DOI:
10.1039/C5SM01888A
(Paper)
Soft Matter, 2015,
11, 7656-7662
Supramolecular hydrogels constructed by red-light-responsive host–guest interactions for photo-controlled protein release in deep tissue†
Received
29th July 2015
, Accepted 16th August 2015
First published on 17th August 2015
Abstract
We report a novel red-light-responsive supramolecule. The tetra-ortho-methoxy-substituted azobenzene (mAzo) and β-cyclodextrin (β-CD) spontaneously formed a supramolecular complex. The substituted methoxy groups shifted the responsive wavelength of the azo group to the red light region, which is in the therapeutic window and desirable for biomedical applications. Red light induced the isomerization of mAzo and the disassembly of the mAzo/β-CD supramolecular complex. We synthesized a mAzo-functionalized polymer and a β-CD-functionalized polymer. Mixing the two polymers in an aqueous solution generated a supramolecular hydrogel. Red light irradiation induced a gel-to-sol transition as a result of the disassembly of the mAzo/β-CD complexes. Proteins were loaded in the hydrogel. Red light could control protein release from the hydrogel in tissue due to its deep penetration depth in tissue. We envision the use of red-light-responsive supramolecules for deep-tissue biomedical applications.
Introduction
Supramolecular interactions are non-covalent interactions between two or more molecules. Due to their moderate bond strength and reversibility, supramolecular interactions are usually responsive to external stimuli, such as light, pH, and redox.1 Supramolecular interactions can be used to construct stimuli-responsive materials for various applications.1 Photoresponsive supramolecular materials, for example, exhibit numerous biomedical applications, including drug delivery, photo-controlled protein adsorption and cell adhesion.2 Currently, most photoresponsive supramolecules efficiently absorb UV light and are thus controlled by UV light. However, UV light is problematic in biomedical applications. Compared with UV light, red and near-infrared (NIR) light in the therapeutic window (600–900 nm) are better suited for biomedical applications because they cause less photodamage and can penetrate deeper into tissue. Therefore, red- or NIR-light-responsive supramolecules are desirable for biomedical applications.
One approach to design red- or NIR-light-responsive supramolecules is based on simultaneous two-photon absorption. However, this method is inefficient even when high-intensity femtosecond lasers are used. Another approach to achieve red- or NIR-light-responsive supramolecules is by coupling UV-responsive supramolecules with upconverting nanoparticles. However, photon upconversion requires high-intensity lasers that may cause overheating problems and photodamage to biomaterials.3
Red-shifting the responsive wavelength of photoresponsive supramolecules is an approach to prepare intrinsic red- or NIR-light-responsive supramolecules. This approach should be more efficient than nonlinear optical processes such as two photon absorption and photon upconversion because red or NIR light are directly absorbed by supramolecules. To construct red- or NIR-light-responsive supramolecules, we may red-shift the responsive wavelength of UV-responsive supramolecules. It is well-known that trans azobenzene can spontaneously form supramolecular complexes with α- or β-cyclodextrin (CD).1a–j,2 UV irradiation can induce trans-to-cis isomerization of azobenzene, which leads to the disassembly of the supramolecular complexes.1a–j,2 Recently, new azo derivatives have been synthesized to red-shift the responsive wavelength of the azo group.4 In particular, some azo derivatives are responsive to red or NIR light.5 However, the feasibility of using the azo derivatives to construct red- or NIR-light-responsive supramolecules has not been explored. Moreover, no attention has been focused on constructing intrinsic red- or NIR-light-responsive supramolecules.
Here, we fabricate red-light-responsive supramolecules using tetra-ortho-methoxy-substituted azobenzene (mAzo) and β-CD (Scheme 1). Based on the red-light-responsive supramolecules, we prepared a responsive hydrogel that showed a gel-to-sol transition under red light irradiation. The hydrogel was used as a protein carrier. Red light was demonstrated to be able to control protein release from the hydrogel even in deep tissue. Compared with the conventional UV-responsive supramolecular interaction of azobenzene and CD, the new red-light-responsive supramolecular interaction reported in this paper is more suitable for biomedical applications.
