DOI:
10.1039/C5TA00814J
(Paper)
J. Mater. Chem. A, 2015,
3, 11232-11238
Zwitterionic liquid crystals as 1D and 3D lithium ion transport media†
Received
31st January 2015
, Accepted 19th March 2015
First published on 20th March 2015
Abstract
We describe the development of self-assembled one- and three-dimensional lithium ion conductors composed of zwitterionic liquid crystals, lithium bis(trifluoromethylsulfonyl)imide (LiTFSI) and propylene carbonate (PC). Two types of wedge-shaped zwitterions based on imidazolium dicyanoethenolate and sulfonate were synthesized. These compounds alone show liquid-crystalline (LC) columnar hexagonal (Colh) phases and low ionic conductivities (10−8 to 10−7 S cm−1). The increase in the ionic conductivities was achieved by the addition of LiTFSI (10−5 S cm−1) followed by that of PC (10−4 S cm−1). Moreover, LC bicontinuous cubic (Cubbi) phases are induced by tuning the ionic nature of the zwitterionic liquid crystal with the ratio of LiTFSI and PC. The dissociation of LiTFSI in the zwitterions and the ion–dipole interaction between the lithium ions and PC are shown to be significant keys for the enhancement of the conductivities and stabilization of the nanosegregated LC structures.
Introduction
The construction of supramolecular nanostructures with diverse functionalities using molecular self-assembly is of increasing interest for emerging nanotechnologies.1–19 An important challenge is to build nanochannels capable of ion transport.20–44 The use of liquid-crystalline (LC) self-assembly is a promising approach for the development of nanochannels with various dimensions and enhanced ion transport function.20,30–46 Recently, LC ion-conductive materials have been applied to energy devices, such as lithium ion batteries and dye-sensitized solar cells.47–50 Among the LC organizations, thermotropic LC bicontinuous cubic (Cubbi) assemblies have attracted considerable attention because they form three-dimensionally interconnected ionic channels suitable for ion transport.34,38,44–46 However, the design of three-dimensional (3D) ion-conductive thermotropic LC materials is still challenging because the formation of Cubbi phases requires a delicate balance of volume and interactions between the polar and the non-polar parts in the material.44 One of the approaches for the induction of functional thermotropic Cubbi phases is the use of two-component assemblies.51–54 For example, we achieved 3D proton transport in the Cubbi phases formed by the complexation of zwitterionic molecules and organic acids.52,53 The acid dissociates in the presence of zwitterions, which enables the proton transport. Interestingly, the proton conductivity and LC behavior are tuned by the amount of acid and also by the further addition of water.53 LC Cubbi phases were also induced for the complexes of pyridinium-based zwitterions and lithium salts, although their ionic conductivities were not examined.54 Anhydrous 3D lithium ion transport was previously achieved in LC materials by mixing lithium salts and ionic molecules.31,55 For example, we developed nanostructured polymer electrolytes upon photo-crosslinking of thermotropic LC Cubbi assemblies composed of wedge-shaped ammonium salts and a lithium salt.55 Gin and co-workers also reported LC Cubbi polymer electrolytes based on the lyotropic LC assemblies of a polymerizable lithium sulfonate and a dilute LiClO4 solution in propylene carbonate (PC).31 These approaches are based on the solubilization of lithium ions in nanostructured channels. However, we consider that the dissociation of lithium salts in zwitterionic LC media is a promising strategy for the development of lithium ion transport materials with induced Cubbi phases.
Herein we report on the development of one-dimensional (1D) and 3D lithium ion transport channels (Fig. 1). We designed and prepared imidazolium-type zwitterions 1 and 2 (Fig. 2) to co-assemble with lithium bis(trifluoromethylsulfonyl)imide (LiTFSI) and PC forming well-defined ionic pathways capable of lithium transport (Fig. 1 and 2). Our approach and material design in the present study is to use zwitterionic liquid crystals as media for lithium ion transport. Zwitterionic liquid crystals tethering charge-delocalized anions are expected to form well-defined ionic channels, but have no transportable ions (Fig. 1, left). After the addition of a lithium salt, the salt may be dissociated by the interactions with zwitterionic moieties, which allows lithium to move along the ionic pathways (Fig. 1, middle). At the same time, our intention is to increase the lithium ion mobility by incorporating PC as a polar additive (Fig. 1, right).31 It is expected that the optimization of the ratio of additives, lithium salt and PC, can induce Cubbi phases and enhance the ionic conductivities (Fig. 1).
