleases, proteases, lipases). Therefore, it is important to allow the endosomal escape of the cargo before the enzymatic degradation. This can be achieved for example by increasing the osmotic pressure inside of the lysosome by the influx of ions. The classical example is poly(ethyleneimine), which is well known as a cationic polyelectrolyte, which induces the influx of acid (the so-called proton sponge effect, leading to endosomal escape).184 The size of nanoparticles can strongly affect their distribution, kinetics and intracellular processing pathways in vivo.185 Manolova et al. showed that nanoparticles traffic to the draining lymph nodes in a size-dependent manner: Large particles (500–2000 nm) were mostly associated with dendritic cells in the site of injection and small nanoparticles (20–200 nm) were mostly found in lymph nodes-resident dendritic cells and macrophages.186
It is also advantageous if the biomolecules are protected from degradation by the incorporation into the nanoparticles.187 Another plus point for the application of nanoparticles for the vaccination is the fact that they can transport different biomolecules (e.g. adjuvants and antigens) in a defined concentration ratio at the same time in a specific cell.167 This is favourable compared to the dissolved biomolecules alone, which are distributed in the tissue and in the bloodstream. Furthermore, it is possible to functionalize the nanoparticles on the surface with targeting molecules such as antibodies, peptides, aptamers, and polysaccharides, in order to address specific cells or organs.188–192 Nanoparticles as antiviral vaccines can also integrate other attractive properties, such as slow release of active biomolecules and specific cell targeting.193
The main problem in transportation and storage of vaccine is the risk of protein denaturation and degradation of the applied biomolecules, for example, by nucleases and proteases. In this regard, nanoparticles offer further possibilities. For example, the dehydration by freeze-drying194 leads to storable products and to water-redispersible vaccines.
Conclusions
Nanoparticles are promising materials to carry immunoactive biomolecules for the induction of virus specific immune response. They have some advantages over traditional vaccines: The size of the nanoparticles can be controlled to allow the transport through biological barriers. Vaccine antigens can be protected inside nanoparticles from biodegradation. Different bioactive molecules can be incorporated into one nanoparticle. Nanoparticles can be functionalized on the surface with specific biomolecules to address targeted cells and release antigens. Vaccines on the basis of nanoparticles are therefore a flexible system in order to induce specific immune responses and activate both paths of the immunological memory.
Acknowledgements
We thank Torben Knuschke, Diana Kozlova, Kateryna Loza and Olga Rotan for experimental assistance in the preparation of nanoparticles, cells and viruses for the scanning electron microscopy images. Jan Buer, Matthias Epple, Klaus Überla and Astrid Westendorf thank the DFG for financial support within the framework of Transregio TRR 60. Jan Buer and Klaus Überla thank the Mercator-Stiftung (Essen) for financial support.
Notes and references
- J. L. Hsu, S. D. J. Med., 2013, 33–37 Search PubMed.
- T. Sun, Y. S. Zhang, B. Pang, D. C. Hyun, M. Yang and Y. Xia, Angew. Chem., Int. Ed., 2014, 53, 12320–12364 CAS.
- V. K. Khanna, ISRN Pharmacol., 2012, 2012, 9 Search PubMed.
- T. L. Doane and C. Burda, Chem. Soc. Rev., 2012, 41, 2885–2911 RSC.
- W. J. Stark, Angew. Chem., 2011, 123, 1276–1293 CrossRef PubMed.
- H. Laroui, D. S. Wilson, G. Dalmasso, K. Salaita, N. Murthy, S. V. Sitaraman and D. Merlin, Am. J. Physiol.: Gastrointest. Liver Physiol., 2011, 300, G371–G383 CrossRef CAS PubMed.
- B. Y. S. Kim, J. T. Rutka and W. C. W. Chan, N. Engl. J. Med., 2010, 363, 2434–2443 CrossRef CAS PubMed.
- K. Riehemann, S. W. Schneider, T. A. Luger, B. Godin, M. Ferrari and H. Fuchs, Angew. Chem., Int. Ed., 2009, 48, 872–897 CrossRef CAS PubMed.
- D. Kozlova and M. Epple, BioNanoMaterials, 2013, 14, 161–170 CrossRef.
- F. Kiessling, M. E. Mertens, J. Grimm and T. Lammers, Radiology, 2014, 273, 10–28 CrossRef PubMed.
- R. M. Steinman and J. Banchereau, Nature, 2007, 7161, 419–426 CrossRef PubMed.
- H. Kumar, T. Kawai and S. Akira, Int. Rev. Immunol., 2011, 30, 16–34 CrossRef CAS PubMed.
- M. Lombard, P. P. Pastoret and A. M. Moulin, Rev. Sci. Tech. Off. Int. Epiz., 2007, 26, 29–48 CAS.
- A. Shahzad and G. Kohler, Vaccine, 2009, 27, 5293–5294 CrossRef PubMed.
- B. M. Sullivan, J. R. Teijaro, J. C. de la Torre and M. B. A. Oldstone, PLoS Pathog., 2015, 11, e1004588 Search PubMed.
- A. Busca and A. Kumar, Virology, 2014, 11, 22 CrossRef PubMed.