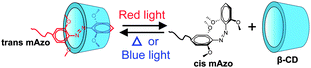 |
| Scheme 1 Schematic illustration of the supramolecular interaction between mAzo and β-CD. Red light irradiation triggers the disassembly of the mAzo/β-CD complex; blue light irradiation or heating triggers the reassembly of the complex. | |
Experimental section
Materials
2,6-Dimethoxyaniline, 12-aminododecanoic acid and β-cyclodextrin (β-CD) were purchased from Alfa Aesar. 3,5-Dimethoxyaniline, sodium nitrite, di-tert-butyl dicarbonate (BOC), triethylamine, 4-(dimethylamino)pyridine (DMAP), poly(acrylic acid) (PAA) (Mw = 450
000 g mol−1), 6-bromohexanoic acid, pyridine and α-cyclodextrin (α-CD) were purchased from Sigma Aldrich. Trifluoroacetic acid, N-(3-dimethylaminopropyl)-N′-ethylcarbodiimide hydrochloride (EDC) and (benzotriazol-1-yloxy) tripyrrolidinophosphonium hexafluorophosphate (PyBOP) were purchased from Carl Roth. 3A-amino-3A-deoxy-(2AS, 3AS)-β-cyclodextrin hydrate (NH2-β-CD) was purchased from TCI. The fluorescently labeled protein (Bovine Serum Albumin (BSA), Alexa Fluor® 680 conjugate) was purchased from Life Technologies. All the solvents (HPLC grade) were purchased from Sigma Aldrich and were used without further purification. Milli-Q water (resistivity: 18.2 MΩ cm) provided by a Sartorius Arium 611 VF Purification System was used throughout the research.
Methods
1H and 13C nuclear magnetic resonance (NMR) spectra were recorded on a Bruker Avance 250 MHz spectrometer and on a Bruker Avance III 700 MHz system. The 1H-NMR experiments were acquired with a 5 mm BBI z-gradient probe on the 700 MHz Bruker AVANCE III system. For a proton spectrum, 128 transients were used with a 10 μs long 90° pulse and a 12
600 Hz spectral width together with a recycling delay of 5 s. The 13C NMR (176 MHz) measurements were made with a J-modulated (coupling constant of 145 Hz 1H–13C was used) spin-echo for C-nuclei coupled to protons to determine the number of attached protons and proton decoupling during acquisition. The 90° pulse for carbon was 14.5 μs long and 16
000 scans were taken with a relaxation delay of 2 s. The temperature was regulated at 298.3 K and calibrated with a standard 1H methanol NMR sample using the topspin 3.2 software (Bruker). The control of the temperature was realized with a VTU (variable temperature unit) and an accuracy of ±0.1 K.
The assignment was accomplished by 1H, 1H 2D NOESY (nuclear Overhauser effect spectroscopy). The spectroscopic widths of the homo-nuclear NOESY experiments were typically 13
600 Hz in both dimension (f1 and f2) and the relaxation delay 2 s. The mixing time used in the 2D NOESY was kept at 350 ms.
The 2D 1H,13C-HSQC (heteronuclear single quantum correlations via double inept transfer and phase sensitive using Echo/Antiecho-TPPI gradient selection with decoupling during acquisition) and the 2D 1H,13C-HSQC-NOESY experiments were recorded with 2048 points in f2 and 256 points in f1 dimension. A NOESY mixing time of 350 ms were used for the HSQC-NOESY.
Before Fourier transformation, the data were zero filled to 1024 points in f1 and multiplied by a window function (q-sine bell or sine bell) in both dimension. The spectral widths were 30
000 Hz (236 ppm) for 13C and 9000 Hz (18 ppm) for 1H, both nuclei with a relaxation delay of 2.5 s. Proton and carbon spectra were referenced using the solvents signals (1H: HDO = 4.80 ppm or DMSO-d5H = 2.49 ppm and 13C: DMSO-d6 = 39.5 ppm) as an internal standard. The carbon spectra in D2O were calibrated against external TMS (tetramethylsilane) reference at 0 ppm.
Mass spectra (MS) were obtained using a VG instrument ZAB 2-SE-FPD. UV/vis absorption spectra were measured on a Lambda 900 spectrometer (Perkin Elmer). Fluorescence spectra were measured on a TIDAS II spectrometer (J&M).