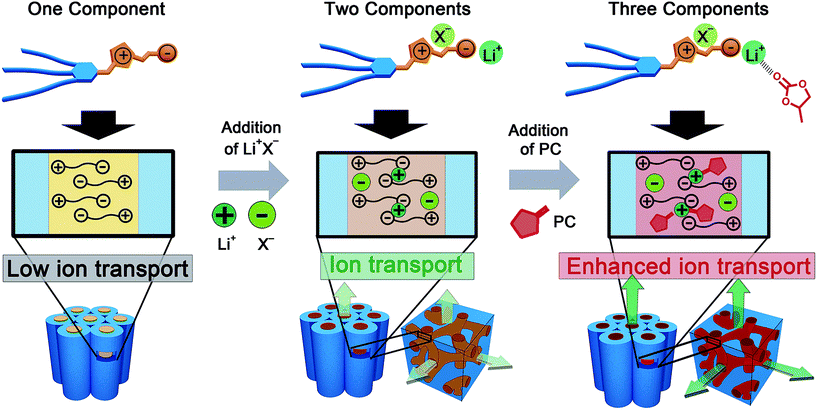 |
| Fig. 1 Our design strategy for the enhancement of lithium ion transport based on three components of nanostructured liquid-crystalline (LC) materials formed by the self-assembly of a zwitterionic liquid crystal, a lithium salt and propylene carbonate (PC). Green arrows indicate the direction of ion transport. The ionic channels are shown in yellow, orange, or red colors depending on their composition and the aliphatic parts are indicated in blue. The proposed organizations of molecules and salt inside the ionic channels are shown in the zoom windows from side views. | |
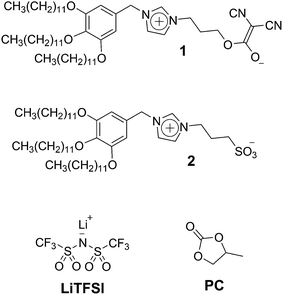 |
| Fig. 2 Chemical structures of the LC zwitterions 1 and 2, lithium bis(trifluoromethylsulfonyl)imide (LiTFSI) and PC. | |
Results and discussion
Material design
Wedge-shaped zwitterions 1 and 2 (Fig. 2) are designed to act as LC ion transport media. The ionic parts of 1 and 2 consist of an imidazolium moiety tethering a dicyanoethenolate anion56–59 or a sulfonate anion52–54,60 through a propylene spacer. We expected that compounds 1 and 2 would show different LC phase transition behavior and ion transport properties due to the differences in the size of the anions and in the distribution of negative charges. LiTFSI (Fig. 2) is chosen as a lithium ion source because of its thermal stability and high delocalization of negative charges.35,54,61 Ohno and co-workers previously reported that the equimolar mixtures of imidazolium-based sulfobetaine whose melting point is over 100 °C and lithium salts (LiTFSI, LiOSO2CF3, LiBF4, and LiClO4) become ionic liquids at room temperature.62 Among them, the LiTFSI complex exhibits the lowest glass transition temperature and the highest ionic conductivity.62 Lin and co-workers reported that the mixtures of imidazolium-type carboxylates or sulfonates and LiTFSI form smectic A phases exhibiting ionic conductivities.63,64 In addition, the employment of bulky LiTFSI as an additive for zwitterionic liquid crystals can induce structural changes of LC phases because of the change of the molecular shapes and the volume balance between the polar and nonpolar parts.35,54 Thus we considered that the complexation of 1 or 2 and LiTFSI would lead to the development of 3D lithium ion conductive materials exhibiting Cubbi LC phases. Furthermore, to enhance the dissociation of lithium ions in the zwitterionic ion transport media, PC (Fig. 2) is used as a polar additive because the carbonyl group of PC has the ability to coordinate to lithium ions.31,40,48,61
Supramolecular interactions between components
In order to examine the interactions between lithium ions, zwitterions and PC, 7Li NMR measurements of LiTFSI, the mixture of 1 and LiTFSI and the tertiary mixture of LiTFSI, 1 and PC were conducted in the CDCl3/THF-d6 solutions using LiCl in D2O as an internal standard (Fig. 3). The results for 2 are shown in the ESI (Fig. S10†). The 7Li chemical shift of LiTFSI shows a downfield shift of 0.06 ppm in the presence of 1 (Fig. 3, middle). This result suggests the formation of a complex between 1 and LiTFSI, where the lithium ion may interact with the dicyanoethenolate anion (Fig. 1, top middle and Fig. S9†).65,66 The addition of PC to the mixture of 1 and LiTFSI induces a further downfield shift of 0.03 ppm (Fig. 3, top) compared to the chemical shift for the mixture of 1 and LiTFSI (Fig. 3, middle). This shift may be attributed to the ion–dipole interactions between lithium ions and PC. It should be mentioned that the stepwise downfield shifts of the 7Li signal are detected by the addition of zwitterions followed by PC, in spite of the presence of THF which is a competitive solvent.
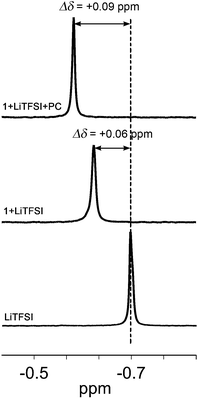 |
| Fig. 3
7Li NMR spectra of LiTFSI (0.013 m, 450 μl) (bottom), 1/LiTFSI (0.02 m/0.013 m, 450 μl) (middle) and 1/LiTFSI/PC (0.02 m/0.013 m/0.13 m, 450 μl) (top) in CDCl3 : THF-d6 (v/v, 6 : 1) solution. LiCl D2O solution in a coaxial NMR tube was used as an internal standard and the lithium signal was set at 0 ppm. The dashed line indicates the chemical shift of the lithium ion for the pure LiTFSI solution. All the spectra were recorded at room temperature. | |
The interactions between 1, LiTFSI and PC were also confirmed by IR measurements in the bulk state. The IR spectra of 1, the mixture of 1 and LiTFSI and the tertiary mixture of 1, LiTFSI and PC were recorded at room temperature (Fig. S11†). Single compound 1 shows two absorption bands at 2193 and 2165 cm−1 which are characteristic of the C
N vibrations of the dicyanoethenolate moiety.57 These bands are shifted to 2202 and 2173 cm−1, respectively, after the addition of 50 mol% of LiTFSI. Further addition of 10 wt% of PC resulted in a high wavenumber shift of CN bands to 2206 and 2179 cm−1, respectively. These results suggest the existence of interactions between the zwitterionic molecule, LiTFSI and PC.
