DOI:
10.1039/C4SC03865G
(Edge Article)
Chem. Sci., 2015,
6, 3724-3737
Bodipy–C60 triple hydrogen bonding assemblies as heavy atom-free triplet photosensitizers: preparation and study of the singlet/triplet energy transfer†
Received
13th December 2014
, Accepted 4th April 2015
First published on 9th April 2015
Abstract
Supramolecular triplet photosensitizers based on hydrogen bonding-mediated molecular assemblies were prepared. Three thymine-containing visible light-harvesting Bodipy derivatives (B-1, B-2 and B-3, which show absorption at 505 nm, 630 nm and 593 nm, respectively) were used as H-bonding modules, and 1,6-diaminopyridine-appended C60 was used as the complementary hydrogen bonding module (C-1), in which the C60 part acts as a spin converter for triplet formation. Visible light-harvesting antennae with methylated thymine were prepared as references (B-1-Me, B-2-Me and B-3-Me), which are unable to form strong H-bonds with C-1. Triple H-bonds are formed between each Bodipy antenna (B-1, B-2 and B-3) and the C60 module (C-1). The photophysical properties of the H-bonding assemblies and the reference non-hydrogen bond-forming mixtures were studied using steady state UV/vis absorption spectroscopy, fluorescence emission spectroscopy, electrochemical characterization, and nanosecond transient absorption spectroscopy. Singlet energy transfer from the Bodipy antenna to the C60 module was confirmed by fluorescence quenching studies. The intersystem crossing of the latter produced the triplet excited state. The nanosecond transient absorption spectroscopy showed that the triplet state is either localized on the C60 module (for assembly B-1·C-1), or on the styryl-Bodipy antenna (for assemblies B-2·C-1 and B-3·C-1). Intra-assembly forward–backward (ping-pong) singlet/triplet energy transfer was proposed. In contrast to the H-bonding assemblies, slow triplet energy transfer was observed for the non-hydrogen bonding mixtures. As a proof of concept, these supramolecular assemblies were used as triplet photosensitizers for triplet–triplet annihilation upconversion.
1. Introduction
Triplet photosensitizers (PSs) have attracted much attention due to their applications in photocatalysis,1–5 photodynamic therapy,6–8 and more recently triplet–triplet annihilation upconversion.9–12 Conventional triplet PSs include porphyrins, halogenated xanthanes such as Rose Bengal and methylene blue, and transition metal complexes such as Pt(II), Ru(II) or Ir(III) complexes, etc.13–15 Recently, halogenated Bodipys were developed as a new family of triplet PSs, due to their easily derivatized molecular structures, good photostability and strong absorption of visible light.7,16
There are a few challenges to be addressed in the molecular design of new triplet PSs. Firstly, most of the known triplet PSs contain heavy atoms to facilitate efficient intersystem crossing (ISC).7,14,16 However, for some chromophores, it is difficult to introduce heavy atoms into the molecular structure, and the heavy atom effect may become inefficient for chromophores with short S1 state lifetimes, or for bulky chromophores.13b,14 On the other hand, heavy atom-free organic triplet photosensitizers are difficult to ‘design’ because the ISC capability of such chromophores is almost unpredictable.14 Secondly, the known triplet PSs are based on an integrated molecular structure motif, i.e. the visible light-harvesting and the ISC are implemented by the same chromophore, or by covalently bonded chromophores in dyad/triad triplet PSs, for example the recently developed broadband visible light-absorbing organic triplet PSs.7,14 This approach is synthetically demanding if a library of chromophores is to be screened for the preparation of triplet PSs.5,17–19 Therefore, new methods are desired for the feasible preparation of organic triplet PSs.
Concerning this aspect, supramolecular assembly is particularly interesting due to the ability to fabricate molecular assemblies with different modules.20,21 For example, the supramolecular photochemistry of the hydrogen bonded C60–ferrocene dyad was studied.22a But the components showed weak absorption of visible light, thus the assembly was unsuitable for use as a triplet PS. Recently, a rotaxane was studied with fluorescence-resonance-energy-transfer (FRET), but the fluorophore present in the rotaxane was unable to produce any triplet excited states upon photoexcitation.22b
In order to address the above challenges in the area of triplet PSs, herein we propose using hydrogen bonded molecular assembly as a new approach for the design of organic triplet PSs.23–25 This method is also useful for designing heavy atom-free triplet PSs. One of the hydrogen bonding modules is used as a visible light-harvesting antenna and singlet energy donor, whereas the complementary H-bonding module is used as a singlet energy acceptor and a spin converter for triplet formation at the same time.14 The distance between the energy donor and acceptor can be controlled, and singlet energy transfer is assured. With this method, a wide variety of singlet energy donors can be feasibly screened, because the H-bonded molecular assembly is easy to obtain simply by mixing the H-bond donor and the H-bond acceptor in appropriate solvents, and the triplet formation upon photoexcitation can be feasibly evaluated by various spectroscopic methods. We confirmed this new molecular design concept with the preparation of H-bonded Bodipy–C60 assemblies. Efficient and fast intra-assembly singlet and triplet energy transfers were observed, based on steady state and nanosecond transient absorption spectroscopy. In contrast, much slower intermolecular triplet state energy transfer was observed for the reference modules with which no H-bonds could be formed. The application of the H-bond assemblies in singlet oxygen (1O2) photosensitization and triplet–triplet annihilation (TTA) upconversion was studied. This molecular design method will be useful for the study of organic triplet photosensitizers.