- U. Protzer and H. Schaller, Virus Genes, 2000, 1–2, 27–37 CrossRef.
- G. T. Jennings and M. F. Bachmann, Biol. Chem., 2008, 389, 521–536 CrossRef CAS.
- M. L. Mbow, E. De Gregorio, N. M. Valiante and R. Rappuoli, Curr. Opin. Immunol., 2010, 22, 411–416 CrossRef CAS PubMed.
- P. Erlich and P. J. Sansonetti, Presse Med., 2013, 42, 93–101 CrossRef PubMed.
- A. M. Harandi, D. Medaglini and R. J. Shattock, Vaccine, 2010, 28, 2363–2366 CrossRef PubMed.
- D. M. Smith, J. K. Simon and J. R. Baker Jr., Nat. Rev. Immunol., 2013, 13, 592–605 CrossRef CAS PubMed.
- H. HogenEsch, Vaccine, 2002, 20, S34–S39 CrossRef CAS.
- G. L. Morefield, A. Sokolovska, D. Jiang, H. HogenEsch, J. P. Robinson and S. L. Hem, Vaccine, 2005, 23, 1588–1595 CrossRef CAS PubMed.
- A. S. Arnold, Y. L. Tang, K. P. Qian, L. P. Shen, V. Valencia, M. I. Phillips and Y. C. Zhang, J. Hypertens., 2007, 25, 197–205 CrossRef CAS PubMed.
- X. Li, A. M. Aldayel and Z. Cui, J. Controlled Release, 2014, 173, 148–157 CrossRef CAS PubMed.
- B. Sun, Z. Ji, Y. P. Liao, M. Wang, X. Wang, J. Dong, C. H. Chang, R. Li, H. Zhang, A. E. Nel and T. Xia, ACS Nano, 2013, 7, 10834–10849 CrossRef CAS PubMed.
- T. Wang, Y. Zhen, X. Ma, B. Wei and N. Wang, ACS Appl. Mater. Interfaces, 2015, 12, 6391–6396 Search PubMed.
- A. Podda, Vaccine, 2001, 19, 2673–2680 CrossRef CAS.
- G. Leroux-Roels, Expert Opin. Biol. Ther., 2009, 9, 1057–1071 CrossRef CAS PubMed.
- S. L. Giannini, E. Hanon, P. Moris, M. Van Mechelen, S. Morel, F. Dessy, M. A. Fourneau, B. Colau, J. Suzich, G. Losonksy, M. T. Martin, G. Dubin and M. A. Wettendorff, Vaccine, 2006, 24, 5937–45949 CrossRef CAS PubMed.
- D. M. Harper, Expert Rev. Vaccines, 2009, 8, 1663–1679 CrossRef CAS PubMed.
- Q. He, A. R. Mitchell, S. L. Johnson, C. Wagner-Bartak, T. Morcol and S. J. D. Bell, Clin. Diagn. Lab. Immunol., 2000, 7, 899–903 CAS.
- C. Schellack, K. Prinz, A. Egyed, J. H. Fritz, B. Wittmann, M. Ginzler, G. Swatosch, W. Zauner, C. Kast, S. Akira, A. von Gabain, M. Buschle and K. Lingnau, Vaccine, 2006, 24, 5461–5472 CrossRef CAS PubMed.
- C. Aagaard, T. T. Hoang, A. Izzo, R. Billeskov, J. Troudt, K. Arnett, A. Keyser, T. Elvang, P. Andersen and J. Dietrich, PLoS One, 2009, 4, e5930 Search PubMed.
- A. M. Hafner, B. Corthésy and H. P. Merkle, Adv. Drug Delivery Rev., 2013, 65, 1386–1399 CrossRef CAS PubMed.
- T. Knuschke, M. Epple and A. M. Westendorf, Hum. Vaccines Immunother., 2014, 10, 164–169 CrossRef CAS PubMed.
- G. Reid, Vaccine, 1992, 10, 597–602 CrossRef CAS.
- K. Morris, Lancet Infect. Dis., 2013, 13, 396–397 CrossRef.
- M. E. Marohn and E. M. Barry, Vaccine, 2013, 3485–3491 CrossRef CAS PubMed.
- P. Verdijk, N. Y. Rots, M. G. van Oijen, M. S. Oberste, C. J. Boog, H. Okayasu, R. W. Sutter and W. A. Bakker, Vaccine, 2013, 31, 5531–5536 CrossRef CAS PubMed.
- P. Riese, K. Schulze, T. Ebensen, B. Prochnow and C. A. Guzmán, Curr. Top. Med. Chem., 2013, 13, 2562–2580 CrossRef CAS.
- R. Mazid, X. M. Tan and K. M. Danquah, Curr. Pharm. Biotechnol., 2013, 14, 615–622 CAS.
- A. E. Gregory, R. Titball and D. Williamson, Front. Cell. Infect. Microbiol., 2013, 25, 1–13 Search PubMed.
- P. Marrack, A. S. McKee and M. W. Munks, Nat. Rev. Immunol., 2009, 9, 287–293 CrossRef CAS PubMed.