Liquid-crystalline properties
Compounds 1 and 2 show LC columnar hexagonal (Colh) phases from room temperature to around 200 °C at which decomposition occurs (Table 1 and S1†). Mixtures of the zwitterions with LiTFSI [1/Li+(x) and 2/Li+(x), x denotes the mol% of LiTFSI] were prepared by mixing the THF solutions of LiTFSI and the zwitterions, followed by the evaporation of the solvent to yield the anhydrous mixtures as white solids. The liquid crystallinity of the 1/Li+(x) and 2/Li+(x) mixtures was examined by polarizing optical microscopy (POM), X-ray diffraction (XRD) and differential scanning calorimetry. The 1/Li+(x) mixtures with 10–40 mol% of LiTFSI and all the 2/Li+(x) mixtures exhibit only Colh phases (Table 1 and S1†).‡ As for 1/Li+(50), the Cubbi phase is induced in addition to the formation of the Colh phase. The Cubbi phase is observed from room temperature to 65 °C and slowly changes to the Colh phase between 65 and 88 °C under POM observation at the heating rate of 5 °C min−1. The formation of ionic pairs composed of ionic liquid-like imidazolium TFSI and lithium dicyanoethenolate, which is bulkier than lithium sulfonate, may disturb the columnar packing.59 The change in the volume fraction of the polar and nonpolar parts may also contribute to the induction of the Cubbi phase.51–54Fig. 4 and 5 show the XRD patterns and POM images of 1/Li+(50) in the Cubbi and Colh phases. The small-angle XRD pattern of 1/Li+(50) at 55 °C (Fig. 4a) shows two strong and three weak peaks at 39.2, 34.0, 25.7, 21.4 and 20.4 Å corresponding to the (211), (220), (321), (420) and (332) reflections, respectively. The reciprocal d-spacing ratio of these peaks is √6
:
√8
:
√14
:
√20
:
√22, which corresponds to a Cubbi phase with an Ia3d symmetry. In contrast, the wide-angle XRD pattern of 1/Li+(50) at 120 °C (Fig. 4b) shows the (100), (110) and (200) reflections, which are characteristic of a Colh phase. The POM image of 1/Li+(50) at 58 °C shows no birefringence (Fig. 5a), indicating the formation of a Cubbi phase. By heating the sample to 90 °C (Fig. 5b), a birefringence upon the formation of the Colh phase with polydomain organization is observed.
Table 1 LC behavior of zwitterionic compounds and their mixtures with LiTFSI and/or PC
Samplea |
Phase transition behaviorb |
Ratio of LiTFSI and PC is indicated as follows: 1/Li+(x), 2/Li+(x), 1/Li+(x)PC(y) and 2/Li+(x)PC(y), where x is the mol% of LiTFSI and y indicates the wt% of PC.
Determined by polarizing optical microscope observation. The LC behavior was examined on heating processes (5 °C min−1) from 25 °C to decomposition or isotropization temperature of the sample. The given transition temperatures correspond to the starting of the phase transitions. In the case of a slow phase transition, a temperature range is given. Mixtures containing more than 50 mol% of LiTFSI show no homogeneous LC phases. Mixtures with 15 wt% or more of PC showed biphasic behavior.
For PC containing samples, the microscopic observation was conducted up to 120 °C. All the mixtures showed decomposition at around 200 °C. Colh: columnar hexagonal, Cubbi: bicontinuous cubic, Iso: isotropic, and Decomp.: decomposition.