2. Results and discussions
2.1 Molecular design rationale
An N-acetyl-1,6-diaminopyridine-thymine based triple hydrogen bonding motif was used (Schemes 1–3). Thymine was connected to different Bodipy derivatives in order to act as the visible light-harvesting H-bonding module, i.e. the singlet energy donor. The N-acetyl-1,6-diaminopyridine unit was connected to C60 to form C-1 (Scheme 1), and acted as the singlet energy acceptor and spin converter for triplet formation.22a,26–29 Note that the visible light-absorbing ability of C60 is very weak,30 but it is a good singlet energy acceptor because of the low S1 state energy level (1.76 eV).31–37
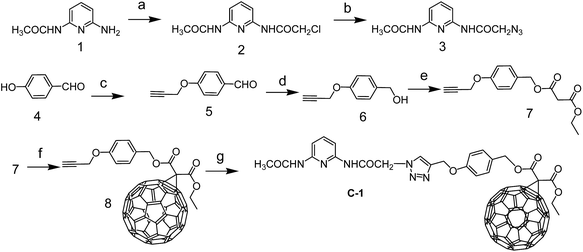 |
| Scheme 1 Preparation of the hydrogen bonding C60 module C-1. (a) 2-Chloro-acetyl chloride, dry CH2Cl2, Ar, 25 °C, 2 h; (b) NaN3, DMF, 70 °C, 5 h; (c) 3-bromo-1-propyne, potassium carbonate, ethanol, 79 °C, 6 h; (d) NaBH4, THF–CH3OH, rt, 5 min; (e) methyl malonyl chloride, CH2Cl2, Ar, 25 °C, 12 h; (f) iodine, C60, DBU, toluene, 10 h, Ar, 25 °C; (g) sodium ascorbate, CuSO4, anhydrous CHCl3–EtOH–water (12 : 1 : 1, v/v), rt, 12 h. | |
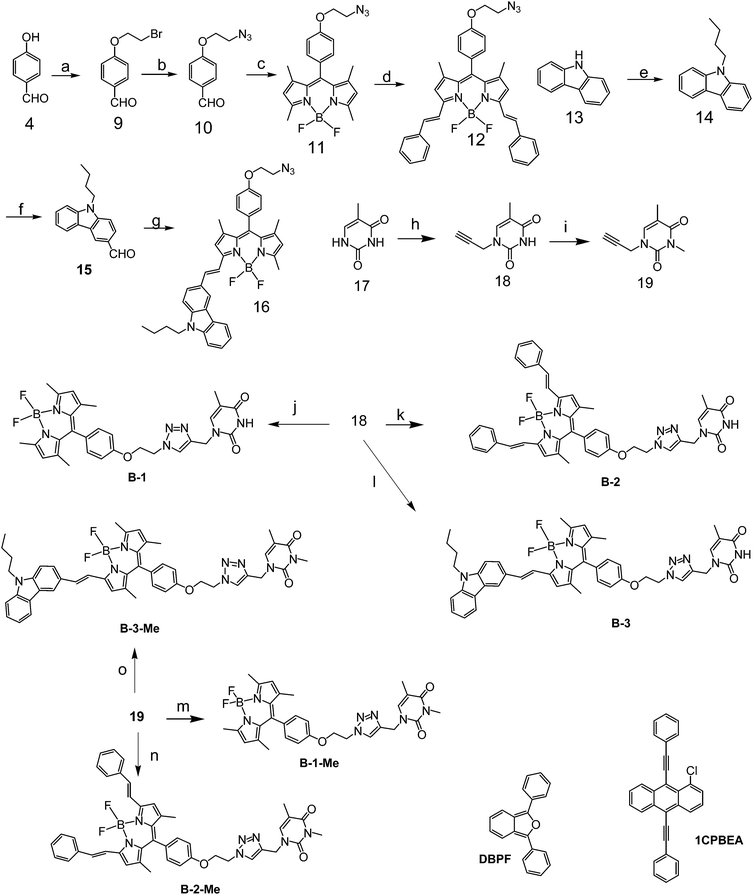 |
| Scheme 2 Preparation of the hydrogen bonding Bodipy modules B-1, B-2, and B-3, and the reference compounds which are unable to form strong hydrogen bonds with module C-1, i.e.B-1-Me, B-2-Me and B-3-Me. The singlet oxygen (1O2) scavenger DBPF and the triplet acceptor used in TTA upconversion, 1CPBEA, were also presented. (a) Anhydrous K2CO3, 1,2-dibromoethane, dry ethanol, Ar, 70 °C; (b) NaN3, DMF, 100 °C, 10 h; (c) 2,4-dimethylpyrrole, TFA, anhydrous CH2Cl2, rt, 12 h; DDQ, BF3·Et2O, 12 h; (d) benzaldehyde, acetic acid, piperidine, microwave irradiation, 8 min; (e) sodium hydride, n-butyl bromide, DMF, rt, 2 h; (f) DMF, POCl3, rt, 3 h, then 60 °C, 6 h; (g) 9-butyl-9H-carbazole-3-carbaldehyde, acetic acid, piperidine, microwave irradiation, 5 min; (h) propargyl bromide, K2CO3, DMF, rt, Ar, 10 h; (i) NaH, methyl iodide, rt, Ar, 12 h; (j) 18, sodium ascorbate, CuSO4, rt, Ar, 12 h; (k) 18, sodium ascorbate, CuSO4, rt, Ar, 12 h; (l) 18, sodium ascorbate, CuSO4, rt, Ar, 20 h; (m) 19, sodium ascorbate, CuSO4, rt, Ar, 12 h; (n) 19, sodium ascorbate, CuSO4, rt, Ar, 12 h; (o) 19, sodium ascorbate, CuSO4, rt, Ar, 24 h. | |
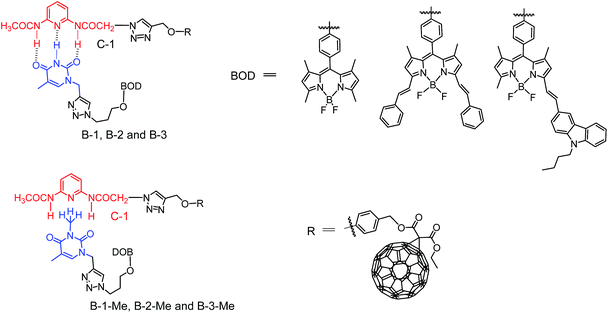 |
| Scheme 3 Structures of the hydrogen-bonding assemblies (C-1/B-1, B-2 and B-3, upper row), and the modules which are unable to form strong hydrogen bonds (C-1/B-1-Me, B-2-Me and B-3-Me, lower row). | |