- T. F. Schwarz, T. Horacek, M. Knuf, H. G. Damman, F. Roman, M. Drame, P. Gillard and W. Jilg, Vaccine, 2009, 27, 6284–6290 CrossRef CAS PubMed.
- A. M. Didierlaurent, S. Morel, L. Lockman, S. L. Giannini, M. Bisteau, H. Carlsen, A. Kielland, O. Vosters, N. Vanderheyde, F. Schiavetti, D. Larocque, M. Van Mechelen and N. Garçon, J. Immunol., 2009, 183, 6186–6197 CrossRef CAS PubMed.
- T. Vesikari, N. Groth, A. Karvonen, A. Borkowski and M. Pellegrini, Vaccine, 2009, 27, 6291–6295 CrossRef CAS PubMed.
- D. T. O'Hagan, G. S. Ott, G. V. Nest, R. Rappuoli and G. D. Giudice, Expert Rev. Vaccines, 2013, 1, 13–30 CrossRef PubMed.
- S. M. Standley, I. Mende, S. L. Goh, Y. J. Kwon, T. T. Beaudette, E. G. Engleman and J. M. Fréchet, Bioconjugate Chem., 2007, 18, 77–83 CrossRef CAS PubMed.
- D. M. Klinman, S. Klaschik, T. Sato and D. Tross, Adv. Drug Delivery Rev., 2009, 61, 248–255 CrossRef CAS PubMed.
- C. Bode, G. Zhao, F. Steinhagen, T. Kinjo and D. M. Klinman, Expert Rev. Vaccines, 2011, 4, 499–511 CrossRef PubMed.
- H. Yan, M. E. Lamm, E. Björling and Y. T. Huang, J. Virol., 2002, 76, 10972–10979 CrossRef CAS.
- A. N. Honko and S. B. Mizel, Immunol. Res., 2005, 33, 83–101 CrossRef CAS PubMed.
- W. Shi, Y. H. Li, F. Liu, J. Y. Yang, D. H. Zhou, Y. Q. Chen, Y. Zhang, Y. Yang, B. X. He, C. Han, M. W. Fan and H. M. Yan, J. Dent. Res., 2012, 91, 249–254 CrossRef CAS PubMed.
- D. Kozlova, V. Sokolova, M. Zhong, E. Zhang, J. Yang, W. Li, Y. Yang, J. Buer, A. M. Westendorf, M. Epple and H. Yan, Virol. Sin., 2014, 29, 1–7 CrossRef PubMed.
- T. A. Olafsdottir, K. Lingnau, E. Nagy and I. Jonsdottir, Scand. J. Immunol., 2009, 3, 69194–69202 Search PubMed.
- K. Tewari, B. J. Flynn, S. B. Boscardin, K. Kastenmueller, A. M. Salazar, C. A. Anderson, V. Soundarapandian, A. Ahumada, T. Keler, S. L. Hoffman, M. C. Nussenzweig, R. M. Steinman and R. A. Seder, Vaccine, 2010, 45, 7256–7266 CrossRef PubMed.
- P. L. Alonso, J. Sacarlal, J. J. Aponte, A. Leach, E. Macete, J. Milman, I. Mandomando, B. Spiessens, C. Guinovart, M. Espasa, Q. Bassat, P. Aide, O. Ofori-Anyinam, M. M. Navia, S. Corachan, M. Ceuppens, M. C. Dubois, M. A. Demoitié, F. Dubovsky, C. Menéndez, N. Tornieporth, W. R. Ballou, R. Thompson and J. Cohen, Lancet, 2004, 364, 1411–1420 CrossRef CAS.
- H. X. Sun, Y. Xie and Y. P. Ye, Vaccine, 2009, 12, 1787–1796 CrossRef PubMed.
- M. Vajdy, I. Srivastava, J. Polo, J. Donnelly, D. O'Hagan and M. Singh, Immunol. Cell Biol., 2004, 82, 617–627 CrossRef CAS PubMed.
- J. P. Kraehenbuhl and M. R. Neutra, Curr. Top. Med. Chem., 2013, 13, 2609–2628 CrossRef CAS.
- A. Shahiwala, T. K. Vyas and M. M. Amiji, Recent Pat. Drug Delivery Formulation, 2007, 1, 1–9 CrossRef CAS.
- A. V. Li, J. J. Moon, W. Abraham, H. Suh, J. Elkhader, M. A. Seidman, M. Yen, E. J. Im, M. H. Foley, D. H. Barouch and D. J. Irvine, Sci. Transl. Med., 2013, 5, 204ra130 Search PubMed.
- M. Rodriguez-Garcia, M. V. Patel and C. R. Wira, J. Reprod. Immunol., 2013, 97, 74–84 CrossRef CAS PubMed.
- P. C. Demuth, W. F. Garcia-Beltran, M. L. Ai-Ling, P. T. Hammond and D. J. Irvine, Adv. Funct. Mater., 2013, 23, 161–172 CrossRef CAS PubMed.
- E. L. Johanssona, C. Bergquista, A. Edebob, C. Johanssona and A. M. Svennerholm, Vaccine, 2004, 22, 984–990 CrossRef PubMed.
- J. M. Silva, M. Videira, R. Gaspar, V. Préat and H. F. Florindo, J. Controlled Release, 2013, 168, 179–199 CrossRef CAS PubMed.