|
1
|
|
|
Colh |
217 |
Decomp. |
1/Li+(20) |
|
|
Colh |
190 |
Decomp. |
1/Li+(50) |
Cubbi |
65–88 |
Colh |
190 |
Decomp. |
1/Li+(20)PC(5) |
Cubbi |
50–68 |
Colh |
120c |
|
1/Li+(20)PC(10) |
Cubbi |
50–75 |
Colh |
120c |
|
1/Li+(50)PC(5) |
Cubbi |
81–92 |
|
|
Iso |
1/Li+(50)PC(10) |
Cubbi |
74–86 |
|
|
Iso |
2
|
|
|
Colh |
207 |
Decomp. |
2/Li+(20) |
|
|
Colh |
200 |
Decomp. |
2/Li+(50) |
|
|
Colh |
200 |
Decomp. |
2/Li+(20)PC(5) |
|
|
Colh |
120c |
|
2/Li+(20)PC(10) |
|
|
Colh |
120c |
|
2/Li+(50)PC(5) |
|
|
Colh |
120c |
|
2/Li+(50)PC(10) |
|
|
Colh |
120c |
|
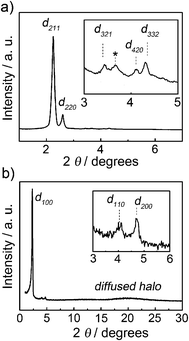 |
| Fig. 4 X-ray diffraction (XRD) patterns of 1/Li+(50) at (a) 55 °C and (b) 120 °C. The mark * corresponds to the diffraction of the Kapton® film. | |
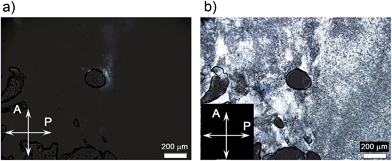 |
| Fig. 5 Polarizing optical microscopy (POM) images of 1/Li+(50) at (a) 58 °C and (b) 90 °C. a: analyzer; p: polarizer. | |
Compounds 1 and 2 and the mixtures 1/Li+(20), 1/Li+(50), 2/Li+(20) and 2/Li+(50) were selected to study the effects of the addition of PC. Compounds 1 and 2 show biphasic behavior after the addition of 5 and 10 wt% of PC.§ In contrast, the mixtures 1/Li+(x) and 2/Li+(x) form homogenous LC mixtures upon the addition of 5 or 10 wt% of PC [1/Li+(x)PC(y) and 2/Li+(x)PC(y), y indicates the wt% of PC] (Table 1).¶ These results suggest that LiTFSI is essential for the stabilization of the LC phases in the mixtures containing zwitterions 1 or 2 and PC. The mixtures of 2/Li+(x)PC(y) show only Colh phases, but the mixtures based on 1/Li+(x)PC(y) exhibit Cubbi LC phases (Table 1). This behavior also indicates that the interactions of dicyanoethenolate anions, lithium ions and PC play key roles in the induction of cubic phases. For example, 1/Li+(20) shows only a Colh phase, but Cubbi phases are formed after the addition of 5 or 10 wt% of PC (Table 1). Moreover, the cubic phase observed for 1/Li+(50) is thermally stabilized up to 80 °C by adding PC (Table 1). Remarkably, as far as we know the use of PC for the induction or stabilization of Cubbi phases has not yet been reported.
In order to obtain more details about the structural organization of these complexes, we studied the XRD parameters of the LC phases of 1, 2, and the selected mixtures with LiTFSI and LiTFSI/PC. Table 2 displays the unit cell distances for the Cubbi phases and the intercolumnar distances for the Colh phases of the selected mixtures. Compounds 1 and 2 in the Colh phases show intercolumnar distances of 40 Å. The molecular lengths of 1 and 2 in the extended conformation are estimated to be 33 and 30 Å, respectively. Thus, these results suggest that these zwitterionic molecules self-assemble into well-packed Colh structures, where aliphatic chains and zwitterionic moieties are interdigitated (Fig. 1 and S9†).51–54,60,63,64 The addition of 50 mol% of LiTFSI to 1 and 2 leads to an increase in the intercolumnar distances (Table 2). In the case of 2/Li+(50), the intercolumnar distance also increases upon the addition of 10 wt% of PC. A similar increase is observed in the Cubbi phases of 1/Li+(50) after the addition of 10 wt% of PC, where the unit cell distance increases by 2.6 Å (Table 2). These results imply that LiTFSI and PC are incorporated within the ionic channels by interacting with the zwitterionic moieties (Fig. 1, S8, S9 and S11†).
Table 2 Lattice parameters of the LC phases determined by XRD measurements
Sample |
Lattice parameters (Å) |
LC phasea |
Temperature (°C) |
Colh: columnar hexagonal; Cubbi: bicontinuous cubic.
