DOI:
10.1039/C5TA06958K
(Paper)
J. Mater. Chem. A, 2015,
3, 21337-21342
Ultrathin MnO2 nanoflakes grown on N-doped carbon nanoboxes for high-energy asymmetric supercapacitors†
Received
2nd September 2015
, Accepted 14th September 2015
First published on 14th September 2015
Abstract
We demonstrate the synthesis of ultrathin MnO2 nanoflakes grown on N-doped carbon nanoboxes, forming an impressive hierarchical MnO2/C nanobox hybrid with an average size of 500 nm, which exhibits an excellent electrochemical performance due to the unique structure, N-doping and strong synergistic effects between them. In addition, we also assembled a green asymmetric supercapacitor (ASC) using the as-synthesized MnO2/C nanoboxes as a positive electrode and the corresponding N-doped carbon nanoboxes as a negative electrode in a neutral aqueous electrolyte, aiming to further enhance its energy density by extending the operating potential. More significantly, our ASC device is able to reversibly cycle within a wide operating voltage of 2.0 V and delivers a maximum energy density of 39.5 W h kg−1 with superior cycling stability (∼90.2% capacitance retention after 5000 cycles). These intriguing results show that hollow nanostructures will be promising electrode materials for advanced supercapacitors.
Introduction
In recent years, considerable attention has been paid to hollow nanostructures owing to their attractive properties such as high surface area, low effective density, low coefficients of thermal expansion and good permeation, which render them promising candidates for applications in energy storage, catalysis, adsorption materials, drug delivery, and some others.1–4 As for supercapacitors, a promising power source for portable electronic devices and electric vehicles (EVs) owing to their intriguing merits of high power density, long lifespan and environmental friendliness, many hollow structured electrode materials have been widely exploited, achieving a higher energy/power density indeed.5–7 However, their electrochemical performances, especially energy densities, are still unsatisfactory to meet the requirement of practical applications.8–10 Therefore, to further improve the energy density of supercapacitors, we need to design and synthesize novel hollow nanostructured electrode materials. On the other hand, according to equation E = C(ΔV)2/2, another effective strategy is to maximize the operating voltage (ΔV).11,12 In this regard, organic electrolytes or ionic liquids are developed to provide a wider potential window, but these nonaqueous media usually suffer from poor conductivity, high cost and safety concerns.13 Thankfully, asymmetric supercapacitors (ASCs) are a rather wise choice, particularly in neutral aqueous electrolytes, which will remarkably improve the energy density in virtue of the extended operating potential, but also meet the demand of green electrolytes.14–16 It is noted that high energy/power densities will be achieved if we can develop high performance positive and negative electrode materials and meanwhile assemble them into ASC devices accordingly.17
Thus far, manganese oxide (MnO2) has been extensively studied as an electrode material for supercapacitors owing to its high theoretical specific capacitance, environmental benignity and low cost.18–21 More impressively, MnO2 has a wide potential window in neutral aqueous electrolytes, making it more appealing than other metal oxides (e.g. NiO and Co3O4) usually used in strong acidic or alkaline electrolytes. However, pure MnO2 typically suffers from poor conductivity (10−5 to 10−6 S cm−1), which crucially depresses its practical capacity delivery.22 To address this issue, a general strategy is to prepare nanostructured MnO2 supported on conductive carbon substrates.23–25 For example, Chen et al.26 reported the synthesis of a bacterial-cellulose-derived carbon nanofiber@MnO2 (p-BC@MnO2) composite, which was then assembled into an ASC device in 1 M Na2SO4 aqueous solution using the corresponding p-BC as the negative electrode, showing an enhanced energy density of 32.9 W h kg−1. In addition, hetero-atom doping can introduce extra reversible pseudo-capacitance and enhance the electrical conductivity of carbon materials.27,28
Herein, we demonstrate the synthesis of novel hierarchical MnO2/C hollow nanostructures comprised of ultrathin MnO2 nanoflakes on N-doped carbon nanoboxes by using Fe2O3 nanocubes as a template and dopamine as a carbon source. An ASC device has been assembled in 1 M Na2SO4 aqueous solution based on the as-obtained MnO2/C nanoboxes as a positive electrode and the corresponding N-doped carbon nanoboxes as a negative electrode. As expected, the as-fabricated ASC device has a wide and stable operating voltage of 2.0 V, and an energy density as high as 39.5 W h kg−1 can be achieved with superior cycling stability (90.2% capacitance retention after 5000 cycles). Therefore, the design and synthesis of hollow nanostructures will promote the rapid development of advanced supercapacitors.
