Cyclodextrin-functionalized hollow carbon nanospheres by introducing nanogold for enhanced electrochemical sensing of o-dihydroxybenzene and p-dihydroxybenzene†
Received
22nd September 2014
, Accepted 6th November 2014
First published on 11th November 2014
Abstract
In this work, hollow carbon nanospheres (HCNS) were prepared, followed by the introduction of gold nanoparticles (AuNPs) on the HCNS surface, and then functionalization with per-6-thio-β-cyclodextrin (CD) based on the formation of “Au–S” bond, resulting in a novel cyclodextrin/carbon-based nanohybrid (CD–AuNPs/HCNS), which possesses the unique properties of HCNS (excellent electrochemical properties and large surface area), CD (high host–guest recognition and water-solubility) and AuNPs (excellent electrocatalytic activity). The obtained CD–AuNPs/HCNS nanohybrids were characterized by scanning electron microscopy, transmission electron microscopy, inductively coupled plasma-atomic emission spectroscopy, Fourier transform infrared spectroscopy and electrochemical methods. Furthermore, CD–AuNPs/HCNS were applied in the simultaneous electrochemical sensing of o-dihydroxybenzene (o-DHB) and p-dihydroxybenzene (p-DHB) (both o- and p-DHB have similar structures and coexist in environment; moreover, they are toxic to humans and difficult to degrade). Under the optimum conditions, the detection limits of o- and p-DHB obtained in this work are 0.01 and 0.02 μM, respectively.
Introduction
Two types of dihydroxybenzene isomers, o-dihydroxybenzene (o-DHB) and p-dihydroxybenzene (p-DHB), are frequently used as industrial reagents in the production of plastic, leather, paint, cosmetics, dyes, rubber and pharmaceuticals.1–3 However, both o- and p-DHB exist widely in the environment as noteworthy environmental pollutants because they are toxic to humans and difficult to degrade. Owing to their similar structures, properties, and coexistence in environmental samples, the simultaneous determination of o- and p-DHB is very important. To date, many electrochemical sensors have been designed to detect o- and p-DHB.4 Unfortunately, o- and p-DHB usually cannot be distinguished on conventional electrodes because of the overlap of their oxidation–reduction peaks.5 Recently, some interesting works have been developed to resolve this problem,4,6 but highly sensitive simultaneous electrochemical determination of o- and p-DHB is still a great challenge.
Moreover, considerable attention has been paid to hollow nanostructures because of their fascinating properties (e.g., low density, high surface-to-volume ratio, and high shell permeability) and promising applications in a wide range of areas.7 Among the families of hollow nanostructures that are experiencing impressive advances, hollow carbon nanospheres (HCNS, a type of carbon-based material) are interesting because of the exceptional properties of carbon, such as low cost, nontoxicity, good chemical stability and good electrical conductivity, thus HCNS have been applied widely in catalysis,8,9 lithium-ion batteries,10,11 oxygen-reduction,12 supercapacitors13 and water treatment.14,15 However, there are only a few studies on the applications of HCNS in electrochemical sensors. In addition, the unmodified pure HCNS are usually insoluble in water, resulting in the severe restriction of their applications. Therefore, designing or introducing new functional molecules to increase the dispersibility of HCNS while introducing new or enhanced functions for electrochemical sensors is highly desirable and technologically important.
Cyclodextrins (CDs) are oligosaccharides that are composed of six, seven, or eight glucose units (α-, β-, or γ-CD, respectively), which are toroidal in shape with a hydrophobic inner cavity and a hydrophilic exterior.16–18 These interesting characteristics enable CDs to act as solubilizers, which improve the water-solubility of functional materials while enabling the functional materials to bind selectively with a large variety of hydrophobic organic molecules in the cavities of CDs to form stable host–guest inclusion complexes, which show high molecular selectivity and enantioselectivity.19,20 Therefore, if HCNS are modified with CDs and then used in electrochemical sensors, it is possible to obtain a new functionalized material, which would have excellent electrochemical sensor performance for highly sensitive and selective determination of target compounds.
In fact, many attempts, including noncovalent exohedral interactions and covalent sidewall coupling reactions, have been developed to produce CDs/carbon-based (mainly focused on carbon nanotubes and graphene) nanohybrids for obtaining the synergetic effects of CDs (e.g., a hydrophilic external surface and a high supramolecular recognition and enrichment capability) and carbon-based materials (e.g., good electrochemical properties and a large surface area). For noncovalent interactions, a highly aromatic molecule, such as 1-pyreneacetic acid,17,21 3,4,9,10-perylenetetracarboxylic acid,22p-phenylenediamine,23 aniline polypseudorotaxane,24 tris[4,4-bis(4-pyren-1-ylbutyloxy)bipyridinyl)] iron complex25 and adamantine,26 is usually used as a bridge between CDs and carbon-based materials. For covalent reactions, chemical oxygen groups are needed on the surface of the carbon-based materials to react with CDs by esterification,27 sulfonylation28 and many other reactions.29,30 Although the carbon-based materials could be successfully functionalized with CDs by these methods, there are many disadvantages: (i) complex synthesis procedures, (ii) reduction of effective surface area, (iii) loss of electronic conductivity and corrosion resistance, and (iv) structural damage of the nanomaterials. These are not beneficial for enhancing the electrochemical sensor performance of CDs/carbon-based nanohybrids.