|
1
|
40.8 |
Colh |
120 |
1/Li+(50) |
43.4 |
Colh |
120 |
1/Li+(50) |
96.1 |
Cubbi |
55 |
1/Li+(50)PC(10) |
98.7 |
Cubbi |
60 |
2
|
40.4 |
Colh |
100 |
2/Li+(50) |
41.3 |
Colh |
100 |
2/Li+(50)PC(10) |
42.2 |
Colh |
100 |
Ionic conductivities
The ionic conductivities of the zwitterions 1 and 2, and the mixtures 1/Li+(x), 2/Li+(x), 1/Li+(x)PC(y) and 2/Li+(x)PC(y) were measured as a function of temperature (Fig. 6, 7 and S12†) by the alternating current impedance method.33 All the conductivities were measured using heating processes. Fig. 6 shows the ionic conductivities of the mixtures based on 1 (Fig. 6a) and 2 (6b) containing 0 to 50 mol% of LiTFSI. All samples are not aligned. The samples exhibiting the Colh phases show polydomain textures under POM observation (Fig. 5b). The addition of LiTFSI to 1 and 2 significantly increases the ionic conductivities. This observation suggests that the presence of mobile species enhances the ionic conductivities in the systems. The ionic conductivities for 1/Li+(x) and 2/Li+(x) increase with increasing amounts of LiTFSI. This enhancement is more significant for the 2/Li+(x) mixtures than that for the 1/Li+(x) mixtures. For example, the value of the ionic conductivity of 2 at 100 °C is 5 × 10−9 S cm−1 while the 2/Li+(50) mixture shows the value of 6 × 10−6 S cm−1. On the other hand, the addition of 50 mol% of LiTFSI to 1 increases the conductivity from 1 × 10−8 S cm−1 to 4 × 10−6 S cm−1 at 100 °C. All the mixtures show a gradual increase in the ionic conductivity with the increase of temperature. Compound 1/Li+(50) shows a change in the increasing trend of the ionic conductivities between 65 and 80 °C on heating (Fig. 6a), probably due to the slow Cubbi–Colh phase transition.34,52
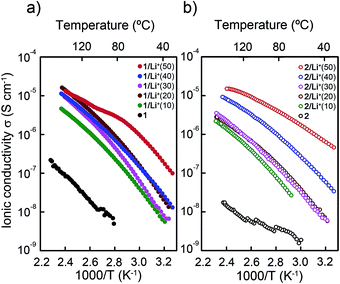 |
| Fig. 6 Ionic conductivities as a function of temperature of (a) 1 and the mixtures 1/Li+(x) and (b) 2 and the mixtures 2/Li+(x). x indicates the mol% of LiTFSI. | |
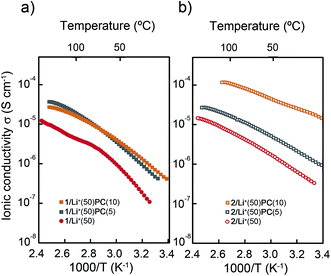 |
| Fig. 7 Ionic conductivities as a function of temperature for the mixtures; (a) 1/Li+(50)PC(y) and (b) 2/Li+(50)PC(y). y denotes the wt% of PC. | |
It is noteworthy that the highest conductivities in the 1/Li+(x) and 2/Li+(x) series are obtained in the 1
:
1 mixtures (Fig. 6). Previous studies on LC lithium ion transport materials used lower amounts of lithium salts.31,55,63,64 Generally, a low lithium ion concentration in the electrolytes leads to better ionic conductivities.67,68 For example, McFarlane and coworkers reported that for the binary mixtures of N-alkylmethylpyrrolidinium TFSI and LiTFSI the conductivity continued to increase by doping less than 30 mol% of LiTFSI and further doping up to 50 mol% of LiTFSI resulted in the decrease in the conductivity.68 It is assumed that in the present system the 1
:
1 mixtures form ionic liquid-like structures62 within the ionic channels, resulting in the increase of the ionic mobility.
Interestingly, the addition of PC to 1/Li+(x) and 2/Li+(x) leads to the enhancement of ionic conductivities (Fig. 7 and S12†). The ionic conductivity of 1/Li+(50) increases four times after the addition of 5 or 10 wt% of PC (Fig. 7a). On the other hand, a stepwise increase in the conductivities for 2/Li+(50) is seen by adding 5 and 10 wt% of PC (Fig. 7b). The highest ionic conductivities in the series are observed for 2/Li+(50)PC(10). The value reaches 10−4 S cm−1 at around 100 °C.||.
The activation energies (Ea) of ion conduction were estimated for 1/Li+(50) and 1/Li+(50)PC(10) in the Cubbi phases and for 2/Li+(50) and 2/Li+(50)PC(10) in the Colh phases (ESI†). It was found that Ea significantly decreased after the addition of PC to 1/Li+(x) and 2/Li+(x). For example, the value of Ea for 1/Li+(50) in the Cubbi phase was 64 kJ mol−1, while that for 1/Li+(50)PC(10) was 41 kJ mol−1. In the case of 2/Li+(50) and 2/Li+(50)PC(10) in the Colh phase, the values are 38 kJ mol−1 and 23 kJ mol−1, respectively. These reductions in the Ea by the addition of PC were attributed to the weakening of the interactions between zwitterions and lithium ions upon the formation of ion–dipole interactions between the lithium ions and PC (Fig. 3). The formation of more mobile ionic channels would facilitate the transport of lithium ions.
Conclusions
We successfully developed 1D and 3D lithium ion transport LC materials by the co-assembly of wedge-shaped zwitterions (1 or 2), LiTFSI and PC. LC zwitterions provide stable nanostructured media for lithium ion transport and exhibit LC phases with up to 50 mol% of LiTFSI and up to 10 wt% of PC. The LC phases and ionic conductivities are tuned by the appropriate molecular design and changing the ratio of LiTFSI and PC. Remarkably, the supramolecular interactions between zwitterions, LiTFSI and PC are the key for the stabilization of LC phases and for the enhancement of conductivities. It is expected that the ionic conductivities may be further enhanced by increasing the PC fraction of the electrolyte materials. The system described here introduces important advances in the development of nanostructured lithium ion-conductive materials, and opens new pathways for the development of LC electrolytes that can be applied in energy devices.