Bodipy was selected as the visible light-harvesting antenna, due to its satisfactory photophysical properties and easily derivatized molecular structures.38–42 The Bodipy unit in B-1 shows absorption at ca. 505 nm, whereas in B-2 and B-3, the styryl-Bodipy unit shows absorption at 627 nm and 592 nm, respectively (Scheme 2).43 H-bonded assemblies with different absorption wavelengths can be easily prepared with B-1, B-2 or B-3 as the visible light-harvesting H-bonding module, and with C-1 as the singlet energy acceptor and spin converter (Scheme 3). In order to study the effect of H-bonding on the photophysical processes, singlet energy donors B-1-Me, B-2-Me and B-3-Me (Schemes 2 and 3), which are unable to form H-bonds with C-1, were prepared. These reference compounds contain a methyl group at the N-position of the thymine, and as a result the H-bonding with C-1 is substantially inhibited.27–29 All the compounds were prepared using routine synthetic methods and obtained in moderate to satisfactory yields. The molecular structures were fully characterized with 1H NMR, 13C NMR and HRMS.
2.2 UV/vis absorption and fluorescence spectra
The UV/vis absorption spectra of the compounds were studied (Fig. 1 and Table 1). C-1 shows the characteristic absorption of the C60 unit, which is centered at 331 nm. For the singlet energy donors, i.e. the Bodipy derivatives B-1, B-2 and B-3, the absorption bands are located at 505 nm, 630 nm and 593 nm, respectively. The S1 state energy level of C60 is 1.72 eV,30,34 which is lower than those of the visible light-harvesting energy donors (B-1, B-2 and B-3). The methylated visible light-harvesting compounds B-1-Me, B-2-Me and B-3-Me show similar absorption spectra to their analogues without methyl substitution on the N atom of thymine.
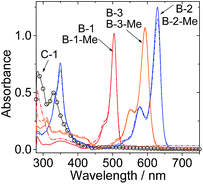 |
| Fig. 1 UV/vis absorption spectra of C-1, B-1, B-1-Me, B-2, B-2-Me, B-3 and B-3-Me (note that the absorptions of the methylated Bodipy modules are identical to those of the non-methylated Bodipy modules). c = 1.0 × 10−5 M in toluene, 20 °C. | |
Table 1 Photophysical parameters of the Bodipy and C60 hydrogen bonding modulesa
|
λ
abs
|
ε
|
λ
em
|
Φ
F (%) |
τ
F
(ns) |
In toluene (1.0 × 10−5 M).
Molar extinction coefficient at the absorption maximum (104 M−1 cm−1).
With 2,6-diiodo-4,4-difluoro-1,3,5,7-tetramethyl-8-phenyl-4-bora-3a,4a-diaza-s-indacene as the standard (Φ = 71.2% in acetonitrile).
With carbazole-styryl-Bodipy as the standard (Φ = 72.0% in toluene).
With carbazole-styryl-Bodipy as the standard (Φ = 63.0% in acetonitrile).
Fluorescence lifetimes.
Not determined.
|
B-1
|
505 |
10.2 |
517 |
75.7c |
3.5 |
B-1-Me
|
505 |
10.2 |
517 |
64.0c |
3.6 |
B-2
|
630 |
12.4 |
642 |
73.5d |
5.0 |
B-2-Me
|
630 |
11.5 |
642 |
65.4d |
4.9 |
B-3
|
593 |
10.6 |
611 |
70.3e |
3.7 |
B-3-Me
|
593 |
10.4 |
611 |
61.2e |
3.9 |
C-1
|
331 |
5.1 |
—g |
—g |
—g |
The UV/vis absorption in toluene of C-1/B-1, in which H-bonds have presumably been formed, is the sum of the UV/vis absorptions of B-1 and C-1 (Fig. 2a). This result indicated that H-bonding does not induce any interaction between the Bodipy and C60 moieties in the ground state.34 Similar results were observed for the mixture of B-1-Me and C-1, in which no H-bonding is expected (see ESI, Fig. S45†).27 This conclusion was supported by the titration of C-1 with higher B-1 concentrations (2 eq. vs.C-1, Fig. 2b).