- L. J. Peek, C. R. Middaugh and C. Berkland, Adv. Drug Delivery Rev., 2008, 60, 915–928 CrossRef CAS PubMed.
- L. Shang, S. J. Dong and G. U. Nienhaus, Nano Today, 2011, 6, 401–418 CrossRef CAS PubMed.
- D. A. Kuhn, D. Vanhecke, B. Michen, F. Blank, P. Gehr, A. Petri-Fink and B. Rothen-Rutishauser, Beilstein J. Nanotechnol., 2014, 5, 1625–1636 CrossRef CAS PubMed.
- L. Yang, L. Shang and G. U. Nienhaus, Nanoscale, 2013, 5, 1537–1543 RSC.
- I. Canton and G. Battaglia, Chem. Soc. Rev., 2012, 41, 2718–2739 RSC.
- G. Sahay, D. Y. Alakhova and A. V. Kabanov, J. Controlled Release, 2010, 145, 182–195 CrossRef CAS PubMed.
- H. Goesmann and C. Feldmann, Angew. Chem., Int. Ed., 2010, 49, 1362–1395 CrossRef CAS PubMed.
- D. A. Giljohann, D. S. Seferos, W. L. Daniel, M. D. Massich, P. C. Patel and C. A. Mirkin, Angew. Chem., Int. Ed., 2010, 49, 3280–3294 CrossRef CAS PubMed.
- V. Sokolova and M. Epple, Angew. Chem., Int. Ed., 2008, 47, 1382–1395 CrossRef CAS PubMed.
- M. Ferrari, Trends Biotechnol., 2010, 28, 181–188 CrossRef CAS PubMed.
- A. Saupe, W. McBurney, T. Rades and S. Hook, Expert Opin. Drug Delivery, 2006, 3, 345–354 CrossRef CAS PubMed.
- E. Schlosser, M. Mueller, S. Fischer, S. Basta, D. H. Busch, B. Gander and M. Groettrup, Vaccine, 2008, 26, 1626–1637 CrossRef CAS PubMed.
- T. Akagi, M. Baba and M. Akashi, Adv. Polym. Sci., 2012, 247, 31–64 CAS.
- G. L. Verdine and L. D. Walensky, Clin. Cancer Res., 2007, 13, 7264–7270 CrossRef CAS PubMed.
- T. G. Iversen, T. Skotland and K. Sandvig, Nano Today, 2011, 6, 176–185 CrossRef CAS PubMed.
- F. S. Quan, C. Huang, R. W. Compans and S. M. Kang, J. Virol., 2007, 81, 3514–3524 CrossRef CAS PubMed.
- W. Zhao, W. Wu and X. Xu, Vaccine, 2007, 25, 7664–7673 CrossRef CAS PubMed.
- L. F. Fries, D. M. Gordon, R.
L. Richards, J. E. Egan, M. R. Hollingdale, M. Gross, C. Silverman and C. R. Alving, Proc. Natl. Acad. Sci. U. S. A., 1992, 89, 358–362 CrossRef CAS.
- M. Tafaghodi, M. R. Jaafari and S. A. Sajadi Tabassi, Eur. J. Pharm. Biopharm., 2006, 64, 138–144 CrossRef CAS PubMed.
- T. Musumeci, C. A. Ventura, I. Giannone, B. Ruozi, L. Montenegro, R. Pignatello and G. Puglisi, Int. J. Pharm., 2006, 325, 172–179 CrossRef CAS PubMed.
- S. Amselem, C. R. Alving and A. J. Domb, Polym. Adv. Technol., 1992, 3, 351–357 CrossRef CAS PubMed.
- R. J. Verheul, B. Slütter, S. M. Bal, J. A. Bouwstra, W. Jiskoot and W. E. Hennink, J. Controlled Release, 2011, 156, 46–52 CrossRef CAS PubMed.
- T. Knuschke, V. Sokolova, O. Rotan, M. Wadwa, M. Tenbusch, W. Hansen, P. Staeheli, M. Epple, J. Buer and A. M. Westendorf, J. Immunol., 2013, 190, 6221–6229 CrossRef CAS PubMed.
- X. Zhou, X. Zhang, X. Yu, X. Zha, Q. Fu, B. Liu, X. Wang, Y. Chen, Y. Chen, Y. Shan, Y. Jin, Y. Wu, J. Liu, W. Kong and J. Shen, Biomaterials, 2008, 29, 111–117 CrossRef CAS PubMed.
- W. Tao, K. S. Ziemer and H. S. Gill, Nanomedicine, 2013, 9, 237–251 CrossRef PubMed.
- H. C. Guo, X. M. Feng, S. Q. Sun, Y. Q. Wei, D. H. Sun, X. T. Liu, Z. X. Liu, J. X. Luo and H. Yin, Virol. J., 2012, 9, 108 CrossRef CAS PubMed.
- A. Roldão, M. C. Mellado, L. R. Castilho, M. J. Carrondo and P. M. Alves, Expert Rev. Vaccines, 2010, 9, 1149–1176 CrossRef PubMed.