Experimental
Synthesis and characterization of compounds 1 and 2 are described in the ESI.†
Preparation of the mixtures
Compounds 1 and 2 were dried under vacuum at 80 °C for 12 h before their use. They showed no hygroscopic behavior for a period of days under ambient conditions. All the materials and solvents for the preparation of the mixtures were dried before use.
Mixtures 1/Li+(x) and 2/Li+(x) were prepared by adding the appropriate volume of a THF solution of LiTFSI (0.026 M) to a weighed amount of zwitterions 1 and 2 (10–30 mg) in a microtube. The solution was homogenised by sonication and then the solvent was slowly removed by rotary evaporation. The samples were dried under vacuum at 80 °C for 12 h before their study. The absence of volatiles was confirmed by thermogravimmetric analyses (Fig. S1†).
The mixtures 1/Li+(x)PC(y) and 2/Li+(x)PC(y) were prepared by adding the appropriate amount of PC to 1/Li+(x) and 2/Li+(x), respectively. The solid blend was homogenised by using a spatula and then heated to 50 °C for 5 min. These mixtures were prepared under ambient conditions in the laboratory (humidity: 40–60 %). The presence of volatiles was monitored by thermogravimetric analyses (Fig. S1†). The thermograms indicate no inclusion of volatiles in the sample, but the loss of less than 1 wt% of the added PC during the sample preparation (Fig. S1†).
Acknowledgements
This study was partially supported by the Funding Program for World-Leading Innovative R&D on Science and Technology (FIRST) from the Cabinet Office, Government of Japan. This work was also partially supported by a Grant-in-Aid for Scientific Research (no. 22107003) in the Innovative Area of “Fusion Materials” (Area no. 2206) from the Ministry of Education, Culture, Sports, Science and Technology (MEXT). M. Y. is grateful for financial support from Grant-in-Aid for Young Scientists (A) (no. 2570813) from the Japan Society for the Promotion of Science and The Noguchi Institute.
Notes and references
-
Handbook of Liquid Crystals, ed. J. W. Goodby, P. J. Collings, T. Kato, C. Tschierske, H. Gleeson and P. Raynes, Wiley-VCH, Weinheim, Germany, 2nd edn, 2014 Search PubMed
.
- T. Kato, N. Mizoshita and K. Kishimoto, Angew. Chem., Int. Ed., 2006, 45, 38–68 CrossRef CAS PubMed
.
- D. J. Broer, C. M. W. Bastiaansen, M. G. Debije and A. P. H. J. Schenning, Angew. Chem., Int. Ed., 2012, 51, 7102–7109 CrossRef CAS PubMed
.
- W. Pisula, X. Feng and K. Müllen, Adv. Mater., 2010, 22, 3634–3649 CrossRef CAS PubMed
.
- N. Houbenov, J. S. Haataja, H. Iatrou, N. Hadjichristidis, J. Ruokolainen, C. F. J. Faul and O. Ikkala, Angew. Chem., Int. Ed., 2011, 50, 2516–2520 CrossRef CAS PubMed
.
- B. M. Rosen, C. J. Wilson, D. A. Wilson, M. Peterca, M. R. Imam and V. Percec, Chem. Rev., 2009, 109, 6275–6540 CrossRef CAS PubMed
.
- T. Kato, Science, 2002, 295, 2414–2418 CrossRef CAS PubMed
.
- S. Chen and S. H. Eichhorn, Isr. J. Chem., 2012, 52, 830–843 CrossRef CAS
.
- C. F. J. Faul and M. Antonietti, Adv. Mater., 2003, 15, 673–683 CrossRef CAS
.
- B. R. Wiesenauer and D. L. Gin, Polym. J., 2012, 44, 461–468 CrossRef CAS
.
- D. L. Gin, C. S. Pecinovsky, J. E. Bara and R. L. Kerr, Struct. Bonding, 2008, 128, 181–222 CrossRef CAS
.
- M. Funahashi, H. Shimura, M. Yoshio and T. Kato, Struct. Bonding, 2008, 128, 151–180 CrossRef CAS
.
- S. Sergeyev, W. Pisula and Y. H. Geerts, Chem. Soc. Rev., 2007, 36, 1902–1929 RSC
.
- K. Binnemans, Chem. Rev., 2005, 105, 4148–4204 CrossRef CAS PubMed
.
- T. Kato, Angew. Chem., Int. Ed., 2010, 49, 7847–7848 CrossRef CAS PubMed
.
- I. M. Saez and R. J. W. Goodby, Struct. Bonding, 2008, 128, 1–62 CrossRef CAS
.
- C. Tschierske, Chem. Soc. Rev., 2007, 36, 1930–1970 RSC
.
- B. Donnio, S. Buathong, I. Bury and D. Guillon, Chem. Soc. Rev., 2007, 36, 1495–1513 RSC
.
- M. Marcos, R. Martın-Rapún, A. Omenat and J. L. Serrano, Chem. Soc. Rev., 2007, 36, 1889–1901 RSC
.
-
M. Yoshio and T. Kato, in Handbook of Liquid Crystals, ed. J. W. Goodby, P. J. Collings, T. Kato, C. Tschierske, H. Gleeson and P. Raynes, Wiley-VCH, Weinheim, 2nd edn, 2014, vol. 8, ch. 23, pp. 727–749 Search PubMed
.