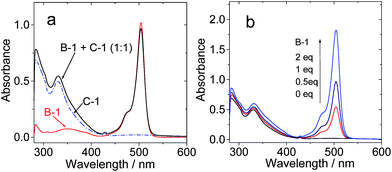 |
| Fig. 2 UV/vis absorption spectra of (a) B-1, C-1 and C-1/B-1 (1/1); (b) C-1 (1.0 × 10−5 M) with increasing concentrations of B-1. c = 1.0 × 10−5 M in toluene, 20 °C. | |
In order to verify the H-bonding between the Bodipy visible light-harvesting unit and C-1, fluorescent titration of B-1 with C-1 was carried out (Fig. 3). The rationale is that the fluorescence of B-1 will be quenched due to energy transfer (such as through space energy transfer) to C60 if H-bonds are formed with C-1.24,27,29,42bB-1 alone shows intense fluorescence at 517 nm (quantum yield ΦF = 75.7%). Upon addition of C-1 into a solution of B-1, however, the fluorescence intensity of B-1 was substantially quenched (Fig. 3a), and saturation was established after addition of 12 eq. C-1. The fluorescence intensity was reduced by 80%. The formation of hydrogen bonds between C-1 and B-1 is an equilibrium process. In order for most of the B-1 molecules to be H-bonded, i.e. in order to fully quench the fluorescence of B-1, excess C-1 has to be used. Interestingly, for B-1-Me, which is unable to form strong H-bonds with C-1 due to the methyl group on the N atom of the thymine moiety, the fluorescence was quenched to a much lesser extent, by 40% on addition of the same amount of C-1 (Fig. 3b). Therefore, we propose that strong H-bonds formed for C-1/B-1, but not for C-1/B-1-Me.27
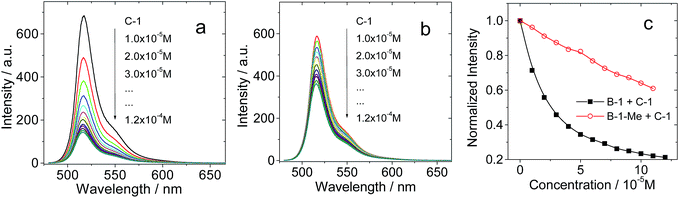 |
| Fig. 3 Quenching of the fluorescence of (a) B-1 and (b) B-1-Me by C-1. c = 1.0 × 10−5 M in toluene (λex = 475 nm). (c) Emission intensity of B-1 and B-1-Me at 517 nm (c = 1.0 × 10−5 M in toluene) versus concentration of C-1, following excitation at 475 nm at 20 °C. | |
Similar fluorescence quenching profiles were observed for B-2 and B-3 (see ESI, Fig. S51 and S53†). The fluorescence emission wavelength of B-3 is much longer than that of B-1. It should be pointed out that the S1 state energy level of B-3 (approximated as 2.04 eV) is still higher than that of C-1 (1.76 eV), thus intra-assembly singlet energy transfer is assured. For B-3-Me, no H-bonds can be formed, thus the quenching occurs to a much lesser extent (see ESI, Fig. S53b†).
The fluorescence lifetime of B-1 (or B-2, or B-3) was measured upon titration with C-1 (see ESI, Fig. S68†). The fluorescence lifetime is nearly constant (3.5–3.4 ns), despite the quenching of the fluorescence intensity with increasing C-1 concentration. Thus, we conclude that the fluorescence quenching is due to a mechanism similar to static quenching, not dynamic quenching,44,45 upon formation of the hydrogen bonds. That is, the fluorescence of the H-bonded assembly is completely quenched, while the un-bonded Bodipy fluorophore shows residual fluorescence.44 In other words, the H-bonding assembly becomes non-fluorescent, and the residual fluorescence observed during the titration is due to the free Bodipy modules in the solution. In order to verify that the fluorescence quenching during the titration is due to H-bond mediated assembly, hexafluoroisopropanol was added in order to disrupt the H-bonds. As a result, fluorescence recovery was observed (see ESI, Fig. S54†), which indicated that the fluorescence quenching was due to the formation of a molecular assembly via H-bonding.
These studies, using the visible light-harvesting chromophores B-1, B-2 and B-3 to form molecular assemblies with C-1, demonstrate the advantage of using H-bonding to induce intra-assembly energy transfer, that is, different combinations can be easily accessed and screened for efficient intramolecular energy transfer and formation of triplet states.
The stability constants of the visible light-harvesting Bodipys and C-1 were calculated based on the fluorescence titration experiments (Table 2).46,47 The stability constants for the H-bond forming combinations show that the bonding is strong. For the methylated Bodipy derivatives, however, the stability constants are 10-fold smaller.
Table 2 The stability constants for the interactions of the Bodipy H-bonding modules with the C-1 H-bonding module
|
B-1
|
B-1-Me
|
B-2
|
B-2-Me
|
B-3
|
B-3-Me
|
K
SV is the linear Stern–Volmer quenching constant.