- M. Kanekiyo, C. J. Wei, H. M. Yassine, P. M. McTamney, J. C. Boyington, J. R. R. Whittle, S. S. Rao, W. P. Kong, L. Wang and G. J. Nabel, Nature, 2013, 499, 102–106 CrossRef CAS PubMed.
- E. V. Grgacic and D. A. Anderson, Methods, 2006, 40, 60–65 CrossRef CAS PubMed.
- B. Mengiardi, R. Berger, M. Just and R. Glück, Vaccine, 1995, 13, 1306–1315 CrossRef CAS.
- E. Strable and M. G. Finn, Curr. Top. Microbiol. Immunol., 2009, 327, 1–21 CAS.
- K. G. Patel and J. R. Swartz, Bioconjugate Chem., 2011, 3, 376–387 CrossRef PubMed.
- S. M. Goldinger, R. Dummer, P. Baumgaertner, D. Mihic-Probst, K. Schwarz, A. Hammann-Haenni, J. Willers, C. Geldhof, J. O. Prior, T. M. Kündig, O. Michielin, M. F. Bachmann and D. E. Speiser, Eur. J. Immunol., 2012, 42, 3049–3061 CrossRef CAS PubMed.
- V. Juarez, H. A. Pasolli, A. Hellwig, N. Garbi and A. C. Arregui, Open Virol. J., 2012, 6, 270–276 CrossRef PubMed.
- T. G. Evans, W. Bonnez, R. C. Rose, S. Koenig, L. Demeter, J. A. Suzich, D. O'Brien, M. Campbell, W. I. White, J. Balsley and R. C. Reichman, J. Infect. Dis., 2001, 183, 1485–1493 CrossRef CAS PubMed.
- S. E. Olsson, L. L. Villa, R. L. Costa, C. A. Petta, R. P. Andrade, C. Malm, O. E. Iversen, J. Høye, M. Steinwall, G. Riis-Johannessen, A. Andersson-Ellstrom, K. Elfgren, G. von Krogh, M. Lehtinen, J. Paavonen, G. M. Tamms, K. Giacoletti, L. Lupinacci, M. T. Esser, S. C. Vuocolo, A. J. Saah and E. Barr, Vaccine, 2007, 25, 4931–4939 CrossRef CAS PubMed.
- C. Moser, M. Amacker, A. R. Kammer, S. Rasi, N. Westerfeld and R. Zurbriggen, Expert Rev. Vaccines, 2007, 6, 711–721 CrossRef CAS PubMed.
- P. Pushko, M. B. Pearce, A. Ahmad, I. Tretyakova, G. Smith, J. A. Belser and T. M. Tumpey, Vaccine, 2011, 29, 5911–5918 CrossRef CAS PubMed.
- K. R. Young, S. P. McBurney, L. U. Karkhanis and T. M. Ross, Methods, 2006, 40, 98–117 CrossRef CAS PubMed.
- G. Sailaja, I. Skountzou, F. S. Quan, R. W. Compans and S. M. Kang, Virology, 2007, 362, 331–341 CrossRef CAS PubMed.
- M. Henriksen-Lacey, K. S. Korsholm, P. Andersen, Y. Perrie and D. Christensen, Expert Opin. Drug Delivery, 2011, 8, 505–519 CrossRef CAS PubMed.
- M. L. Burke, M. D. Veer, J. Pleasance, M. Neeland, M. Elhay, P. Harrison and E. Meeusen, Innate Immun., 2013, 5, 501–510 Search PubMed.
- H. Mishra, D. Mishra, P. K. Mishra, M. Nahar, V. Dubey and N. K. Jain, J. Pharm. Pharm. Sci., 2010, 13, 495–509 CAS.
- M. E. Baca-Estrada, M. M. Foldvari, M. M. Snider, K. K. Harding, B. B. Kournikakis, L. A. Babiuk and P. P. Griebel, Vaccine, 2000, 18, 2203–2211 CrossRef CAS.
- B. Heurtault, B. Frisch and F. Pons, Expert Opin. Drug Delivery, 2010, 7, 829–844 CrossRef CAS PubMed.
- T. Storni, T. M. Kündig, G. Senti and P. Johansen, Adv. Drug Delivery Rev., 2005, 57, 333–355 CrossRef CAS PubMed.
- A. Caputo, A. Castaldello, E. Brocca-Cofano, R. Voltan, F. Bortolazzi, G. Altavilla, K. Sparnacci, M. Laus, L. Tondelli, R. Gavioli and B. Ensoli, Vaccine, 2009, 27, 3605–3615 CrossRef CAS PubMed.
- T. Uto, T. Akagi, T. Hamasaki, M. Akashi and M. Baba, Immunol. Lett., 2009, 125, 46–52 CrossRef CAS PubMed.
- T. Uto, T. Akagi, M. Toyama, Y. Nishi, F. Shima, M. Akashi and M. Baba, Immunol. Lett., 2011, 140, 36–43 CrossRef CAS PubMed.
- H. F. Florindo, S. Pandit, L. M. Gonçalves, M. Videira, O. Alpar and A. J. Almeida, Biomaterials, 2009, 30, 5161–5169 CrossRef CAS PubMed.