It has been reported that dihydroxybenzene isomers could bind to the cavity of β-CD to form a stable host–guest inclusion and that the ability to associate with β-CD varies for different isomers,18 indicating that β-CD could be used for the selective detection of o- and p-DHB. Based on the abovementioned facts, HCNS were prepared herein by the polymerization and carbonization of dopamine hydrochloride (DA) (Scheme S1†). Then, by decorating gold nanoparticles (AuNPs) on HCNS surfaces to form AuNPs/HCNS hybrids, followed by the functionalization of the AuNPs/HCNS with per-6-thio-β-cyclodextrin (CD, Fig. S1†), a novel nanohybrid (denoted as CD–AuNPs/HCNS) was obtained. CD–AuNPs/HCNS showed the synergetic effects of HCNS, CD and AuNPs. Importantly, compared to the previous methods for preparing CDs/carbon-based nanohybrids, the method presented in this study is simple, convenient and low-cost, coupled with the advantage that the effective surface area, electronic conductivity, corrosion resistance, and structural stability of the carbon-based nanohybrids are not considerably reduced or damaged due to the presence of the highly aromatic molecules and hydroxyl groups. The CD–AuNPs/HCNS modified glassy carbon (GC) (CD–AuNPs/HCNS/GC) electrode was applied in the simultaneous electrochemical determination of o- and p-DHB with cyclic voltammetry (CV) and differential pulse voltammetry (DPV). The results show that the electrochemical performance of o- and p-DHB at the CD–AuNPs/HCNS/GC electrode is obviously improved, and low detection limits for o-DHB and p-DHB are achieved. The procedures for preparing CD–AuNPs/HCNS and constructing the electrochemical sensor for o- and p-DHB are shown in Scheme 1.
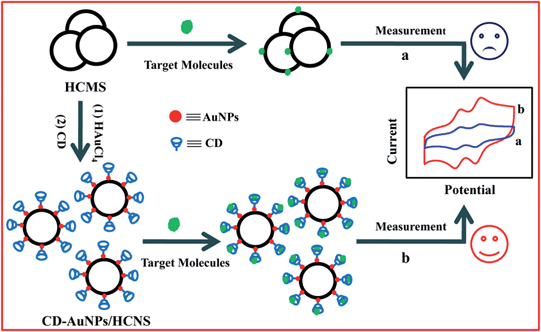 |
| Scheme 1 The process for preparing CD–AuNPs/HCNS and sensing o- and p-DHB by electrochemical method. | |
Experimental section
Reagents and apparatus
o-DHB, p-DHB, DA and SiO2 particles were purchased from Alfa Aesar (USA). CD was purchased from Shandong Binzhou Zhiyuan Bio-Technology Co. Ltd., China. All other chemicals were of analytical grade and directly used without further purification. The supporting electrolyte was 0.1 M phosphate buffer solution (PBS) prepared with Na2HPO4 and NaH2PO4. Aqueous solutions used throughout were prepared with ultra-pure water (>18 MΩ cm) obtained from a Millipore system.
All the electrochemical measurements were performed on a CHI 660E Electrochemical Workstation (Chenhua Instrument Company of Shanghai, China). A conventional three-electrode cell was used with a GC electrode (GC, with a diameter of 3 mm) as the working electrode, a platinum wire as the counter electrode and a Ag/AgCl electrode (saturated KCl) as the reference electrode. Unless specifically stated, the electrochemical measurements were carried out in 0.1 M PBS (pH 7.0) at room temperature (25 ± 2 °C). All the potentials in this paper were in reference to the Ag/AgCl electrode (saturated KCl).
Synthesis of HCNS, AuNPs/HCNS and CD–AuNPs/HCNS
HCNS.
HCNS was prepared according to a previous report.9,14 In brief, SiO2 particles (100.0 mg) were thoroughly washed with tris(hydroxymethyl)aminomethane (TRIS) buffer (50.0 mM; pH = 8.5) and mixed with 40.0 mL of TRIS buffer containing DA (80.0 mg) for 24 h with vigorous stirring. Silica@polydopamine (SiO2@PDA) nanocomposites were obtained by centrifugation and drying in vacuum at 60 °C overnight. For preparing the HCNS, SiO2@PDA nanocomposites were carbonized under N2 atmosphere at 800 °C for 2 h at a heating rate of 5 °C min−1. After washing in 2.0 M HF + 8.0 M NH4F solution for 8 h, the template (SiO2) was removed and HCNS were obtained. Finally, the HCNS were washed and dried.