K
b represents the intrinsic binding constant of the compound with C-1.
Not applicable.
|
K
SV
/M−1 |
4.08 × 104 |
3.02 × 103 |
3.67 × 104 |
1.22 × 103 |
5.14 × 104 |
5.43 × 103 |
K
b
/M−1 |
3.6 × 105 |
—c |
1.38 × 106 |
—c |
1.15 × 106 |
—c |
2.3 Electrochemical studies and the free energy changes of the intramolecular electron transfers
In order to study the intra-assembly photoinduced electron transfer, the electrochemical properties of the Bodipy and C60 modules were studied using cyclic voltammetry (Fig. 4).34,48,49 For B-1, a reversible reduction wave was observed at −1.32 V. A pseudo-reversible oxidation wave was observed at +1.11 V. Similar redox potentials were observed for B-1-Me. For B-2, a pseudo-reversible oxidation wave was observed at +0.84 V, which was shifted anodically as compared with B-1. For the carbazole-containing B-3, two reversible oxidation waves at +0.74 and +1.03 V were observed, which were different from B-1 and B-2. The two reversible reduction waves of B-3 and B-3-Me are due to the carbazole-styryl-Bodipy moiety, which was confirmed by studying a reference compound (see ESI, Fig. S67†). For the C60 module, i.e.C-1, two reversible reduction waves at −0.7 V and −1.08 V were observed (Fig. 5), as well as a pseudo-reversible reduction wave at −1.53 V. These reduction waves are attributed to the C60 moiety.
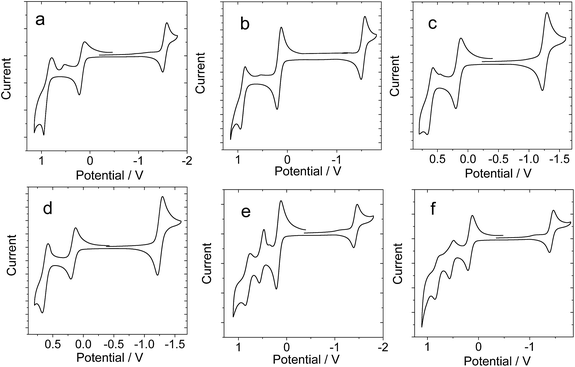 |
| Fig. 4 Cyclic voltammograms of the Bodipy H-bonding modules. Ferrocene (Fc) was used as an internal reference (E1/2 = +0.38 V (Fc+/Fc) vs. SCE). (a) B-1, (b) B-1-Me, (c) B-2, (d) B-2-Me, (e) B-3, and (f) B-3-Me. Experiments were carried out in deaerated DCM solutions containing 0.5 mM photosensitizer, alone or with ferrocene, 0.10 M Bu4NPF6 as the supporting electrolyte, and a Ag/AgNO3 reference electrode; scan rate: 0.05 V s−1. | |
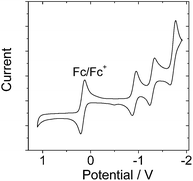 |
| Fig. 5 Cyclic voltammogram of C-1. Ferrocene (Fc) was used as an internal reference (E1/2 = +0.38 V (Fc+/Fc) vs. SCE). Experiments were carried out in deaerated DCM solutions containing 0.5 mM C-1 alone, 0.10 M Bu4NPF6 as the supporting electrolyte, and a Ag/AgNO3 reference electrode; scan rate: 0.05 V s−1. | |
The plausible photoinduced intra-assembly electron transfer between the Bodipy and the C60 module can be evaluated with the Gibbs free energy changes, which are calculated with the Rehm–Weller equation,34,48,49
|  | (1) |
|  | (2) |
where Δ
GS is the static Coulombic energy, which is described by
eqn (2).
e is the electronic charge,
EOX is the half-wave potential for one-electron oxidation of the electron-donor unit,
ERED = half-wave potential for one-electron reduction of the electron acceptor unit,
E00 is the approximate energy level obtained from the fluorescence emission (for the singlet excited state),
εS = static dielectric constant of the solvent, and
RCC = center-to-center separation distance between the electron donor (Bodipy module) and the electron acceptor (
C-1 module). As determined by DFT optimization of the geometries,
RCC (
B-1·C-1) = 40.0 Å,
RCC (
B-2·C-1) = 43.9 Å and
RCC (
B-1·C-1) = 49.5 Å.
RD is the radius of the electron donor,
RA is the radius of the electron acceptor,
εREF is the static dielectric constant of the solvent used for the electrochemical studies, and
ε0 is the permittivity of free space. The solvents used in the calculation of the free energy of the electron transfer are toluene (
εS = 2.4) and CH
2Cl
2 (
εS = 8.93). The electrochemical data and the free energy changes of the photoinduced electron transfers in the H-bonding assemblies are summarized in
Table 3. The results show that intra-assembly photoinduced electron transfer is thermodynamically prohibited. Therefore, the fluorescence quenching effect observed during the H-bonding titration (
Fig. 3) is due to intra-assembly energy transfer, not electron transfer.