Experimental
Material synthesis
Synthesis of N-doped carbon nanoboxes.
Fe2O3 nanocubes, as a template, were firstly synthesized according to Lou's work.29 And then, 80 mg of the as-obtained Fe2O3 nanocubes was dispersed in 100 mL of 10 mM 2-amino-2-hydroxymethyl-propane-1,3-diol (Tris) solution. After that, 40 mg of dopamine was added into the mixture with magnetic stirring for 5 h. The products, i.e. Fe2O3@PDA, were collected and washed several times with deionized water and ethanol before drying at 70 °C. Finally, the N-doped carbon nanoboxes were obtained by annealing the Fe2O3@PDA nanocubes under Ar and subsequent acid etching of the core templates.
Synthesis of MnO2/C nanoboxes.
In a typical synthesis, 0.1 g of the above obtained N-doped carbon nanoboxes was dispersed into 50 mL of 0.1 M KMnO4 solution under stirring at 40 °C for 2 h. Subsequently, 10 mL of 0.2 M Mn(NO3)2 solution was added dropwise into the as-mentioned mixture with vigorous stirring for another 21 h. Then, the MnO2/C nanoboxes were collected, by filtration, washed several times with deionized water and alcohol, and dried at 70 °C overnight.
Material characterization
The structure and morphology of the as-prepared products were characterized with an X-ray powder diffractometer (XRD, Rigaku D/Max2550, Cu Kα radiation) at a scan rate of 1° min−1, a scanning electron microscope (FESEM, Hitachi S-4800), and a transmission electron microscope (TEM, JEOL-2100F) operating at 200 kV. X-ray photoelectron spectroscopy (XPS) spectra and the Raman spectrum were recorded with an AXIS Ultra DLD spectrometer (Al Kα X-ray source) and a NEXUS 670 FT-IR Raman spectrometer, respectively. N2 adsorption/desorption was carried out by Brunauer–Emmett–Teller (BET) measurements using a Micromeritics ASAP 2010 analyzer.
Electrochemical measurements
Electrochemical evaluation was carried out in 1 M Na2SO4 aqueous solution on an Autolab PGSTAT302N potentiostat electrochemical workstation. The electrode was prepared by mixing the active material, acetylene black and polyvinylidene fluoride (PVDF) at a ratio of 8
:
1
:
1 in NMP solvent. Then the as-formed slurry was coated onto graphite paper (1 cm2) using a doctor blade method and dried at 120 °C for 2 h. The ASC device was built based on MnO2/C nanoboxes as a positive electrode and N-doped carbon nanoboxes as a negative electrode with a glassy fibrous separator, which was then investigated using a two-electrode configuration in 1 M Na2SO4 aqueous electrolyte. Prior to constructing the ASC device, the mass ratio of positive and negative electrode materials was determined to be about m+/m− = 0.78 based on the specific capacitance values calculated from their CV results in a three-electrode system. The weights were 0.77 mg and 0.98 mg, respectively, for positive and negative electrodes. The specific capacitance of the ASC was calculated based on the total mass of both electrodes.