AuNPs/HCNS.
In an ice-water bath, HCNS (10.0 mg) were dispersed in water (10.0 mL) + ethanol (10.0 mL) for 30 min by sonication, and then hydrogen tetrachloroaurate(III)trihydrate and sodium borohydride solutions were added dropwise. After centrifuging and thoroughly washing with ultra-pure water, AuNPs/HCNS were successfully obtained.
CD–AuNPs/HCNS.
Under sonication, 5.0 mL of CD solution (1.0 mg mL−1) was mixed with 5.0 mL of AuNPs/HCNS suspension (1.0 mg mL−1) for 24 h in the dark. After centrifugation, washing and drying, the CD–AuNPs/HCNS were finally obtained.
Construction of electrochemical sensor for highly sensitive simultaneous determination of o- and p-DHB
The GC electrode was carefully polished to a mirror-like plane with 0.5 and 0.05 μm alumina powder and rinsed with ultra-pure water, followed by sequential sonication in acetone and ultra-pure water. Then, the electrode was allowed to dry under N2 gas. The CD–AuNPs/HCNS/GC electrode was obtained by casting 10.0 μL of CD–AuNPs/HCNS suspension (0.5 mg mL−1) onto the surface of the GC electrode and drying under an infrared lamp. For comparison, HCNS/GC and AuNPs/HCNS/GC electrodes were prepared via a similar procedure.
The simultaneous determination of o- and p-DHB was carried out in 0.1 M PBS, and the determination consisted of two consecutive steps: (1) pre-concentration of target molecules from the solution to the modified electrodes with stirring at a definite accumulation time, and (2) electrochemical measurements by CV and DPV.
Results and discussion
Characterization of CD–AuNPs/HCNS and related materials
Fig. 1 shows the scanning electron microscopy (SEM) images of SiO2 particles (Fig. 1A), SiO2@PDA (Fig. 1B) and HCNS (Fig. 1C). It should be noted that the diameter of SiO2 particles is ∼360 nm and the surface of the particle is very smooth. For SiO2@PDA nanocomposite, the diameter is ∼390 nm, suggesting that the shell thickness of PDA is ∼30 nm. Following the carbonization of SiO2@PDA and removal of SiO2, the HCNS were successfully obtained. The morphology of AuNPs/HCNS was further characterized by transmission electron microscopy (TEM). As shown in Fig. 2, it should be noted that AuNPs were uniformly dispersed on the surface of HCNS, and the particle size of AuNPs is mainly distributed between 2.0 and 7.0 nm, with an average diameter of 4.0 nm (statistically calculated through measuring the diameter of 200 AuNPs). The content of AuNPs was evaluated to be 6.2 wt% by inductively coupled plasma-atomic emission spectroscopy.
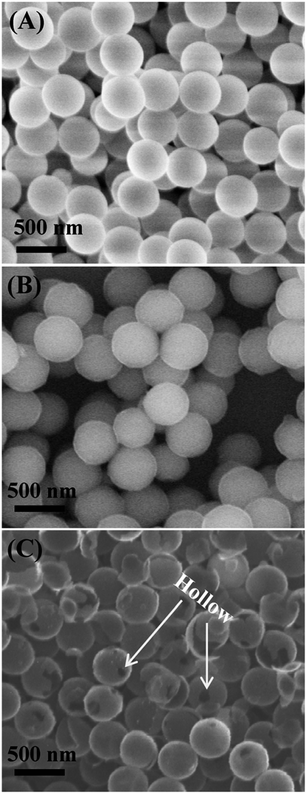 |
| Fig. 1 SEM images of SiO2 (A), SiO2@PDA (B) and HCNS (C). | |
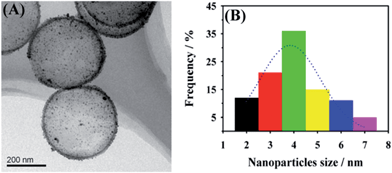 |
| Fig. 2 (A) TEM image of AuNPs/HCNS and (B) the particle size distribution of AuNPs. | |
The functionalization of AuNPs/HCNS with CD was investigated by Fourier transform infrared spectroscopy (FT-IR, Fig. 3A). It should be noted from the curve a that the CD–AuNPs/HCNS exhibit the typical CD absorption features of ring vibrations at 578, 757, and 951 cm−1, the coupled C–O/C–C stretching/O–H bending vibrations at 1025 and 1063 cm−1, the coupled C–O–C stretching/O–H bending vibrations at 1146 cm−1, –SH stretching vibrations at 2512 cm−1, CH2 stretching vibrations at 2910 cm−1, and O–H stretching vibrations at 3421 cm−1. In addition, the images of AuNPs/HCNS and CD–AuNPs/HCNS dispersed in water were evaluated (Fig. 3B). After sonicating for 30 min, the AuNPs/HCNS still could not be dispersed and some black precipitates were observed. However, the CD–AuNPs/HCNS efficiently dissolved in water due to the good water-solubility of CD. These observations suggest that the AuNPs/HCNS were successfully functionalized with CD.