- C. F. Van Nostrum and R. J. M. Nolte, Chem. Commun., 1996, 2385–2392 RSC
.
- Y. G. Zheng, J. G. Liu, Y. P. Liao, G. Ungar and P. V. Wright, Dalton Trans., 2004, 3053–3060 RSC
.
- U. Beginn, G. Zipp and M. Moller, Adv. Mater., 2000, 12, 510–511 CrossRef CAS
.
- N. Sakai and S. Matile, Langmuir, 2013, 29, 9031–9040 CrossRef CAS PubMed
.
- M. Armand and J. M. Tarascon, Nature, 2008, 451, 652–657 CrossRef CAS PubMed
.
- Y. B. Chen, M. Thorn, S. Christensen, C. Versek, A. Poe, R. C. Hayward, M. T. Tuominen and S. Thayumanavan, Nat. Chem., 2010, 2, 503–508 CrossRef CAS PubMed
.
- Y. Kim, W. Li, S. Shin and M. Lee, Acc. Chem. Res., 2013, 46, 2888–2897 CrossRef CAS PubMed
.
- B.-K. Cho, A. Jain, S. M. Gruner and U. Wiesner, Science, 2004, 305, 1598–1601 CrossRef CAS PubMed
.
- H.-K. Lee, H. Lee, Y. H. Ko, Y. J. Chang, N.-K. Oh, W.-C. Zin and K. Kim, Angew. Chem., Int. Ed., 2001, 40, 2669–2671 CrossRef CAS
.
- V. Percec, G. Johansson, J. Heck, G. Ungar and S. V. Batty, J. Chem. Soc., Perkin Trans. 1, 1993, 1411–1420 RSC
.
- R. L. Kerr, S. A. Miller, R. K. Shoemaker, B. J. Elliott and D. L. Gin, J. Am. Chem. Soc., 2009, 131, 15972 CrossRef CAS PubMed
.
- M. Yoshio, T. Mukai, K. Kanie, M. Yoshizawa, H. Ohno and T. Kato, Adv. Mater., 2002, 14, 351–354 CrossRef CAS
.
- M. Yoshio, T. Mukai, H. Ohno and T. Kato, J. Am. Chem. Soc., 2004, 126, 994–995 CrossRef CAS PubMed
.
- T. Ichikawa, M. Yoshio, A. Hamasaki, T. Mukai, H. Ohno and T. Kato, J. Am. Chem. Soc., 2007, 129, 10662–10663 CrossRef CAS PubMed
.
- M. Yoshio, T. Ichikawa, H. Shimura, T. Kagata, A. Hamasaki, T. Mukai, H. Ohno and T. Kato, Bull. Chem. Soc. Jpn., 2007, 80, 1836–1841 CrossRef CAS
.
- T. Ohtake, M. Ogasawara, K. Ito-Akita, N. Nishina, S. Ujiie, H. Ohno and T. Kato, Chem. Mater., 2000, 12, 782–789 CrossRef CAS
.
- P. H. J. Kouwer and T. M. Swager, J. Am. Chem. Soc., 2007, 129, 14042–14052 CrossRef CAS PubMed
.
- B.-K. Cho, RSC Adv., 2014, 4, 395–405 RSC
.
- K. Kishimoto, M. Yoshio, T. Mukai, M. Yoshizawa, H. Ohno and T. Kato, J. Am. Chem. Soc., 2003, 125, 3196–3197 CrossRef CAS PubMed
.
- H. Shimura, M. Yoshio, A. Hamasaki, T. Mukai, H. Ohno and T. Kato, Adv. Mater., 2009, 21, 1591–1594 CrossRef CAS
.
- X. Feng, M. E. Tousley, M. G. Cowan, B. R. Wiesenauer, S. Nejati, Y. Choo, R. D. Noble, M. Elimelech, D. L. Gin and C. O. Osuji, ACS Nano, 2014, 8, 11977–11986 CrossRef CAS PubMed
.
- B. Soberats, E. Uchida, M. Yoshio, J. Kagimoto, H. Ohno and T. Kato, J. Am. Chem. Soc., 2014, 136, 9552–9555 CrossRef CAS PubMed
.
- A. Yamashita, M. Yoshio, S. Shimizu, T. Ichikawa, H. Ohno and T. Kato, J. Polym. Sci., Part A: Polym. Chem., 2015, 53, 366–371 CrossRef CAS
.
- T. Ichikawa, M. Yoshio, A. Hamasaki, S. Taguchi, F. Liu, X. B. Zeng, G. Ungar, H. Ohno and T. Kato, J. Am. Chem. Soc., 2012, 134, 2634–2643 CrossRef CAS PubMed
.
- S. Kutsumizu, Isr. J. Chem., 2012, 52, 844–853 CrossRef CAS
.
- E. S. Hatakeyama, B. R. Wiesenauer, C. J. Gabriel, R. D. Noble and D. L. Gin, Chem. Mater., 2010, 22, 4525–4527 CrossRef CAS
.
- D. Högberg, B. Soberats, S. Uchida, L. Kloo, H. Segawa and T. Kato, Chem. Mater., 2014, 26, 6496–6502 CrossRef
.