Results and discussion
The synthesis process of hierarchical MnO2/C nanoboxes is illustrated in Scheme 1. The carbon nanoboxes were first synthesized according to Lou's work29 with a minor revision. Speaking in detail, uniform Fe2O3 nanocubes with an average size of 500 nm as templates were first synthesized for the coating of carbon source polydopamine (PDA); the corresponding SEM images are shown in Fig. S1.† After carbonization and acid etching of the Fe2O3 core, the N-doped carbon nanoboxes were obtained, as shown in Fig. 1a, which are intact and uniform with a mean size of about 500 nm. The corresponding TEM image (Fig. 1b) demonstrates that these carbon nanoboxes possess a hollow nanostructure with a uniform shell thickness of about 20 nm. Notably, the hollow inner space of N-doped carbon nanoboxes can serve as the “buffering reservoir” for fast ion transport during the rapid charge/discharge process. Meanwhile, we also realize the ultrathin MnO2 nanoflakes grown on N-doped carbon nanoboxes by a simple strategy, forming the hierarchical MnO2/C nanoboxes. Fig. 1c shows the corresponding SEM image of the as-prepared MnO2/C nanoboxes. It can be seen that a layer of MnO2 has been entirely and uniformly grown on the surface of N-doped carbon nanoboxes. The high-magnification SEM image of a representative MnO2/C nanobox (Fig. S2†) reveals that the MnO2 layer is randomly assembled by numerous ultrathin MnO2 nanoflakes. The detailed microstructure of the MnO2/C nanoboxes was further characterized by TEM, as shown in Fig. 1d–f. Fig. 1d confirms the homogeneous coverage of MnO2 on N-doped carbon nanoboxes, which agrees well with SEM observation. The high-magnification TEM image (Fig. 1e) clearly shows that ultrathin MnO2 nanoflakes are directly grown on the surface of carbon substrates with lengths of about 20 nm. Such ultrathin MnO2 nanostructures possess enhanced accessibility to the electrolyte, ensuring high electrochemical utilization of active materials. From the high-resolution TEM (HRTEM) image (Fig. 1f), we can see the clear lattice fringes of MnO2 nanoflakes which are calculated to be about 0.23 nm. The selected area electronic diffraction (SAED) pattern (inset of Fig. 1f), taken from a random MnO2 nanoflake, shows a polycrystalline nature.
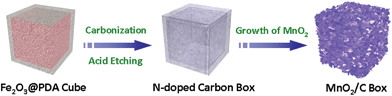 |
| Scheme 1 Schematic illustration of the synthesis process of the as-obtained MnO2/C nanoboxes. | |
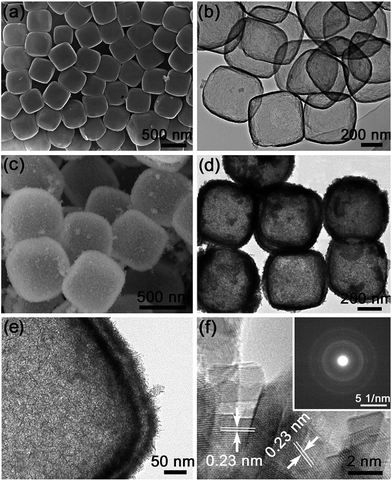 |
| Fig. 1 (a) SEM and (b) TEM images of the N-doped carbon nanoboxes. (c) SEM, (d and e) TEM and (f) HRTEM images of the MnO2/C nanoboxes. The inset of (f) shows the SAED pattern of the ultrathin MnO2 nanoflakes. | |
To analyze the chemical composition and oxidation state of Mn in the as-prepared MnO2/C composite, X-ray photoelectron spectroscopy (XPS) was conducted. The spectrum (Fig. 2a) shows the signals of Mn, O, C and N elements, implying the presence of MnO2 and successful doping of nitrogen which comes from the carbon precursor dopamine. The high-resolution Mn 2p spectrum (left inset of Fig. 2a) shows a Mn 2p3/2 peak at 642.0 eV and a Mn 2p1/2 peak at 653.7 eV with a spin-energy separation of 11.7 eV, being in good agreement with those reported for MnO2.22,30 The N 1s peak (right inset of Fig. 2a) can be resolved into three components centered at 398.4, 400.7, and 403.5 eV, corresponding to pyridinic (N-6), quaternary (N-Q), and graphitic types of nitrogen, respectively.31,32 It is reported that the nitrogen atoms can improve the wettability of active materials in the electrolyte and thus favor the ion transport.32,33 Moreover, the presence of N-Q and N-6 is beneficial to the conductivity and extra pseudocapacitance of