- B. N. Fredriksen and J. Grip, Vaccine, 2012, 30, 656–667 CrossRef CAS PubMed.
- G. Dordelmann, D. Kozlova, S. Karczewski, R. Lizio, S. K. Knauer and M. Epple, J. Mater. Chem. B, 2014, 2, 7250–7259 RSC.
- P. A. Sales-Junior, F. Guzman, M. I. Vargas, S. Sossai, V. A. M. Patarroyo, C. Z. González and J. H. Patarroyo, Vet. Immunol. Immunopathol., 2005, 107, 281–290 CrossRef CAS PubMed.
- L. Feng, X. R. Qi, X. J. Zhou, Y. Maitani, S. C. Wang, Y. Jiang and T. Nagai, J. Controlled Release, 2006, 112, 35–42 CrossRef CAS PubMed.
- S. Fischer, E. Schlosser, M. Mueller, N. Csaba, H. P. Merkle, M. Groettrup and B. Gander, J. Drug Targeting, 2009, 17, 652–661 CrossRef CAS PubMed.
- W. Ma, M. Chen, S. Kaushal, M. McElroy, Y. Zhang, C. Ozkan, M. Bouvet, C. Kruse, D. Grotjahn, T. Ichim and B. Minev, Int. J. Nanomed., 2012, 7, 1475–1487 CrossRef CAS PubMed.
- G. Kaiser-Schulz, A. Heit, L. Quintanilla-Martinez, F. Hammerschmidt, S. Hess, L. Jennen, H. Rezaei and H. Wagner, J. Immunol., 2007, 179, 2797–2807 CrossRef CAS.
- B. San Román, J. M. Irache, S. Gómez, N. Tsapis, C. Gamazo and M. S. Espuelas, Eur. J. Pharm. Biopharm., 2008, 70, 98–108 CrossRef PubMed.
- S. L. Demento, S. C. Eisenbarth, H. G. Foellmer, C. Platt, M. J. Caplan, M. W. Saltzman, I. Mellman, M. Ledizet, E. Fikrig, R. A. Flavell and T. M. Fahmy, Vaccine, 2009, 27, 3013–3021 CrossRef CAS PubMed.
- D. A. Edwards, J. Hanes, G. Caponetti, J. Hrkach, A. Ben-Jebria, M. L. Eskew, J. Mintzes, D. Deaver, N. Lotan and R. Langer, Science, 1997, 276, 1868–1871 CrossRef CAS.
- S. Prabha, W. Z. Zhou and V. Labhasetwar, Int. J. Pharm., 2002, 244, 105–115 CrossRef CAS.
- Y. N. Konan, J. Chevallier, R. Gurny and E. Allemann, Photochem. Photobiol., 2003, 77, 638–644 CrossRef CAS.
- L. N. Luong, K. M. McFalls and D. H. Kohn, Biomaterials, 2009, 30, 6996–7004 CrossRef CAS PubMed.
- C. Wischke, J. Zimmermann, B. Wessinger, A. Schendler, H. H. Borchert, J. H. Peters, T. Nesselhut and D. R. Lorenzen, Int. J. Pharm., 2009, 1–2, 61–68 CrossRef PubMed.
- S. Shi and A. J. Hickey, Pharm. Res., 2010, 27, 350–360 CrossRef CAS PubMed.
- M. Manish, A. Rahi, M. Kaur, R. Bhatnagar and S. Singh, PLoS One, 2013, 8, e61885 CAS.
- D. J. Bharali, V. Pradhan, G. Elkin, W. Qi, A. Hutson, S. A. Mousa and Y. Thanavala, Nanomedicine, 2008, 4, 311–317 CrossRef CAS PubMed.
- S. L. Demento, W. Cui, J. M. Criscione, E. Stern, J. Tulipan, S. M. Kaech and T. M. Fahmy, Biomaterials, 2012, 33, 4957–4964 CrossRef CAS PubMed.
- K. Tappertzhofen, M. Bednarczyk, K. Koynov, M. Bros, S. Grabbe and R. Zentel, Macromol. Biosci., 2014, 14, 1444–1457 CrossRef CAS PubMed.
- J. Bühler, S. Gietzen, A. Reuter, C. Kappel, K. Fischer, S. Decker, D. Schäffel, K. Koynov, M. Bros, I. Tubbe, S. Grabbe and M. Schmidt, Chem. – Eur. J., 2014, 20, 12405–12410 CrossRef PubMed.
- B. Dineshkumar, S. A. Dhanaraj, K. Santhi, P. Vijayan and R. Chandrasekhar, Int. J. Adv. Pharm. Sci., 2010, 1, 42–49 CrossRef CAS.
- I. Jabbal-Gill, P. Watts and A. Smith, Expert Opin. Drug Delivery, 2012, 1051–1067 CrossRef CAS PubMed.
- K. Sonaje, E. Y. Chuang, K. J. Lin, T. C. Yen, F. Y. Su, M. T. Tseng and H. W. Sung, Mol. Pharmaceutics, 2012, 9, 1271–1279 CAS.
- S. Varma and C. Sadasivan, Biomed. Pharmacother., 2013, 68, 225–230 CrossRef PubMed.
- P. G. Seferian and M. L. Martinez, Vaccine, 2000, 19, 661–668 CrossRef CAS.