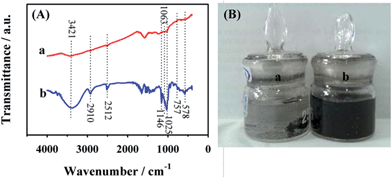 |
| Fig. 3 (A) FT-IR spectra of CD–AuNPs/HCNS (a) and CD (b); (B) images of AuNPs/HCNS (a) and CD–AuNPs/HCNS (b) in water. | |
Electrochemical impedance analysis (EIS) was further used to investigate the electron transfer behavior of CD–AuNPs/HCNS. Fig. 4 shows the EIS results of bare GC (Fig. 4a), AuNPs/HCNS/GC (Fig. 4b) and CD–AuNPs/HCNS/GC (Fig. 4c) electrodes recorded in aqueous solutions of 5.0 mM Fe(CN)63−/4− and 0.1 M KCl. It should be noted that the fitted charge transfer resistance (Rct) value of the AuNPs/HCNS/GC electrode is considerably lower than that of a bare GC electrode, suggesting that the AuNPs/HCNS has high conductivity. For the CD–AuNPs/HCNS/GC electrode, the slight increase in Rct value resulted from the poor electronic conductivity of CD, and the electron conductivity of the CD–AuNPs/HCNS (2.2 S cm−1) measured via the four-point method is lower than that of AuNPs/HCNS (2.9 S cm−1), implying that the AuNPs/HCNS were successfully functionalized with CD.
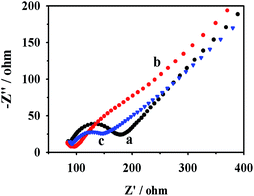 |
| Fig. 4 EIS plots of the bare GC (a), AuNPs/HCNS/GC (b) and CD–AuNPs/HCNS/GC (c) electrodes in an aqueous solution of 5.0 mM Fe(CN)63−/4− + 0.1 M KCl. | |
Electrochemical behaviors of o-DHB and p-DHB
The electrochemical redox behaviors of 10.00 μM o-DHB and 10.00 μM p-DHB at bare GC, HCNS/GC, AuNPs/HCNS/GC and CD–AuNPs/HCNS/GC electrodes were investigated in 0.1 M PBS by CV (Fig. 5). From Fig. 5a, it should be noted that a broad oxidation peak was observed at ∼0.224 V on the GC electrode due to the overlap of the oxidation peaks of o- and p-DHB, and two weak reduction peaks were observed at ∼0.152 V and 0.039 V, corresponding to the reduction of the oxidation products of o- and p-DHB, respectively. At the HCNS/GC electrode, both o- and p-DHB exhibit obvious redox peak currents, and the peak separation is up to 0.110 V (oxidation peaks at ∼0.210 V for o-DHB and 0.100 V for p-DHB). In addition, two well-defined reduction peaks were observed. The redox currents of o- and p-DHB at the HCNS/GC electrode are considerably higher than those at GC electrode. As for the values of peak current on AuNPs/HCNS/GC electrode, they are further apart compared with those on the HCNS/GC and GC electrodes. These observations indicate that both HCNS and AuNPs have excellent electrocatalytic properties towards o- and p-DHB. After the AuNPs/HCNS were functionalized with CD, the peak currents of o- and p-DHB at the CD–AuNPs/HCNS/GC electrode were remarkably enhanced and outperformed all the other modified electrodes because of the synergistic effects from HCNS (excellent electrochemical properties and large surface area), CD (high host–guest recognition and water-solubility) and AuNPs (excellent electrocatalytic activity). In particular, CD could not only increase the dispersibility of HCNS and enhance the utilization of HCNS-based composites, but also increased the accumulation of analytes on the surface of the modified electrodes; thus, the sensitivity of CD–AuNPs/HCNS/GC electrode is better than that of AuNPs/HCNS/GC electrode. These observations suggest that the CD–AuNPs/HCNS nanohybrids have excellent electrochemical sensing capabilities toward dihydroxybenzene isomers. The reaction mechanisms of o- and p-DHB on the modified electrodes are shown in Scheme 2.