carbon materials.34 To further confirm the crystalline phase of the ultrathin MnO2 nanoflakes, X-ray diffraction (XRD) analysis was carried out. Fig. 2b shows the XRD patterns of MnO2/C nanoboxes together with the corresponding N-doped carbon nanoboxes, in which the broad peak at about 26° for both samples is a typical characteristic of high graphitization carbon, while the diffraction peaks at 28.7°, 37.6°, 42.0°, 47.2°, 56.2°, 59.5° and 65.5° can be well assigned to the (310), (121), (301), (510), (600), (260) and (002) planes of the tetragonal α-MnO2 phase (JCPDS no. 72-1982).35 The Raman spectrum was also measured to analyze the carbon quality in MnO2/C nanoboxes. As shown in Fig. 2c, two strong peaks can be found at 1358 and 1597 cm−1, corresponding to the disordered D band and graphitic G band of carbon, respectively.36 The intensity ratio ID/IG is calculated to be 0.88, indicating a high graphitization degree. In addition, our MnO2/C nanoboxes also possess a high BET surface area of 130 m2 g−1 (Fig. S3†). Such intriguing features are beneficial for the rapid transportation of ions/electrons, leading to high energy/power densities.
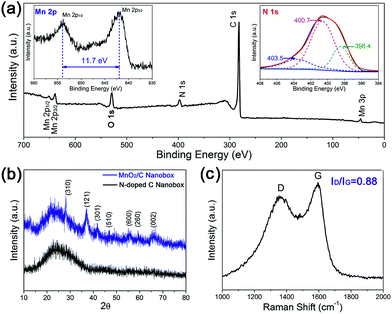 |
| Fig. 2 (a) XPS spectrum, (b) XRD patterns and (c) Raman spectrum of MnO2/C nanoboxes. The insets of (a) show the high-resolution spectra of Mn 2p and N 1s, respectively. | |
To evaluate the electrochemical potential windows and quantify the specific capacitance values of N-doped carbon nanoboxes and MnO2/C nanoboxes, cyclic voltammogram (CV) measurements were first carried out using a three-electrode configuration in 1 M Na2SO4 electrolyte. The N-doped carbon and MnO2/C electrodes were tested within the potential window of −1.0 to 0.2 V and 0–1.0 V, respectively, at a scan rate of 20 mV s−1. As shown in Fig. 3a, the relatively rectangular shapes of the CV curves without noticeable redox peaks indicate the good capacitive behavior for both electrodes. A series of galvanostatic charge/discharge tests were also conducted for both electrodes within the above potential windows at different current densities, as shown in Fig. S4.† The almost linear and triangular shapes further demonstrate the rapid current response and excellent electrochemical reversibility. The electrochemical performances are also better, at least comparable to the related studies in the literature.37–40 Thus, the stable operating potential window can be defined for the N-doped carbon electrode (−1.0 to 0.2 V) and MnO2/C electrode (0–1.0 V) with capacitive behavior. In this context, an extended operating voltage of 2.0 V is expected to be achieved when assembling an ASC device using the lower cutoff voltage of N-doped carbon (−1.0 V, negative electrode) and the higher cutoff voltage of MnO2/C (1.0 V, positive electrode), as shown in Fig. S5.†
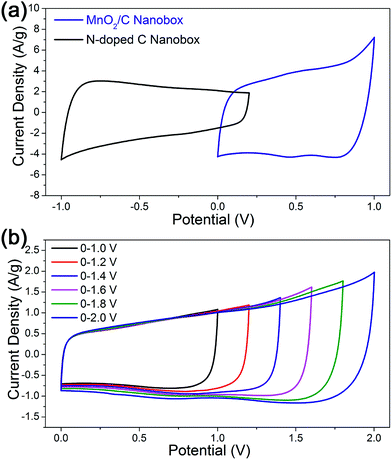 |
| Fig. 3 (a) CV curves of the N-doped carbon and the MnO2/C electrodes performed in a three-electrode configuration in 1 M Na2SO4 electrolyte at a scan rate of 20 mV s−1. (b) CV curves of the as-fabricated ASC device measured at different potential windows in 1 M Na2SO4 electrolyte at a scan rate of 20 mV s−1. | |
Prior to constructing the ASC device, the mass ratio of positive and negative electrodes (m+/m−) should be optimized based on the charge balance theory: q+ = q− (q = C × ΔE × m), where C, m, and ΔE represent the specific capacitance, loading mass, and potential range of each electrode.41 Based on the specific capacitance values calculated from the corresponding CV curves (Fig. 3a), which are 187 and 288 F g−1 for N-doped carbon and MnO2/C, respectively, the optimal mass ratio of m+/m− will be 0.78 in the present ASC device.