- L. Illum, I. Jabbal-Gill, M. Hinchcliffe, A. N. Fisher and S. S. Davis, Adv. Drug Delivery Rev., 2001, 51, 81–96 CrossRef CAS.
- E. M. Bachelder, T. T. Beaudette, K. E. Broaders, S. E. Paramonov, J. Dashe and J. M. Fréchet, Mol. Pharmaceutics, 2008, 5, 876–884 CrossRef CAS PubMed.
- S. L. Demento, N. Bonafé, W. Cui, S. M. Kaech, M. J. Caplan, E. Fikrig, M. Ledizet and T. M. Fahmy, J. Immunol., 2010, 185, 2989–2997 CrossRef CAS PubMed.
- W. Tai, L. Roberts, A. Seryshev, J. M. Gubatan, C. S. Bland, R. Zabriskie, S. Kulkarni, L. Soong, I. Mbawuike, B. Gilbert, F. Kheradmand and D. B. Corry, Mucosal Immunol., 2011, 4, 197–207 CrossRef CAS PubMed.
- R. Sardar, A. M. Funston, P. Mulvaney and R. W. Murray, Langmuir, 2009, 25, 13840–13851 CrossRef CAS PubMed.
- X. Huang, S. Neretina and M. A. El-Sayed, Adv. Mater., 2009, 21, 4880–4910 CrossRef CAS PubMed.
- R. A. Sperling, P. Rivera, F. Zhang, M. Zanella and W. J. Parak, Chem. Soc. Rev., 2008, 37, 1896–1908 RSC.
- N. L. Rosi, D. A. Giljohann, C. S. Thaxton, A. K. R. Lytton-Jean, M. S. Han and C. A. Mirkin, Science, 2006, 312, 1027–1030 CrossRef CAS PubMed.
- Y. Huang, F. Yu, Y. S. Park, J. Wang, M. C. Shin, H. S. Chung and V. C. Yang, Biomaterials, 2010, 34, 9086–9091 CrossRef PubMed.
- R. Bhattacharya, C. R. Patra, R. Verma, S. Kumar, P. R. Greipp and P. Mukherjee, Adv. Mater., 2007, 19, 711–716 CrossRef CAS PubMed.
- P. Baptista, E. Pereira, P. Eaton, G. Doria, A. Miranda, I. Gomes, P. Quaresma and R. Franco, Anal. Bioanal. Chem., 2008, 391, 943–950 CrossRef CAS PubMed.
- A. Maitra, Expert Rev. Mol. Diagn., 2005, 5, 893–905 CrossRef CAS PubMed.
- V. Uskokovic and D. P. Uskokovic, J. Biomed. Mater. Res., Part B, 2011, 96, 152–191 CrossRef PubMed.
- M. Epple, K. Ganesan, R. Heumann, J. Klesing, A. Kovtun, S. Neumann and V. Sokolova, J. Mater. Chem., 2010, 20, 18–23 RSC.
- Y. Cai and R. Tang, J. Mater. Chem., 2008, 18, 3775–3787 RSC.
- O. Rotan, V. Sokolova, P. Gilles, W. Hu, S. Dutt, T. Schrader and M. Epple, Materialwiss. Werkstofftech., 2013, 44, 176–182 CrossRef CAS PubMed.
- V. Sokolova, O. Rotan, J. Klesing, P. Nalbant, J. Buer, T. Knuschke, A. M. Westendorf and M. Epple, J. Nanopart. Res., 2012, 14, 910 CrossRef.
- V. V. Sokolova, I. Radtke, R. Heumann and M. Epple, Biomaterials, 2006, 27, 3147–3153 CrossRef CAS PubMed.
- D. Kozlova, S. Chernousova, T. Knuschke, J. Buer, A. M. Westendorf and M. Epple, J. Mater. Chem., 2012, 22, 396–404 RSC.
- S. Neumann, A. Kovtun, I. D. Dietzel, M. Epple and R. Heumann, Biomaterials, 2009, 30, 6794–6802 CrossRef CAS PubMed.
- V. Sokolova, D. Kozlova, T. Knuschke, J. Buer, A. M. Westendorf and M. Epple, Acta Biomater., 2013, 9, 7527–7535 CrossRef CAS PubMed.
- Q. He, A. Mitchell, T. Morcol and S. J. D. Bell, Clin. Diagn. Lab. Immunol., 2002, 9, 1021–1024 CAS.
- V. Sokolova, T. Knuschke, A. Kovtun, J. Buer, M. Epple and A. M. Westendorf, Biomaterials, 2010, 31, 5627–5633 CrossRef CAS PubMed.
- V. Sokolova, T. Knuschke, J. Buer, A. M. Westendorf and M. Epple, Acta Biomater., 2011, 7, 4029–4036 CrossRef CAS PubMed.
- V. Temchura, D. Kozlova, V. Sokolova, K. Überla and M. Epple, Biomaterials, 2014, 35, 6098–6105 CrossRef CAS PubMed.
- P. Sahdev, S. Podaralla, R. S. Kaushik and O. Perumal, J. Biomed. Nanotechnol., 2013, 1, 132–141 CrossRef PubMed.