- J. Sakuda, E. Hosono, M. Yoshio, T. Ichikawa, T. Matsumoto, H. Ohno, H. Zhou and T. Kato, Adv. Funct. Mater., 2015, 25, 1206–1212 CrossRef CAS
.
- R. D. Costa, F. Werner, X. J. Wang, P. Gronninger, S. Feihl, F. T. U. Kohler, P. Wasserscheid, S. Hibler, R. Beranek, K. Meyer and D. M. Guldi, Adv. Energy Mater., 2013, 3, 657–665 CrossRef CAS
.
- N. Yamanaka, R. Kawano, W. Kubo, N. Masaki, T. Kitamura, Y. Wada, M. Watanabe and S. Yanagida, J. Phys. Chem. B, 2007, 111, 4763–4769 CrossRef CAS PubMed
.
- T. Ichikawa, M. Yoshio, S. Taguchi, J. Kagimoto, H. Ohno and T. Kato, Chem. Sci., 2012, 3, 2001–2008 RSC
.
- B. Soberats, M. Yoshio, T. Ichikawa, S. Taguchi, H. Ohno and T. Kato, J. Am. Chem. Soc., 2013, 135, 15286–15289 CrossRef CAS PubMed
.
- T. Ichikawa, T. Kato and H. Ohno, J. Am. Chem. Soc., 2012, 134, 11354–11357 CrossRef CAS PubMed
.
- T. Matsumoto, T. Ichikawa, J. Sakuda, T. Kato and H. Ohno, Bull. Chem. Soc. Jpn., 2014, 87, 792–796 CrossRef CAS
.
- T. Ichikawa, M. Yoshio, A. Hamasaki, J. Kagimoto, H. Ohno and T. Kato, J. Am. Chem. Soc., 2011, 133, 2163–2169 CrossRef CAS PubMed
.
- W. J. Middleton and V. A. Engelhardt, J. Am. Chem. Soc., 1958, 80, 2788–2794 CrossRef CAS
.
- M.-L. Pujol-Fortin and J.-C. Galin, Macromolecules, 1991, 24, 4523–4530 CrossRef CAS
.
- M. Galin, A. Chapoton and J. C. Galin, J. Chem. Soc., Perkin Trans. 2, 1993, 545–553 RSC
.
- A. Mathis, M. Galin, J. C. Galin, B. Heinrich and C. G. Bazuin, Liq. Cryst., 1999, 26, 973–984 CrossRef CAS
.
- S. Ueda, J. Kagimoto, T. Ichikawa, T. Kato and H. Ohno, Adv. Mater., 2011, 23, 3071–3074 CrossRef CAS PubMed
.
- K. Xu, Chem. Rev., 2004, 104, 4303–4417 CrossRef CAS
.
- M. Yoshizawa, A. Narita and H. Ohno, Aust. J. Chem., 2004, 57, 139–144 CrossRef CAS
.
- J. C. Y. Lin, C.-J. Huang, Y.-T. Lee, K.-M. Lee and I. J. B. Lin, J. Mater. Chem., 2011, 21, 8110–8121 RSC
.
- R. Rondla, J. C. Y. Lin, C. T. Yang and I. J. B. Lin, Langmuir, 2013, 29, 11779–11785 CrossRef CAS PubMed
.
- M. Yoshizawa, M. Hirao, K. Ito-Akita and H. Ohno, J. Mater. Chem., 2001, 11, 1057–1062 RSC
.
- H. Lee, D. B. Kim, S.-H. Kim, H. S. Kim, S. J. Kim, D. K. Choi, Y. S. Kang and J. Won, Angew. Chem., Int. Ed., 2004, 43, 3053–3056 CrossRef CAS PubMed
.
- S. Sylla, J.-Y. Sanchez and M. Armand, Electrochim. Acta, 1992, 37, 1699–1701 CrossRef CAS
.
- D. R. MacFarlane, J. Huang and M. Forsyth, Nature, 1999, 402, 792–794 CrossRef CAS PubMed
.
Footnotes |
† Electronic supplementary information (ESI) available: Materials and methods, synthetic procedures, characterization of compounds, thermogravimetric analyses of the materials, liquid crystalline characterization experiments including XRD patterns and POM images, 7Li NMR studies, IR spectra and supplementary ion conduction measurements. See DOI: 10.1039/c5ta00814j |
‡ Mixtures containing more than 50 mol% of LiTFSI show no homogeneous LC phases. |
§ The wt% of PC is given with respect to the mass of the zwitterion. 5 and 10 wt% of PC is roughly equivalent to 22 and 45 mol% of PC with respect to the zwitterion. |
¶ Mixtures 1/Li+(x) and 2/Li+(x) show biphasic behaviour after the addition of 15 wt% or more of PC. Microscopy images of a mixture exhibiting biphasic behavior are shown in Fig. S2. |
|| Thermogravimetric analyses of PC-containing mixtures indicate that the evaporation of PC under an N2 flow starts below 100 °C. (Fig. S1). This evaporation is slower when the samples are sandwiched. |
|
This journal is © The Royal Society of Chemistry 2015 |
Click here to see how this site uses Cookies. View our privacy policy here.