Fig. 3b shows the CV curves of the as-fabricated ASC device within different potential windows ranging from 1.0 to 2.0 V in 1 M Na2SO4 electrolyte at a scan rate of 20 mV s−1. As expected, CV curves at all potential windows showed quasi-rectangular and symmetric shapes, implying that the present ASC device possesses ideal capacitive behavior within a wide and stable operating voltage up to 2.0 V in neutral aqueous medium. It is known that the energy density is proportional to the square of voltage, so the enlarged operating voltage will lead to a remarkably enhanced energy density of supercapacitors.
Fig. 4a provides the galvanostatic charge/discharge curves recorded at various current densities of 0.2–2 A g−1 from which the rate performance of the as-fabricated ASC device can be evaluated. The typical triangular shapes of the charge/discharge curves exhibit considerable linear slopes with good symmetry at all current densities, indicating the good capacitive characteristics and fast charging/discharging profile of the ASC cell. A high specific capacitance of 71 F g−1 is achieved at 0.2 A g−1 for the ASC device calculated from the discharge curve, which still retains 42.4 F g−1 at 2 A g−1 with a capacitance retention of 60%, as shown in Fig. 4b, indicating a good rate performance. Moreover, the present ASC also possesses excellent cycling stability. As shown in Fig. 4c, a high capacitance retention of 90.2% is achieved after 5000 cycles performed at a current density of 2 A g−1. A Ragone plot derived from various discharge curves is provided to highlight the energy density vs. power density of the present ASC device, as shown in Fig. 4d. The as-prepared ASC cell shows a high energy density of 39.5 W h kg−1 at a power density of 200 W kg−1, which still retains 23.1 W h kg−1 at a high power density of 2000 W kg−1. This value also exceeds those of MnO2-based ASC devices with the aqueous electrolyte reported previously, such as MnO2/graphene//graphene (30.4 W h kg−1 at 100 W kg−1),19 MnO2/GO//hierarchical porous carbon (34.4 W h kg−1 at 200 W kg−1),42 MnO2/carbon nanofiber//activated carbon nanofiber (30.6 W h kg−1 at 200 W kg−1)43 and MnO2/graphitic hollow carbon spheres (GHCS-MnO2)//GHCS (22 W h kg−1 at 100 W kg−1).44 In a previous study, an ASC device based on petal-shaped MnO2 nanosheets as the positive electrode and a network of CNT–carbon nanofibers as the negative electrode was assembled by Wang et al., which delivered a very high energy density of 52.22 W h kg−1 at 100 W kg−1 in 0.5 M Na2SO4 electrolyte, but only 2000 cycles were accomplished in the cycling test with apparent capacity fading in the last several hundred cycles.45 These intriguing results demonstrate that the green ASC in our work can simultaneously achieve high energy density and excellent cycling stability.