- T. Knuschke, W. Bayer, O. Rotan, V. Sokolova, M. Wadwa, C. J. Kirschning, W. Hansen, U. Dittmer, M. Epple, J. Buer and A. M. Westendorf, Nanomedicine, 2014, 10, 1787–1798 CrossRef CAS PubMed.
- L. Treccani, T. Y. Klein, F. Meder, K. Pardun and K. Rezwan, Acta Biomater., 2013, 9, 7115–7150 CrossRef CAS PubMed.
- J. M. Rosenholm, C. Sahlgren and M. Linden, Nanoscale, 2010, 2, 1870–1883 RSC.
- M. Vallet-Regi, F. Balas and D. Arcos, Angew. Chem., Int. Ed., 2007, 46, 7548–7558 CrossRef CAS PubMed.
- A. Awaad, M. Nakamura and K. Ishimura, Int. J. Nanomed., 2012, 7, 1423–1439 CAS.
- Y. N. Xia, Y. Xiong, B. Lim and S. E. Skrabalak, Angew. Chem., Int. Ed., 2009, 48, 60–103 CrossRef CAS PubMed.
- J. Kim, W. A. Li, Y. Choi, S. A. Lewin, C. S. Verbeke, G. Dranoff and D. J. Mooney, Nat. Biotechnol., 2015, 33, 64–72 CrossRef CAS PubMed.
- S. K. Lai, K. Hida, S. T. Man, C. Chen, C. Machamer, T. A. Schroer and J. Hanes, Biomaterials, 2007, 28, 2876–2884 CrossRef CAS PubMed.
- M. Nazarenus, Q. Zhang, M. G. Soliman, P. del Pino, B. Pelaz, S. Carregal-Romero, J. Rejman, B. Rothen-Rutishauser, M. J. D. Clift, R. Zellner, G. U. Nienhaus, J. B. Delehanty, I. L. Medintz and W. J. Parak, Beilstein J. Nanotechnol., 2014, 5, 1477–1490 CrossRef PubMed.
- L. Treuel, C. Jiang and G. U. Nienhaus, J. R. Soc., Interface, 2013, 10, 20120939 CrossRef PubMed.
- C. Foged, B. Brodin, S. Frokjaer and A. Sundblad, Int. J. Pharm., 2005, 2, 315–322 CrossRef PubMed.
- I. Gutierro, R. M. Hernández, M. Igartua, A. R. Gascón and J. L. Pedraz, Vaccine, 2002, 1–2, 67–77 CrossRef.
- J. A. Champion and S. Mitragotri, Proc. Natl. Acad. Sci. U. S. A., 2006, 13, 4930–4934 CrossRef PubMed.
- K. Hirota, T. Hasegawa, H. Hinata, F. Ito, H. Inagawa, C. Kochi, G. Soma, K. Makino and H. Terada, J. Controlled Release, 2007, 119, 69–76 CrossRef CAS PubMed.
- A. Akinc, M. Thomas, A. M. Klibanov and R. S. Langer, J. Gene Med., 2005, 7, 657–663 CrossRef CAS PubMed.
- D. J. Irvine, M. A. Swartz and G. L. Szeto, Nat. Mater., 2013, 12, 978–990 CrossRef CAS PubMed.
- V. Manolova, A. Flace, M. Bauer, K. Schwarz, P. Saudan and M. F. Bachmann, Eur. J. Immunol., 2008, 38, 1404–1413 CrossRef CAS PubMed.
-
V. Sokolova, Synthesis, characterization and application of calcium phosphate nanoparticles for the transfection of cells, PhD thesis, University of Duisburg-Essen, Germany, 2006 Search PubMed.
- K. J. Lee, L. M. Browning, P. D. Nallathamby, T. Desai, P. K. Cherukuri and X. H. N. Xu, Chem. Res. Toxicol., 2012, 25, 1029–1046 CrossRef CAS PubMed.
- C. H. Saroja, P. K. Lakshmi and S. Bhaskaran, Int. J. Pharm. Invest., 2011, 2, 64–74 Search PubMed.
- F. M. Kievit and M. Zhang, Adv. Mater., 2011, 23, H209 CrossRef PubMed.
- A. Bolhassani, S. Safaiyan and S. Rafati, Mol. Cancer, 2011, 10, 3 CrossRef CAS PubMed.
- J. Jardine, J. P. Julien, S. Menis, T. Ota, O. Kalyuzhniy, A. McGuire, D. Sok, P. S. Huang, S. MacPherson, M. Jones, T. Nieusma, J. Mathison, D. Baker, A. B. Ward, D. R. Burton, L. Stamatatos, D. Nemazee, I. A. Wilson and W. R. Schief, Science, 2013, 340, 711–716 CrossRef CAS PubMed.
- L. Zhao, A. Seth, N. Wibowo, C.-X. Zhao, N. Mitter, C. Yu and A. P. J. Middelberg, Vaccine, 2014, 32, 327–337 CrossRef PubMed.
- J. Klesing, S. Chernousova and M. Epple, J. Mater. Chem., 2012, 22, 199–204 RSC.
|
This journal is © The Royal Society of Chemistry 2015 |