Table 3 Redox potentials of the H-bond modules and the free energy changes (ΔGCS) for the intramolecular electron transfers (with the Bodipy unit as electron donor and the C60 unit as electron acceptor). Anodic and cathodic peak potentials are presented. The potential values of the compounds are vs. Ag/AgNO3 reference electrode, with Fc as internal reference (E1/2(Fc+/Fc) = +0.38 V)a
|
E
OX (V) |
E
RED (V) |
ΔGCS (eV) |
Cyclic voltammetry carried out in Ar saturated acetonitrile containing 0.10 M Bu4NPF6 supporting electrolyte; counter electrode was a Pt electrode; working electrode was a glassy carbon electrode; the Ag/AgNO3 couple was used as the reference electrode. c[Ag+] = 0.1 M. Conditions: 0.5 mM dyad photosensitizers and 0.5 mM ferrocene in CH2Cl2, 293 K.
With reference C-1 as electron acceptor, in CH2Cl2.
With reference C-1 as electron acceptor, in toluene.
No reduction potentials were observed.
No ΔGcs values were calculated.
|
B-1
|
+1.11 |
−1.32 |
+0.21,b 1.17c |
B-1-Me
|
+1.12 |
−1.31 |
+0.25,b 1.33c |
B-2
|
+0.84 |
−1.04 |
+0.28,b 0.92c |
B-2-Me
|
+0.84 |
−1.04 |
+0.32,b 1.06c |
B-3
|
+0.74/+1.03 |
−1.21 |
+0.36,b 0.97c |
B-3-Me
|
+0.74/+1.03 |
−1.22 |
+0.40,b 1.14c |
C-1
|
—d |
−0.70/−1.08/−1.53 |
—e |
2.4 Nanosecond transient absorption spectroscopy: intra-assembly and intermolecular triplet state energy transfer
The formation of H-bonds between the Bodipy visible light-harvesting chromophores and the fullerene module C-1 will induce singlet energy transfer from the Bodipy part to the C60 part.34,49 As a result, a triplet excited state will be produced via the inherent ISC of C60.35–37 Thus, nanosecond transient absorption spectroscopy was used to confirm the production of the triplet state via H-bonding mediated intra-assembly energy transfer.22a Indeed, we observed the triplet state of C-1 upon photoexcitation (no signals could be detected in aerated solution, see ESI, Fig. S65†).
First, C-1 was titrated with B-1 (Fig. 6). The mixed solution was photoexcited at 495 nm with a nanosecond pulsed laser. The absorption of C-1 at 495 nm is weak. Thus, selective excitation of B-1 in the mixture is possible. On increasing the concentration of B-1, the excited state absorption (ESA) of the C60 part at 715 nm was enhanced (indicated by the ΔO.D. values, which are proportional to the population of the transient species, Fig. 6f), indicating enhanced singlet energy transfer and finally the production of the triplet state with increasing concentration of B-1 (Fig. 6d). The presence of the triplet state was confirmed by measurement of the transient signal in aerated solution (no signal was observed, see ESI, Fig. S65 and S66†). It should be pointed out that the triplet excited state is localized on the C60 moiety, not on the Bodipy moiety, because the ground state bleaching band at 505 nm (at which the Bodipy unit shows steady state absorption) was not observed. This is in agreement with our previous studies of C60–Bodipy dyads.35 The triplet state lifetimes are in the range of 40.9–44.2 μs, which supports the localization of the triplet state on the C60 moiety.30 In comparison, excitation of B-1 alone didn’t produce any triplet state (see ESI, Fig. S62a†); thus, the cascade singlet energy transfer and ISC only occur in the B-1·C-1 assembly.
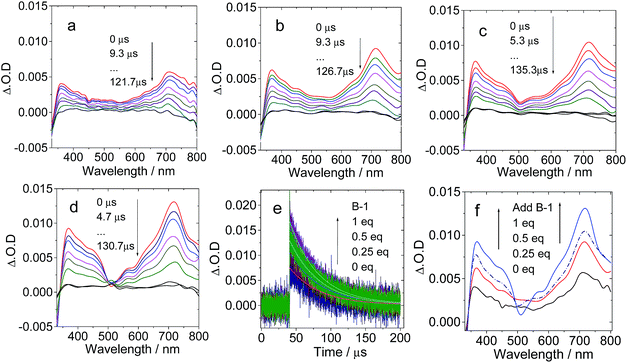 |
| Fig. 6 Nanosecond transient absorption spectra of C-1 on addition of increasing amounts of compound B-1. The transient signal intensified with increasing B-1 concentration: (a) 0 eq. B-1; (b) 0.25 eq. B-1; (c) 0.5 eq. B-1; and (d) 1 eq. B-1. (e) Decay trace at 720 nm; (f) comparison of the transient absorption spectra at different B-1 concentrations with delay time of 0 μs. c = 1.0 × 10−5 M; spectra were recorded in toluene after pulsed excitation at 495 nm under N2 at 20 °C. | |
The triplet state quantum yields of C-1 and the B-1·C-1 hydrogen bonding assembly (1
:
1) were determined as 86.1% and 67.5%, respectively, using the triplet energy transfer method, β-carotene as triplet energy acceptor, and Ru(bpy)3Cl2 as a reference (see Experimental section for details).16b,c
The triplet state of C-1 upon titration with B-1-Me was also studied (see ESI, Fig. S56†). In this situation, no strong H-bonding is expected.27 Intermolecular singlet energy transfer is unlikely for two compounds mechanically mixed in solution (the distance between the energy donor and the energy acceptor can be treated as infinite, well exceeding the distance required for the Förster energy transfer). We did not observe any increase in the transient absorption of the T1 state of C60 on increasing the concentration of B-1-Me (see ESI, Fig. S56†). Thus, the intra-assembly singlet energy transfer was confirmed by studying this reference compound.