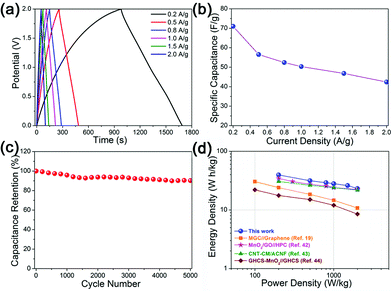 |
| Fig. 4 (a) Charge/discharge curves of the as-fabricated ASC device at different current densities. (b) The specific capacitance as a function of the current densities. (c) The capacitance retention as a function of cycle number at a current density of 2 A g−1. (d) Ragone plots of the present ASC device and other reported MnO2-based ASC devices. | |
The outstanding performance of the present ASC device could be attributed to the unique hollow nanostructures and the appropriate combination of each electrode component: (i) the N-doped carbon nanobox substrate is highly conductive to enhance the electron transport to the outer MnO2 layer, (ii) the ultrathin MnO2 nanoflakes possess abundant electroactive sites for the migration of electrolyte ions, leading to the effective utilization of active materials. Furthermore, the hydrophilic effect of N-doping also allows better access to the electrolyte, (iii) the hollow interiors can act as the ion reservoir to ensure the consecutive supply of the electrolyte even at high rates, further shortening the ion diffusion paths, and (iv) the directly grown MnO2 nanoflakes have close adhesion to the carbon substrate, ensuring the strong integrity of hierarchical MnO2/C nanoboxes and cycling stability. The above attractive findings demonstrate that the present ASC device based on hollow nanostructures would hold great potential as a green candidate for high-energy supercapacitors.
Conclusions
In conclusion, we have demonstrated the synthesis of a novel hierarchical MnO2/C hollow nanostructure by the growth of ultrathin MnO2 nanoflakes on N-doped carbon nanoboxes using Fe2O3 nanocubes as a template and dopamine as a carbon source, which exhibits excellent electrochemical performance due to the unique structure, N-doping and strong synergistic effects between them. To further enhance the energy density, a green ASC device has been assembled with a neutral aqueous electrolyte based on the as-prepared MnO2/C nanoboxes as a positive electrode and the corresponding N-doped carbon nanoboxes as a negative electrode. As expected, the as-fabricated ASC device can be operated reversibly in a wide potential range between 0 and 2.0 V in 1 M Na2SO4 aqueous electrolyte and deliver a maximum energy density as high as 39.5 W h kg−1 at a power density of 200 W kg−1. Furthermore, the present ASC device also exhibits superior cycling stability with 90.2% capacitance retention after 5000 cycles. These results will provide more insight into the development of hollow nanostructures for advanced supercapacitors.
Acknowledgements
This work was supported by the National Natural Science Foundation of China (21206043, 21236003, 21371057), the Basic Research Program of Shanghai (13NM1400801), the International Science and Technology Cooperation Program of China (2015DFA51220), the 111 Project (B14018), and the Fundamental Research Funds for the Central Universities. Authors V. B. and P. S. appreciate the financial support of the Ministry of Education, Youth and Sports of the Czech Republic – Program NPU I (LO1504).
Notes and references
- S. H. Im, U. Y. Jeong and Y. N. Xia, Nat. Mater., 2005, 4, 671–675 CrossRef PubMed.
- X. Y. Lai, J. E. Halpert and D. Wang, Energy Environ. Sci., 2012, 5, 5604–5618 CAS.
- H. Jiang, J. Ma and C. Z. Li, Adv. Mater., 2012, 24, 4197–4202 CrossRef CAS PubMed.
- J. Liu, S. Z. Qiao, S. B. Hartono and G. Q. Lu, Angew. Chem., Int. Ed., 2010, 122, 5101–5105 CrossRef PubMed.
- Y. Munaiah, B. G. S. Raj, T. P. Kumar and P. Ragupathy, J. Mater. Chem. A, 2013, 1, 4300–4306 CAS.