Photoisomerization was induced by the LEDs with the wavelengths of 470 and 625 nm (device types LCS-0470-03-22 and LCS-0625-03-22, Mightex Systems). The output intensities of the LEDs were controlled by an LED controller (device type SLC-MA04-MU, Mightex Systems) and were calibrated by an optical powermeter (Model 407A, Spectra-Physics Corporation).
Synthesis
The syntheses of mAzo-NH2, mAzo-Py, PAA-mAzo and PAA-β-CD were shown in Scheme 2. In brief, mAzo-NH2 was synthesized by coupling 2,6-dimethoxyaniline and 3,5-dimethoxyaniline. To prepare water-soluble compounds with mAzo groups, mAzo-Py and PAA-mAzo were synthesized. PAA-β-CD were prepared by grafting NH2-β-CD on PAA. The details of syntheses and characterization of these compounds were provided in the ESI.†
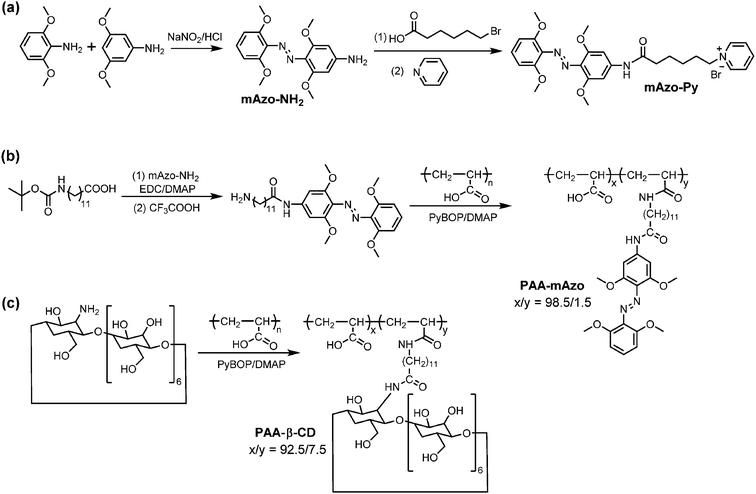 |
| Scheme 2 Route for the synthesis of mAzo-NH2, mAzo-Py, PAA-mAzo and PAA-β-CD. | |
Results and discussion
Photoresponse of tetra-ortho-methoxy-substituted azobenzene
We studied red-light-induced isomerization of mAzo-NH2 by 1H-NMR and UV/vis absorption spectroscopy (Fig. 1). The n–π* transition band of trans mAzo-NH2 extended to the red-light region and was separated from that of cis mAzo-NH2 (Fig. 1a and b). Thus, red light could excite trans mAzo-NH2 and induce trans-to-cis isomerization.4d,e,5a Approximately 85% of the trans isomers switched to cis isomers after 625 nm red light irradiation for 30 min (60 mW cm−2) (Fig. 1c). The cis mAzo-NH2 could change back to trans isomers by heating or blue light irradiation.
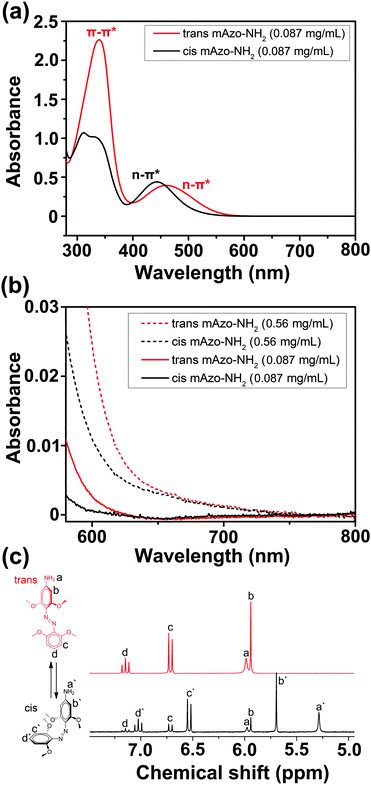 |
| Fig. 1 (a) UV/vis absorption spectra of mAzo-NH2 (0.087 mg mL−1 in DMSO) before and after the 625 nm red light irradiation (60 mW cm−2, 30 min). (b) Enlarged absorption spectra of mAzo-NH2 in the red-light region. mAzo-NH2 solutions with low (0.087 mg mL−1) and high (0.56 mg mL−1) concentrations were measured. The n–π* band of the trans isomer extended to the red-light region. (c) 1H-NMR spectra (250 MHz in DMSO-d6 at 298 K) of mAzo-NH2 before (red) and after (black) red light irradiation (625 nm, 60 mW cm−2, 30 min). | |
Red-light-responsive supramolecular interactions
To investigate the supramolecular interactions between mAzo and CDs in aqueous solutions, the hydrophilic compound mAzo-Py was synthesized (Scheme 2a, Fig. S1, ESI†). Red light could still induce isomerization of mAzo-Py in the presence of CDs (Fig. S5, ESI†). The supramolecular interactions between mAzo-Py and β-CD in D2O were studied by NMR spectroscopy (Fig. 2, Fig. S6 and S7, ESI†). In two-dimensional nuclear Overhauser effect spectroscopy (2D NOESY), the correlation between the protons of trans mAzo-Py (Proton 1 and 2) and the inner