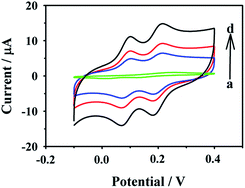 |
| Fig. 5 Voltammetric responses of 10.00 μM o-DHB and 10.00 μM p-DHB at the bare GC (a), HCNS/GC (b), AuNPs/HCNS/GC (c) and CD–AuNPs/HCNS/GC electrodes (d) in 0.1 M PBS. | |
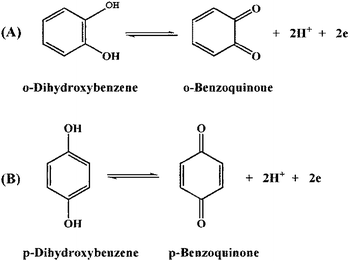 |
| Scheme 2 The mechanisms of the electrochemical oxidations of o-DHB (A) and p-DHB (B). | |
Optimization of the conditions for the simultaneous electrochemical determination of o-DHB and p-DHB
As a highly sensitive electrochemical method, the DPV has considerable higher current sensitivity and better resolution compared with those of CV. Therefore, the condition optimization and simultaneous electrochemical detection of o- and p-DHB using the CD–AuNPs/HCNS/GC electrode were investigated by DPV.
The effects of pH of PBS, amount of CD–AuNPs/HCNS and accumulation time on the oxidation peak current response to o- and p-DHB were evaluated by measuring the DPV responses of 1.00 μM o-DHB and 1.00 μM p-DHB (Fig. 6). It should be noted that the peak currents of both o- and p-DHB gradually increase over the pH range from 5.5 to 7.0, while the oxidation current rapidly decrease after a pH value of 7.0. Therefore, PBS with pH value of 7.0 was selected as the supporting electrolyte.
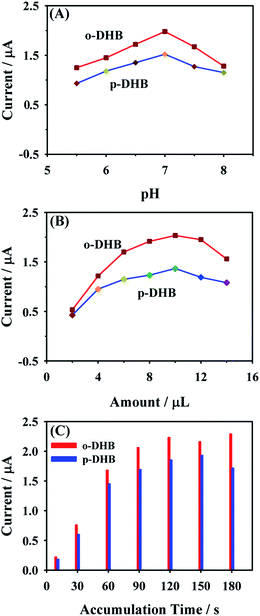 |
| Fig. 6 The effect of the pH value of PBS (A), the amount of CD–AuNPs/HCNS (B) and accumulation time (C) on the DPV peak currents of 1.00 μM o-DHB and 1.00 μM p-DHB at the CD–AuNPs/HCNS/GC electrode in 0.1 M PBS. | |
Fig. 6B shows that the oxidation peak currents of o- and p-DHB increase with the increase in the amount of CD–AuNPs/HCNS, reaching a maximum at 10.0 μL, and when the amount of CD–AuNPs/HCNS further increases, the peak currents clearly decrease. Therefore, this optimum amount (10.0 μL) was used throughout this study unless otherwise specified.
Undoubtedly, the sensitivity of the proposed method is affected by the accumulation time. From Fig. 6C, it should be noted that the oxidation peak currents of o- and p-DHB increase with increase in accumulation time, and the maximum currents are observed at 120 s; thus, this time is selected as the optimum accumulation time in this study.
Simultaneous determination of o-DHB and p-DHB
Under the optimum conditions, the CD–AuNPs/HCNS/GC electrode was used to simultaneously detect o- and p-DHB with DPV. In a binary mixture of o- and p-DHB, the concentration of one species was changed, while that of other was maintained constant. The DPV responses for the different concentrations of o- and p-DHB are shown in Fig. 7. From Fig. 7A, it was found that the oxidation peak currents of o-DHB linearly increase with increase in o-DHB concentration from 0.05 to 6.00 μM, and the corresponding linear function is Ip (μA) = 0.575 + 1.413C (μM) (R2 = 0.9974). Similarly, the oxidation peak currents of p-DHB increase with increase in p-DHB concentration (Fig. 7B), and the linear range is 0.05–6.00 μM with the linear function of Ip (μA) = 0.1773 + 1.0561C (μM) (R2 = 0.9933). The detection limits for o- and p-DHB are 0.01 and 0.02 μM, respectively, which are considerably lower than those obtained by the previously reported electrochemical methods (Table 1). These results suggest that the proposed method in this work is promising for a highly sensitive simultaneous determination of o- and p-DHB.