- T. Zhu, Z. Y. Wang, S. J. Ding, J. S. Chen and X. W. Lou, RSC Adv., 2011, 1, 397–400 RSC.
- W. Du, R. M. Liu, Y. W. Jiang, Q. Y. Lu, Y. Z. Fan and F. Gao, J. Power Sources, 2013, 227, 101–105 CrossRef CAS PubMed.
- H. Jiang, D. Y. Ren, H. F. Wang, Y. J. Hu, S. J. Guo, H. Y. Yuan, P. J. Hu, L. Zhang and C. Z. Li, Adv. Mater., 2015, 27, 3687–3695 CrossRef CAS PubMed.
- Y. Hou, Y. W. Cheng, T. Hobson and J. Liu, Nano Lett., 2010, 10, 2727–2733 CrossRef CAS PubMed.
- H. Jiang, P. S. Lee and C. Z. Li, Energy Environ. Sci., 2013, 6, 41–53 CAS.
- E. R. Pinero, F. Leroux and F. Beguin, Adv. Mater., 2006, 18, 1877–1882 CrossRef PubMed.
- L. L. Zhang and X. S. Zhao, Chem. Soc. Rev., 2009, 38, 2520–2531 RSC.
- H. C. Gao, F. Xiao, C. B. Ching and H. W. Duan, ACS Appl. Mater. Interfaces, 2012, 4, 2801–2810 CAS.
- Z. Fan, J. Yan, T. Wei, L. Zhi, G. Ning, T. Li and F. Wei, Adv. Funct. Mater., 2011, 21, 2366–2375 CrossRef CAS PubMed.
- B. G. Choi, M. Yang, W. H. Hong, J. W. Choi and Y. S. Huh, ACS Nano, 2012, 6, 4020–4028 CrossRef CAS PubMed.
- H. Jiang, C. Z. Li, T. Sun and J. Ma, Nanoscale, 2012, 4, 807–812 RSC.
- X. Zhao, L. L. Zhang, S. Murali, M. D. Stoller, Q. H. Zhang, Y. W. Zhu and R. S. Ruoff, ACS Nano, 2012, 6, 5404–5412 CrossRef CAS PubMed.
- M. J. Zhi, C. C. Xiang, J. T. Li, M. Li and N. Q. Wu, Nanoscale, 2013, 5, 72–88 RSC.
- Z. S. Wu, W. C. Ren, D. W. Wang, F. Li, B. L. Liu and H. M. Cheng, ACS Nano, 2010, 4, 5835–5842 CrossRef CAS PubMed.
- Y. Hou, Y. W. Cheng, T. Hobson and J. Liu, Nano Lett., 2010, 10, 2727–2733 CrossRef CAS PubMed.
- W. F. Wei, X. W. Cui, W. X. Chen and D. G. Ivey, Chem. Soc. Rev., 2011, 40, 1697–1721 RSC.
- S. W. Lee, J. Kim, S. Chen, P. T. Hammond and Y. Shao-Horn, ACS Nano, 2010, 4, 3889–3896 CrossRef CAS PubMed.
- S. Chen, J. W. Zhu, X. D. Wu, Q. F. Han and X. Wang, ACS Nano, 2010, 4, 2822–2830 CrossRef CAS PubMed.
- H. Zhang, G. P. Cao, Z. Y. Wang, Y. S. Yang, Z. J. Shi and Z. N. Gu, Nano Lett., 2008, 8, 2664–2668 CrossRef CAS PubMed.
- J. Ge, H. B. Yao, W. Hu, X. F. Yu, Y. X. Yan, L. B. Mao, H. H. Li, S. S. Li and S. H. Yu, Nano Energy, 2013, 2, 505–513 CrossRef CAS PubMed.
- L. F. Chen, Z. H. Huang, H. W. Liang, Q. F. Guan and S. H. Yu, Adv. Mater., 2013, 25, 4746–4752 CrossRef CAS PubMed.