proton of β-CD (Proton f) demonstrated the host–guest interaction between trans mAzo-Py and β-CD (Fig. 2, left). Moreover, adding trans mAzo-Py to the solution of β-CD caused the shift of the signals of the inner protons of β-CD (Fig. S6, ESI†), suggesting that trans mAzo-Py entered the cavity of β-CD. Furthermore, the correlation between the carbons of trans mAzo-Py and the inner protons of β-CD also proved the host–guest interaction between trans mAzo-Py and β-CD (Fig. S7, ESI†). To study the interaction between cis mAzo-Py and β-CD, trans mAzo-Py was converted to cis mAzo-Py by red light irradiation. No dipole–dipole (2D NOESY) correlation between the protons of cis mAzo-Py (Proton 3 and 4) and the inner protons of β-CD indicated that red light irradiation triggered the disassembly of the host–guest complex (Fig. 2, right). Thus, the supramolecular interaction between mAzo-Py and β-CD was red-light-responsive.
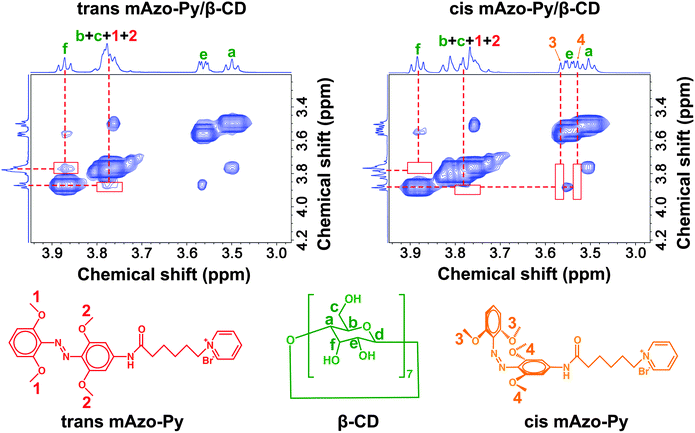 |
| Fig. 2 2D NOESY spectra (700 MHz in D2O at 298 K) of trans mAzo-Py/β-CD (left) and cis mAzo-Py/β-CD (right). Corresponding protons in the spectra are shown on the chemical structures of trans mAzo-Py, β-CD and cis mAzo-Py. The red rectangles in the spectra show whether there are correlation peaks between the protons of mAzo and the protons of β-CD. The concentration of β-CD is 1.67 mg mL−1. The molar ratio of mAzo-Py/β-CD is 1/1. | |
The association constants between the mAzo group and CDs were measured by 1H-NMR spectroscopy to quantify the supramolecular interactions using mAzo-Py or PAA-mAzo (Fig. S9–S13 and S16–S20, ESI†). The association constants between the normal azobenzene group and CDs were also measured for comparison using azobenzene-grafted PAA (PAA-Azo) (Fig. S21, ESI†). The association constants are listed in Table 1. For normal azobenzene, both α- and β-CDs had high association constants with the trans isomer and low association constants with the cis isomer. Thus, both the azobenzene/α-CD and azobenzene/β-CD supramolecules were UV-responsive. For mAzo, α-CD had low association constants with both the trans and cis isomers, indicating that mAzo could not form a stable host–guest complex with α-CD. β-CD had a high association constant with trans mAzo and a low association constant with cis mAzo. Therefore, the mAzo/β-CD supramolecule was red-light-responsive. The results in Table 1 show that the supramolecular interactions between mAzo and CDs were different from those between azobenzene and CDs. We interpreted that the differences in supramolecular interactions came from the different features of mAzo and azobenzene, such as the molecular sizes, shapes and hydrophilicities/hydrophobicities.