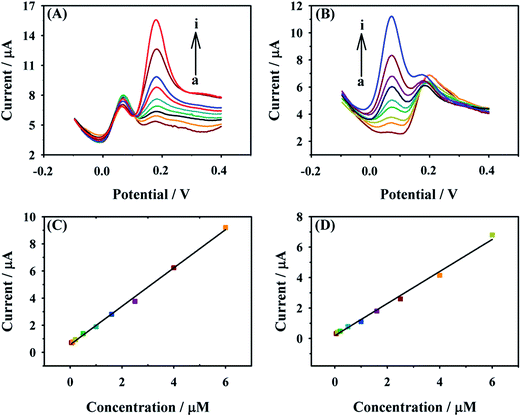 |
| Fig. 7 DPV responses at the CD–AuNPs/HCNS/GC electrode in 0.1 M PBS (pH 7.0) of samples containing (A) 2.50 μM p-DHB and different concentrations of o-DHB (from a to i: 0.05, 0.10, 0.20, 0.50, 1.00, 1.60, 2.50, 4.00 and 6.00 μM) and (B) 2.50 μM o-DHB and different concentrations of p-DHB (from a to i: 0.05, 0.10, 0.20, 0.50, 1.00, 1.60, 2.50, 4.00 and 6.00 μM). The calibration curves for o-DHB (C) and p-DHB (D) detection. | |
Table 1 Comparison of different modified GC electrodes for the determination of o- and p-DHB
Electrode |
Detection limit (μM) |
Linear range (μM) |
Reference |
o-DHB |
p-DHB |
o-DHB |
p-DHB |
2,3-Dihydroxybiphenyl-1,2-dioxygenase |
0.428 |
|
2.0–800.0 |
|
4
|
Graphene–graphene oxide |
0.2 |
0.16 |
0.5–300.0 |
0.5–300.0 |
5
|
Polymeric ionic liquid–multiwalled carbon nanotube |
0.17 |
0.40 |
1.0–40.0 |
1.0–50.0 |
6
|
SiCNb |
0.8 |
1.6 |
39.8–980.0 |
39.8–980.0 |
31
|
DsDNA/polydiphenylamine-4-sulfonic acid |
0.648 |
|
0.75–8.25 |
|
32
|
Reduced graphene oxide |
0.1 |
0.2 |
1.0–200.0 |
6.0–200.0 |
33
|
CdSe |
0.06 |
0.011 |
0.2–300.0 |
0.6–1500.0 |
34
|
Thermally reduced graphene oxide |
0.8 |
0.75 |
1.0–500.0 |
1.0–500.0 |
35
|
Reduced graphene oxide–multiwall carbon nanotubes |
1.8 |
2.6 |
5.5–540.0 |
8.0–391.0 |
36
|
CD–AuNPs/HCNS |
0.01 |
0.02 |
0.05–6.00 |
0.05–6.00 |
This work |
Reproducibility, stability and anti-interference ability of CD–AuNPs/HCNS/GC electrode
The reproducibility and stability of the CD–AuNPs/HCNS/GC electrode were evaluated in 0.1 M PBS containing 1.00 μM o-DHB and 1.00 μM p-DHB. The results reveal that the modified electrode possesses a satisfying reproducibility with a relative standard deviation (RSD) of 4.87% for 12 parallel measurements with different electrodes. In addition, after the modified electrode was stored for 28 days at room temperature, a small current decrease (5.60%) was observed, and this value is 9.75% for 60 days. This reveals that the proposed electrode has good storage stability.
Possible interference during the simultaneous detection of o- and p-DHB at CD–AuNPs/HCNS/GC electrode was also investigated by the addition of various ions and organic pollutants to 0.1 M PBS in the presence of 1.00 μM o-DHB and 1.00 μM p-DHB. The results suggest that no interference was observed for the following inorganic ions: K+, Na+, Mg2+, Al3+, Ca2+, Zn2+, Cl−, SO42−, and NO3− (50 equiv.). Similarly, no interference was observed for methanol, ethanol, fenpropathrin, pentachlorophenol, or anthracene-9-carboxylic acid (10 equiv.). In particular, in the presence of resorcinol (RC, another dihydroxybenzene isomer), a new oxidation peak for RC was observed at 0.580 V (Fig. 8), but the RC did not interfere with the simultaneous detection of o- and p-DHB, indicating that the proposed modified electrode could be applied in selective simultaneous electrochemical determination for the three types of dihydroxybenzene isomers (o-DHB, p-DHB and RC). The abovementioned results also demonstrate that the CD–AuNPs/HCNS/GC electrode is suitable for the routine simultaneous analysis of o- and p-DHB in practical samples.
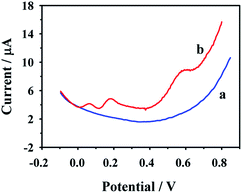 |
| Fig. 8 DPV responses of the CD–AuNPs/HCNS/GC electrode in 0.1 M PBS without (a) and with (b) o-DHB, p-DHB and RC. | |
Determination of o-DHB and p-DHB in real water samples
To further explore its practical applications, the developed CD–AuNPs/HCNS/GC electrode was used to detect o- and p-DHB in real water samples, which were collected from Changjiang River (Zhenjiang). Certain amounts of o- and p-DHB were spiked in the water samples. After dilution with 0.1 M PBS, the concentrations of o- and p-DHB in each sample were determined by the standard addition method and simultaneously analyzed by high-performance liquid chromatography (HPLC) to testify the accuracy of this method. A T-test was performed to compare the values of o- and p-DHB obtained from the proposed method with those from HPLC (Table 2). It showed that there are no statistically significant differences between the results of analyte concentrations obtained from these two methods. Furthermore, it was noted that quantitative recoveries of 94.9–108.0% are obtained by the electrochemical method. These results reveal that the developed method is satisfactory.