Previously, we demonstrated that for a Bodipy–C60 covalent dyad, intramolecular ping-pong energy transfer is possible, i.e. forward singlet energy transfer to the C60 unit, and backward triplet energy transfer to the styryl-Bodipy visible light-harvesting chromophore.36,37 Herein, we studied this photophysical process in the H-bonding mediated supramolecular assemblies. To this end, B-1 is not a good candidate because the T1 state energy level of C60 is lower than that of the Bodipy unit in B-1, therefore no backward triplet state energy transfer is expected, because the upward triplet energy transfer from C60 to the Bodipy unit in B-1 is thermodynamically prohibited. Instead, we selected B-3 for this study, because we have shown that the T1 state of the styryl-Bodipy–C60 dyad/triad is localized on the styryl-Bodipy moiety, not on the C60 unit. That is, the styryl-Bodipy antenna has a lower T1 state energy level than C60.
For B-3, the excitation wavelength is 590 nm, at which the C60 unit shows weak absorption. On addition of B-3 into a solution of C-1, a bleaching band at 590 nm was observed, which could be assigned to the depletion of the ground state of the carbazole-styryl-Bodipy in B-3 (Fig. 7b–d). ESA of the T1 state (T1 → Tn transitions) of the styryl-Bodipy antenna was observed in the range of 600–750 nm.35 Thus, we propose that ping-pong energy transfer occurs in B-3·C-1. First, B-3 is photoexcited with a 590 nm laser, and then intra-assembly singlet energy transfer from the carbazole-styryl-Bodipy to the H-bonded C60 moiety occurs. The ISC of C60 produces a triplet excited state, followed by the backward triplet energy transfer from C60 to the carbazole-styryl-Bodipy module; as a result, the ground state bleaching band and the ESA of the styryl-Bodipy part in B-3 were observed.
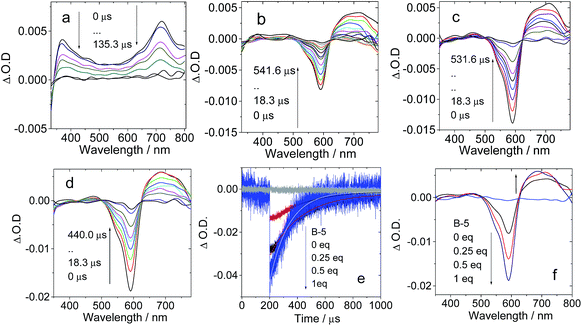 |
| Fig. 7 Nanosecond transient absorption spectra of the C-1 H-bonding module upon titration with the complementary B-3 H-bonding module. Transient absorption of C-1 in the presence of (a) 0 eq. B-3, (b) 0.25 eq. B-3, (c) 0.5 eq. B-3, and (d) 1 eq. B-3. (e) Decay trace at 593 nm; (f) transient spectra extracted from (a)–(d) at delay times of 0 μs. c = 1.0 × 10−5 M; spectra were recorded in toluene after pulsed excitation at 590 nm under N2 at 20 °C. | |
With increasing B-3 concentration, the ΔO.D. magnitude of B-3 increased, indicating enhanced visible light-harvesting, ping-pong singlet–triplet energy transfer in the assembly, and finally the production of the triplet excited state of the antenna (Fig. 7f). When 0.25 eq. to 1 eq. B-3 was added, the triplet state lifetime decreased from 263.2 μs to 138.9 μs. The self-quenching constant is 4.6 × 105 M−1 s−1. This quenching effect is due to the TTA process, which is significant for transient species with long triplet state lifetimes. Similar fast intra-assembly triplet–triplet electron transfer (TTET) was observed for B-2·C-1 (see ESI, Fig. S58†).