Table 1 Association constants (Ka) between α-CD or β-CD and the tetra-ortho-methoxy-substituted azobenzene (mAzo) group or the normal azobenzene groupa
Host |
Guest |
K
a (M−1) |
The details for measuring Ka are provided in the ESI (eqn (S1) and Fig. S16–S20). PAA-mAzo, PAA-Azo and α/β-CD were used to measure Ka.
|
α-CD |
trans mAzo |
145.2 |
α-CD |
cis mAzo |
66.9 |
α-CD |
trans Azobenzene |
1744.7 |
α-CD |
cis Azobenzene |
11.9 |
β-CD |
trans mAzo |
1546 |
β-CD |
cis mAzo |
82.1 |
β-CD |
trans Azobenzene |
892.1 |
β-CD |
cis Azobenzene |
363.4 |
Red-light-responsive supramolecular hydrogels
To demonstrate the potential applications of the mAzo/β-CD interaction in stimuli-responsive materials, we fabricated supramolecular hydrogels. The gelators PAA-mAzo and PAA-β-CD were synthesized by grafting PAA with mAzo and β-CD moieties, respectively (Scheme 2, Fig. S2 and S3, ESI†). Similar to mAzo-NH2 and mAzo-Py, PAA-mAzo showed red-light-induced isomerization (Fig. 3a). Red light induced trans-to-cis isomerization of PAA-mAzo because the n–π* band of the trans isomer extended to the red-light region. Both blue light and heating can induce cis-to-trans isomerization. Approximately 95% of trans PAA-mAzo was obtained after cis PAA-mAzo was irradiated by blue light for 1 min (60 mW cm−2). Approximately 100% of trans PAA-mAzo could be obtained after cis PAA-mAzo was heated at 60 °C for 10 h. The isomerization can be switched reversibly (Fig. 3b).
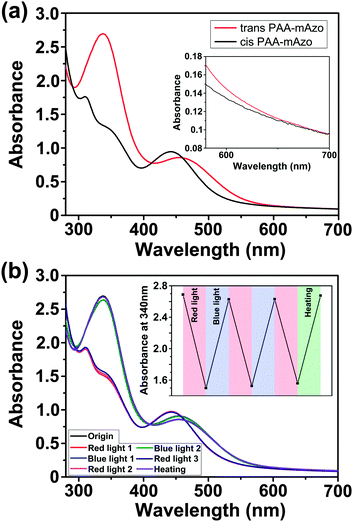 |
| Fig. 3 (a) UV-vis absorption spectra of PAA-mAzo (1.43 mg mL−1 in H2O) before and after red light irradiation (60 mW cm−2, 30 min). The inset shows the enlarged absorption spectra of PAA-mAzo in the red-light region. (b) UV-vis spectra of the PAA-mAzo after alternate red/blue light irradiation (60 mW cm−2, 30 min) and heating (60 °C, 10 h). The inset shows the changes of absorbance at 340 nm. | |
Supramolecular hydrogels were prepared by mixing trans PAA-mAzo and PAA-β-CD in PBS buffer in the dark (ESI†). Gelation occurred because the mAzo/β-CD supramolecular complexes cross-linked the polymers (Fig. 4a). Red light irradiation (625 nm, 60 mW cm−2, 30 min) triggered the disassembly of the supramolecular complexes and a gel-to-sol transition (Fig. 4b). The gel-to-sol transition was reversible. Blue light irradiation or heating could induce the sol-to-gel transition. Our red-light-responsive hydrogel is better suited for biomedical applications than the conventional UV-responsive hydrogel1n,o because red light can penetrate deeper into tissue and cause less photodamage to biomaterials. Thus, it pushes photoresponsive hydrogels one step further toward biomedical applications.