Table 2 The determination of o- and p-DHB in water samples
Water sample |
Added (μM) |
Found mean ± SD (μM) |
Recovery mean ± SD (%) |
By HPLC (μM) |
T-testa |
o-DHB |
p-DHB |
o-DHB |
p-DHB |
o-DHB |
p-DHB |
o-DHB |
p-DHB |
o-DHB |
p-DHB |
is the average concentration measured by the proposed method in this paper; μ0 is the concentration measured by HPLC; n is the degree of freedom and the confidence level is 95%; t0.052 = 4.30.
|
a |
1.00 |
1.00 |
1.08 ± 0.030 |
1.03 ± 0.030 |
108.0 ± 0.030 |
103.0 ± 0.030 |
1.01 |
1.03 |
3.27 |
0.00 |
b |
2.00 |
2.00 |
2.11 ± 0.050 |
1.96 ± 0.050 |
105.5 ± 0.025 |
98.0 ± 0.025 |
2.08 |
1.97 |
0.84 |
−0.28 |
c |
4.50 |
4.50 |
4.39 ± 0.120 |
4.27 ± 0.170 |
97.6 ± 0.028 |
94.9 ± 0.038 |
4.39 |
4.25 |
0.00 |
0.17 |
Conclusions
In summary, by introducing AuNPs, HCNS were successfully functionalized with CD, based on the formation of the “Au–S” bond, resulting in a novel CD–AuNPs/HCNS nanohybrid, which possess the synergistic effects from HCNS, CD and AuNPs. For the simultaneous analysis of dihydroxybenzene isomers, the CD–AuNPs/HCNS/GC electrode has a linear response range of 0.05–6.00 μM for both o- and p-DHB. Compared with the previously reported electrochemical methods for detecting o- and p-DHB, the method presented in this paper has considerably lower detection limits (0.01 and 0.02 μM for o- and p-DHB, respectively). Furthermore, the CD–AuNPs/HCNS/GC electrode has high selectivity, good reproducibility and good stability. It is believed that this work is very significant in creating a new avenue for the preparation of CDs-functionalized carbon-based materials. In addition, we believe that CD–AuNPs/HCNS hybrids have promising applications in electrochemical sensing and other fields such as chiral separation, drug delivery, and catalysis.
Acknowledgements
This work was supported by the National Natural Science Foundation of China (no. 21405062, 21175061 and 21375050), the China Postdoctoral Science Foundation (2014M551507), the Scientific Research Foundation for the Talents in Jiangsu University (13JDG091), and Jiangsu Planned Projects for Postdoctoral Research Funds (2014).
References
- H.-F. Wang, Y.-Y. Wu and X.-P. Yan, Anal. Chem., 2013, 85, 1920–1925 CrossRef CAS PubMed
.
- V. Vyskočil and J. Barek, Crit. Rev. Anal. Chem., 2009, 39, 173–188 CrossRef
.
- P. S. d. Silva, B. C. Gasparini, H. A. Magosso and A. Spinelli, J. Hazard. Mater., 2014, 273, 70–77 CrossRef PubMed
.
- Q. Zhang, Y. Qu, X. Zhang, J. Zhou and H. Wang, Biosens. Bioelectron., 2011, 26, 4362–4367 CrossRef CAS PubMed
.
- X. Zhou, Z. He, Q. Lian, Z. Li, H. Jiang and X. Lu, Sens. Actuators, B, 2014, 193, 198–204 CrossRef CAS PubMed
.
- X. Feng, W. Gao, S. Zhou, H. Shi, H. Huang and W. Song, Anal. Chim. Acta, 2013, 805, 36–44 CrossRef CAS PubMed
.
- S. Feng, W. Li, Q. Shi, Y. Li, J. Chen, Y. Ling, A. M. Asiri and D. Zhao, Chem. Commun., 2014, 50, 329–331 RSC
.
- D.-G. Lee, S. M. Kim, H. Jeong, J. Kim and I. S. Lee, ACS Nano, 2014, 8, 4510–4521 CrossRef CAS PubMed
.
- R. Liu, S. M. Mahurin, C. Li, R. R. Unocic, J. C. Idrobo, H. Gao, S. J. Pennycook and S. Dai, Angew. Chem., Int. Ed., 2011, 50, 6799–6802 CrossRef CAS PubMed
.
- J. Liu, W. Liu, K. Chen, S. Ji, Y. Zhou, Y. Wan, D. Xue, P. Hodgson and Y. Li, Chem.–Eur. J., 2013, 19, 9811–9816 CrossRef CAS PubMed
.