- P. J. Hall, M. Mirzaeian, S. Isobel Fletcher, F. B. Sillars, A. J. R. Rennie, G. O. Shitta-Bey, G. Wilson, A. Cruden and R. Carter, Energy Environ. Sci., 2010, 3, 1238–1251 CAS.
- S. H. Yang, X. F. Song, P. Zhang and L. Gao, ACS Appl. Mater. Interfaces, 2013, 5, 3317–3322 CAS.
- X. Y. Yu, H. Hu, Y. W. Wang, H. Y. Chen and X. W. Lou, Angew. Chem., Int. Ed., 2015, 54, 7395–7398 CrossRef CAS PubMed.
- A. L. M. Reddy, M. M. Shaijumon, S. R. Gowda and P. M. Ajayan, Nano Lett., 2009, 9, 1002–1006 CrossRef CAS PubMed.
- Y. M. Tan, C. F. Xu, G. X. Chen, Z. H. Liu, M. Ma, Q. J. Xie, N. F. Zheng and S. Z. Yao, ACS Appl. Mater. Interfaces, 2013, 5, 2241–2248 CAS.
- F. Su, C. K. Poh, J. S. Chen, G. Xu, D. Wang, Q. Li, J. Lin and X. W. Lou, Energy Environ. Sci., 2011, 4, 717–724 CAS.
- H. Guo and Q. Gao, J. Power Sources, 2009, 186, 551–556 CrossRef CAS PubMed.
- W. R. Li, D. H. Chen, Z. Li, Y. F. Shi, Y. Wan, J. J. Huang, J. J. Yang, D. Y. Zhao and Z. Y. Jiang, Electrochem. Commun., 2007, 9, 569–573 CrossRef CAS PubMed.
- Y. Liu, M. Zhang, J. H. Zhang and Y. T. Qian, J. Solid State Chem., 2006, 179, 1757–1761 CrossRef CAS PubMed.
- H. Jiang, Y. J. Hu, S. J. Guo, C. Y. Yan, P. S. Lee and C. Z. Li, ACS Nano, 2014, 8, 6038–6046 CrossRef CAS PubMed.
- P. C. Chen, G. Z. Shen, Y. Shi, H. T. Chen and C. W. Zhou, ACS Nano, 2010, 4, 4403–4411 CrossRef CAS PubMed.
- M. Yang, Y. Zhong, X. Zhou and Z. Zhou, J. Mater. Chem. A, 2014, 2, 12519–12525 CAS.
- L. Li, R. M. Li, S. L. Gai, S. J. Ding, F. He, M. L. Zhang and P. P. Yang, Chem.–Eur. J., 2015, 21, 7119–7126 CrossRef CAS PubMed.
- M. Yang, Y. R. Zong, J. Bao, X. L. Zhou and Z. Zhou, J. Mater. Chem. A, 2015, 3, 11387–11394 CAS.
- M. Yang, Y. R. Zong, L. W. Su, J. P. Wei and Z. Zhou, Chem.–Eur.
J., 2014, 20, 5046–5053 CrossRef CAS PubMed.
- Y. F. Zhao, W. Ran, J. He, Y. Z. Huang, Z. F. Liu, W. Liu, Y. F. Tang, L. Zhang, D. W. Gao and F. M. Gao, Small, 2015, 11, 1310–1319 CrossRef CAS PubMed.
- J. G. Wang, Y. Yang, Z. H. Huang and F. Y. Kang, Carbon, 2013, 61, 190–199 CrossRef CAS PubMed.
- Z. B. Lei, J. T. Zhang and X. S. Zhao, J. Mater. Chem., 2012, 22, 153–160 RSC.
- C. H. Wang, H. C. Hsu and J. H. Hu, J. Power Sources, 2014, 249, 1–8 CrossRef CAS PubMed.
Footnote |
† Electronic supplementary information (ESI) available. See DOI: 10.1039/c5ta06958k |
|
This journal is © The Royal Society of Chemistry 2015 |
Click here to see how this site uses Cookies. View our privacy policy here.