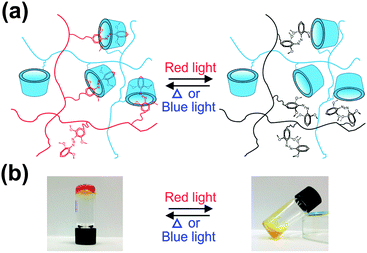 |
| Fig. 4 Schematic model (a) and photographs (b) of the reversible sol–gel transition of the PAA-mAzo/PAA-β-CD mixture. | |
Red-light-controlled protein release
The red-light-responsive supramolecular hydrogel was applied as a platform for controlled release of proteins (Scheme 3). Fluorescently labeled bovine serum albumin (BSA) was loaded in the supramolecular hydrogel (ESI†). Red light irradiation induced the collapse of the hydrogel and the release of BSA (Fig. 5a). The amounts of released BSA under different irradiation conditions were quantified by fluorescence spectroscopy (Fig. 5b, Fig. S22 and S23, ESI†). Only 9% BSA was released when the BSA-loaded hydrogel was kept in the dark for 60 min. Blue light irradiation (470 nm, 60 mW cm−2) for 60 min induced the release of only 15% BSA. Approximately 45% BSA was released after red light irradiation (625 nm, 15 mW cm−2) for 60 min. The release rate increased, and ∼83% BSA was released after higher-intensity (60 mW cm−2) red light irradiation for 60 min. We could also control the protein release kinetics “on-demand” via alternating red and blue light irradiation (Fig. 5c).
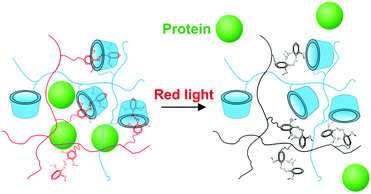 |
| Scheme 3 Schematic illustration of red-light-induced protein release from the PAA-mAzo/PAA-β-CD hydrogel. | |
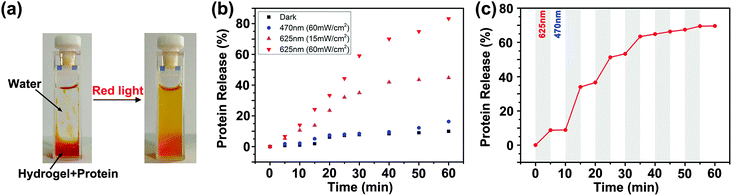 |
| Fig. 5 (a) Photographs of red-light-induced protein release from the PAA-mAzo/PAA-β-CD hydrogel. The BSA-loaded hydrogel at the bottom of the cuvette was irradiated by red light (625 nm, 60 mW cm−2) for 30 min. (b) Protein release profiles for BSA-loaded PAA-mAzo/PAA-β-CD hydrogel in the dark, upon blue light irradiation (470 nm, 60 mW cm−2) and red light irradiation (625 nm, 15 mW cm−2 or 60 mW cm−2). (c) “On-demand” release of BSA from the hydrogel by alternating red (625 nm, 60 mW cm−2) and blue light (470 nm, 60 mW cm−2) irradiation. | |
To test whether the supramolecular hydrogel maintained the red light responsiveness in deep tissue, a piece of pork tissue was placed between the light source and the sample (Fig. 6a). Red light (625 nm, 15 or 60 mW cm−2) still induced protein release after passing through the tissue (Fig. 6b). Importantly, the light intensity used in this experiment (15 and 60 mW cm−2) was an order of magnitude lower than the maximum permissible exposure of skin at 625 nm (200 mW cm−2).6 This light intensity was also much lower than that for upconverting-nanoparticle-assisted photochemistry.3 Therefore, photodamage to the tissue was prevented (Fig. S24, ESI†).