- N. Jayaprakash, J. Shen, S. S. Moganty, A. Corona and L. A. Archer, Angew. Chem., 2011, 123, 6026–6030 CrossRef
.
- Z. L. Schaefer, M. L. Gross, M. A. Hickner and R. E. Schaak, Angew. Chem., 2010, 122, 7199–7202 CrossRef
.
- X. Fang, J. Zang, X. Wang, M.-S. Zheng and N. Zheng, J. Mater. Chem. A, 2014, 2, 6191–6197 CAS
.
- Y. Liu, K. Ai and L. Lu, Chem. Rev., 2014, 114, 5057–5115 CrossRef CAS PubMed
.
- G. Cheng, M.-D. Zhou and S.-Y. Zheng, ACS Appl. Mater. Interfaces, 2014, 6, 12719–12728 CAS
.
- A. Harada, Y. Takashima and M. Nakahata, Acc. Chem. Res., 2014, 47, 2128–2140 CrossRef CAS PubMed
.
- Y. Wei, L.-T. Kong, R. Yang, L. Wang, J.-H. Liu and X.-J. Huang, Chem. Commun., 2011, 47, 5340–5342 RSC
.
- X. Chen, S. G. Parker, G. Zou, W. Su and Q. Zhang, ACS Nano, 2010, 4, 6387–6394 CrossRef CAS PubMed
.
- Á. Martínez, C. O. Mellet and J. M. G. Fernández, Chem. Soc. Rev., 2013, 42, 4746–4773 RSC
.
- G. Zhu, L. Wu, X. Zhang, W. Liu, X. Zhang and J. Chen, Chem.–Eur. J., 2013, 19, 6368–6373 CrossRef CAS PubMed
.
- Y.-L. Zhao, L. Hu, J. F. Stoddart and G. Grüner, Adv. Mater., 2008, 20, 1910–1915 CrossRef CAS
.
- G. Zhu, X. Zhang, P. Gai, X. Zhang and J. Chen, Nanoscale, 2012, 4, 5703–5709 RSC
.
- L. Kong, J. Wang, F. Meng, X. Chen, Z. Jin, M. Li, J. Liu and X.-J. Huang, J. Mater. Chem., 2011, 21, 11109–11115 RSC
.
- J. Shi, Y. Chen, Q. Wang and Y. Liu, Adv. Mater., 2010, 22, 2575–2578 CrossRef CAS PubMed
.
- A. Le Goff, K. Gorgy, M. Holzinger, R. Haddad, M. Zimmerman and S. Cosnier, Chem.–Eur. J., 2011, 17, 10216–10221 CrossRef CAS PubMed
.
- M. Holzinger, L. Bouffier, R. Villalonga and S. Cosnier, Biosens. Bioelectron., 2009, 24, 1128–1134 CrossRef CAS PubMed
.
- C. Xu, J. Wang, L. Wan, J. Lin and X. Wang, J. Mater. Chem., 2011, 21, 10463–10471 RSC
.
- Y. Gao, Y. Cao, D. Yang, X. Luo, Y. Tang and H. Li, J. Hazard. Mater., 2012, 199, 111–118 CrossRef PubMed
.
- P. Chen, H.-W. Liang, X.-H. Lv, H.-Z. Zhu, H.-B. Yao and S.-H. Yu, ACS Nano, 2011, 5, 5928–5935 CrossRef CAS PubMed
.
- Y. Guo, S. Guo, J. Ren, Y. Zhai, S. Dong and E. Wang, ACS Nano, 2010, 4, 5512 CrossRef CAS
.
- T. C. Canevari, L. T. Arenas, R. Landers, R. Custodio and Y. Gushikem, Analyst, 2013, 138, 315–324 RSC
.
- P. Wang, Y. Ni and S. Kokot, Analyst, 2013, 138, 1141–1148 RSC
.
- L. Chen, Y. Tang, K. Wang, C. Liu and S. Luo, Electrochem. Commun., 2011, 13, 133–137 CrossRef CAS PubMed
.
- X. Cao, X. Cai, Q. Feng, S. Jia and N. Wang, Anal. Chim. Acta, 2012, 752, 101–105 CrossRef CAS PubMed
.
- S.-J. Li, C. Qian, K. Wang, B.-Y. Hua, F.-B. Wang, Z.-H. Sheng and X.-H. Xia, Sens. Actuators, B, 2012, 174, 441–448 CrossRef CAS PubMed
.
- F. Hu, S. Chen, C. Wang, R. Yuan, D. Yuan and C. Wang, Anal. Chim. Acta, 2012, 724, 40–46 CrossRef CAS PubMed
.
Footnote |
† Electronic supplementary information (ESI) available. See DOI: 10.1039/c4tb01576b |
|
This journal is © The Royal Society of Chemistry 2015 |