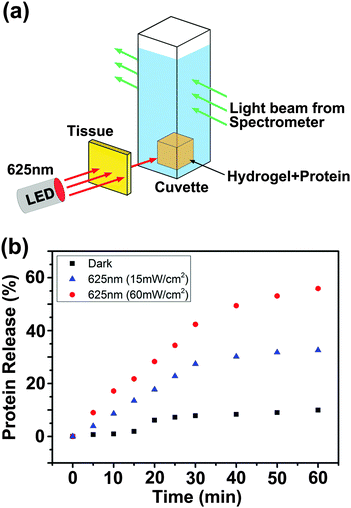 |
| Fig. 6 Experimental setup (a) and protein release profiles (b) when a 2 mm-thick tissue sample was placed between the light source and the BSA-loaded hydrogel. | |
Conclusions
In summary, we demonstrated the red-light-responsive supramolecular interaction between mAzo and β-CD. Supramolecular hydrogels were prepared by mixing the mAzo-functionalized polymer and the β-CD-functionalized polymer in an aqueous solution. Red light irradiation induced the disassembly of the mAzo/β-CD complexes and a gel-to-sol transition. The supramolecular hydrogel was used as a protein carrier. Red light could precisely control the protein release from the hydrogel. The red-light-responsive supramolecules exhibited several advantages over the conventional UV-responsive supramolecules1a–j,2 for biomedical applications: (i) they could be controlled in deep tissue; (ii) controlling the supramolecules using low-intensity red light prevented photodamage to biomaterials. We expect the mAzo/β-CD interaction to be useful not only for constructing hydrogels but also for constructing red-light-responsive supramolecular surfaces, polymers, colloids and macroscopic assemblies. Additionally, we expect that not only azo derivatives but also other visible- or NIR-light-responsive compounds7 should be suitable for constructing supramolecular assemblies via rational design. We envision using red- or NIR-light-responsive supramolecules for drug delivery, protein therapy, optogenetics, and control adhesion and migration of cells.
Acknowledgements
D. W. was supported by the CSC program. This work was partially supported by the Deutsche Forschungsgemeinschaft (DFG, WU 787/2-1).
Notes and references
-
(a)
Supramolecular Chemistry: Concepts and Perspectives, ed. J.-M. Lehn, Wiley-VCH Verlag GmbH & Co. KGaA, Weinheim, 2006 Search PubMed;
(b) M. Cheng, F. Shi, J. Li, Z. Lin, C. Jiang, M. Xiao, L. Zhang, W. Yang and T. Nishi, Adv. Mater., 2014, 26, 3009–3013 CrossRef CAS PubMed;
(c) M. Cheng, Q. Liu, Y. Xian and F. Shi, ACS Appl. Mater. Interfaces, 2014, 6, 7572–7578 CrossRef CAS PubMed;
(d) Y. Zhou, D. Wang, S. Huang, G. Auernhammer, Y. He, H.-J. Butt and S. Wu, Chem. Commun., 2015, 51, 2725–2727 RSC;
(e) D. Wang, D. Xie, W. Shi, S. Sun and C. Zhao, Langmuir, 2013, 29, 8311–8319 CrossRef CAS PubMed;
(f) Y. Takashima, S. Hatanaka, M. Otsubo, M. Nakahata, T. Kakuta, A. Hashidzume, H. Yamaguchi and A. Harada, Nat. Commun., 2012, 3, 1270 CrossRef PubMed;
(g) B. Zhang, Y. Liang, W. Yang, Y. Yang and L. Wu, Chem. Commun., 2014, 50, 10823–10826 RSC;
(h) L. Yue, H. Ai, Y. Yang, W. Lu and L. Wu, Chem. Commun., 2013, 49, 9770–9772 RSC;
(i) Q. Zhao, Y. Wang, Y. Yan and J. Huang, ACS Nano, 2014, 11, 11341–11349 CrossRef PubMed;
(j) L. Jiang, Y. Yan and J. Huang, Adv. Colloid Interface Sci., 2011, 169, 13–25 CrossRef CAS PubMed;
(k) L. Tan, H. Wu, M. Yang, C. Liu and R. Zhuo, RSC Adv., 2015, 5, 10393–10399 RSC;
(l) S. Wu, S. Duan, Z. Lei, W. Su, Z. Zhang, K. Wang and Q. Zhang, J. Mater. Chem., 2010, 20, 5202–5209 RSC;
(m) S. Wu and C. Bubeck, Macromolecules, 2013, 46, 3512–3518 CrossRef CAS;
(n) S. Tamesue, Y. Takashima, H. Yamaguchi, S. Shinkai and A. Harada, Angew. Chem., Int. Ed., 2010, 49, 7461–7464 CrossRef CAS PubMed;
(o) X. Liao, G. Chen, X. Liu, W. Chen, F. Chen and M. Jiang, Angew. Chem., Int. Ed., 2010, 49, 4409–4413 CrossRef CAS PubMed;
(p) J. Deng, X. Liu, W. Shi, C. Cheng, C. He and C. Zhao, ACS Macro Lett., 2014, 3, 1130–1133 CrossRef CAS.
-
(a) P. B. Wan, Y. P. Wang, Y. G. Jiang, H. P. Xu and X. Zhang, Adv. Mater., 2009, 21, 4362–4365 CrossRef CAS PubMed;
(b) A. Samanta, M. C. Stuart and B. J. Ravoo, J. Am. Chem. Soc., 2012, 134, 19909–19914 CrossRef CAS PubMed;
(c) Y.-H. Gong, J. Yang, F.-Y. Cao, J. Zhang, H. Cheng, R.-X. Zhuo and X.-Z. Zhang, J. Mater. Chem. B, 2013, 1, 2013–2017 RSC.
-
(a) S. He, K. Krippes, S. Ritz, Z. Chen, A. Best, H.-J. Butt, V. Mailänder and S. Wu, Chem. Commun., 2015, 51, 431–434 RSC;
(b) Z. Chen, S. He, H.-J. Butt and S. Wu, Adv. Mater., 2015, 27, 2203–2206 CrossRef CAS PubMed;
(c) Z. Chen, W. Sun, H.-J. Butt and S. Wu, Chem. – Eur. J., 2015, 21, 9165–9170 CrossRef CAS PubMed.
-
(a) R. Siewertsen, H. Neumann, B. Buchheim-Stehn, R. Herges, C. Näther, F. Renth and F. Temps, J. Am. Chem. Soc., 2009, 131, 15594–15595 CrossRef CAS PubMed;
(b) S. Venkataramani, U. Jana, M. Dommaschk, F. D. Sönnichsen, F. Tuczek and R. Herges, Science, 2011, 331, 445–448 CrossRef CAS PubMed;
(c) Y. Yang, R. P. Hughes and I. Aprahamian, J. Am. Chem. Soc., 2012, 134, 15221–15224 CrossRef CAS PubMed;
(d) A. A. Beharry, O. Sadovski and G. A. Woolley, J. Am. Chem. Soc., 2011, 133, 19684–19687 CrossRef CAS PubMed;
(e) D. Bléger, J. Schwarz, A. M. Brouwer and S. Hecht, J. Am. Chem. Soc., 2012, 134, 20597–20600 CrossRef PubMed.
-
(a) S. Samanta, A. A. Beharry, O. Sadovski, T. M. McCormick, A. Babalhavaeji, V. Tropepe and G. A. Woolley, J. Am. Chem. Soc., 2013, 135, 9777–9784 CrossRef CAS PubMed;
(b) Y. Yang, R. P. Hughes and I. Aprahamian, J. Am. Chem. Soc., 2014, 136, 13190–13193 CrossRef CAS PubMed.
-
(a)
American National Standard for Safe Use of Lasers, Laser Institute of America, Orlando, FL, 2000 Search PubMed;
(b)
Laser safety handbook, Northwestern University, Evanston, IL, 2011 Search PubMed.
-
(a) H. Kawai, T. Kanegae, S. Christensen, T. Kiyosue, Y. Sato, T. Imaizumi, A. Kadota and M. Wada, Nature, 2003, 421, 287–290 CrossRef CAS PubMed;
(b) A. Levskaya, O. D. Weiner, W. A. Lim and C. A. Voigt, Nature, 2009, 461, 997–1001 CrossRef CAS PubMed;
(c) Z. Shi, P. Peng, D. Strohecker and Y. Liao, J. Am. Chem. Soc., 2011, 133, 14699–14703 CrossRef CAS PubMed;
(d) V. K. Johns, P. Peng, J. Dejesus, Z. Wang and Y. Liao, Chem. – Eur. J., 2014, 20, 689–692 CrossRef CAS PubMed;
(e) Z. Wang, V. K. Johns and Y. Liao, Chem. – Eur. J., 2014, 20, 14637–14640 CrossRef CAS PubMed.
Footnote |
† Electronic supplementary information (ESI) available. See DOI: 10.1039/c5sm01888a |
|
This journal is © The Royal Society of Chemistry 2015 |