No strong H-bonds should be formed in B-3-Me/C-1. The nanosecond transient absorption spectroscopy of B-3-Me in the presence of C-1 was also studied (Fig. 8). Drastically different results were observed, as compared with B-3/C-1. The bleaching band of the carbazole-styryl-Bodipy moiety was observed at 593 nm (Fig. 8b). Since C60 was excited at 425 nm, and excitation of the solution of B-3-Me alone did not produce any triplet excited state (see ESI, Fig. S64b†), the population of the T1 state of the styryl-Bodipy moiety in B-3-Me is attributed to intermolecular triplet energy transfer, with C60 acting as the triplet energy donor.50
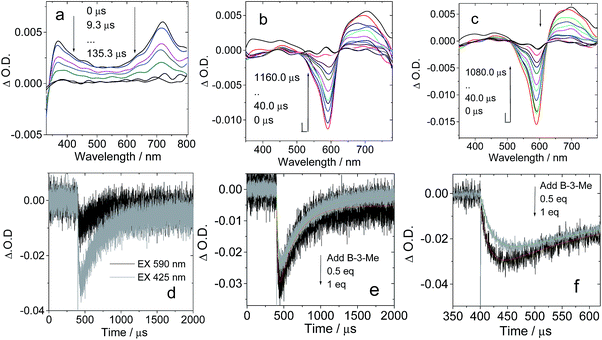 |
| Fig. 8 Nanosecond transient absorption spectra of H-bonding module C-1 upon titration with the non-preferred H-bonding module B-3-Me (no strong H-bonds can be formed with C-1). Transient absorption spectra of C-1 in the presence of (a) 0 eq. B-3-Me, (b) 0.5 eq. B-3-Me and (c) 1 eq. B-3-Me. (d) Decay traces at 630 nm on addition of B-3-Me with pulsed excitation at 425 nm (selective excitation of the C60 moiety) and 590 nm (selective excitation of the carbazole-styryl-Bodipy moiety). (e) Decay traces at 630 nm on addition of 0.5 eq. and 1 eq. B-3-Me. c = 1.0 × 10−5 M for C-1 in toluene after pulsed excitation at 425 nm under N2 at 20 °C (note that for the sake of signal intensity, the excitation is at 425 nm instead of 590 nm, which was used for Fig. 7). (f) Magnification of the decay traces in (e). | |
The evidence for this intermolecular energy transfer, rather than a fast intra-assembly energy transfer, is provided by the biphasic features of the decay traces of the transient absorption monitored at 630 nm (Fig. 8e and f), in which the first phase of the increase in intensity of the bleaching band at 593 nm is due to the intermolecular triplet energy transfer from the C60 moiety to the styryl-Bodipy moiety in B-3-Me, which takes ca. 15 μs. This process is the relatively slow intermolecular TTET as compared to the fast intra-assembly TTET (Fig. 7e), for which the TTET cannot be resolved with the transient absorption spectrometer (resolution of the spectrometer is 10 ns). The later recovery phase is due to decay of the T1 state of the styryl-Bodipy moiety. The lack of formation of an H-bonded B-3-Me–C-1 molecular assembly was confirmed by the ΔO.D. values of the transient absorption upon 425 nm and 590 nm photoexcitation (Fig. 8d). The ΔO.D. value of the transient absorption upon excitation at 590 nm (where the styryl-Bodipy antenna gives strong absorption) is less than that observed with 425 nm excitation, and no slow increase in the intensity of the bleaching band was observed upon 590 nm photoexcitation. This result confirmed that there is no significant formation of a H-bond mediated molecular assembly.
For B-2-Me, a slow evolution of the transient bleaching was also observed (see ESI, Fig. S61†). The triplet state energy transfer takes about 16 μs. This slow process is in stark contrast to that observed for the H-bonding assemblies of B-2·C-1 and B-3·C-1, for which no slowly increasing phases in the transient signals were observed. Thus, the intra-assembly (in other words the intramolecular) triplet energy transfer occurs with rate constant kTTET > 108 s−1 (the time-resolution of the spectrometer is 10 ns). This is an interesting result, since for the C-1·B-2 and C-1·B-3 assemblies, the distance between the C60 and the styryl-Bodipy part is ca. 5.0 nm, and the linker contains a saturated bond, yet the triplet energy transfer is fast.33,51 We propose movement of the Bodipy module and the C60 module; thus intramolecular collision causes the fast intra-assembly triplet state energy transfer to occur.
Previously, intra-assembly triplet energy transfer from C60 to ferrocene was observed in a hydrogen bonded C60 ferrocene dyad (k = 9.2 × 105 s−1).22a A steroid-linked norbornadiene–carbazole dyad showed intramolecular triplet energy transfer with a rate constant of 3.3 × 105 s−1 (from the carbazole to the norbornadiene moiety).52 For the hydrogen bonded assemblies B-2·C-1 and B-3·C-1, the intramolecular triplet energy transfer from the C60 moiety to the styryl-Bodipy moiety is much faster (k > 108 s−1). Triplet energy transfer is very often believed to occur via the Dexter electron exchange mechanism, which requires close contact between the energy donor and the energy acceptor. The triplet energy transfer will be negligible if the distance between the energy donor and acceptor exceeds the sum of their van der Waals radii. We propose that the flexible chains in the B-2·C-1 and B-3·C-1 assemblies allow direct contact of the styryl-Bodipy and the C60 moiety through foldamers; thus the triplet energy transfer is greatly accelerated. Previously, hydrogen bonding porphyrin–zinc assemblies were studied, and 50–80 times faster singlet–singlet energy transfer was observed for the assemblies with flexible linkers, as compared to those with rigid linkers, such as steroids.53
2.5 Molecular dynamics (MD) simulations: explanation of the fast intramolecular triplet energy transfer
The possible conformations of the complexes containing a hydrogen acceptor and donor were explored by molecular dynamics (MD) simulations performed in aqueous solution (see ESI†).53 It was found that a relatively stable complex can be formed in less than 10 ns, indicating that recognition of the hydrogen donor and acceptor in aqueous solution is quite feasible. Note that the three pairs of hydrogen bonds in the initial conformation are largely preserved in the compact conformation, although B-3 prefers to assume an extended conformation in solution. Weak π–π interactions between part of the B-3 structure and the fullerene motifs of the C-1 molecules may further contribute to the stability of this complex. These results indicate that fast intra-assembly TTET is possible, which can be used to rationalize the nanosecond transient absorption spectra of the B-3·C-1 H-bond assembly (Fig. 9).
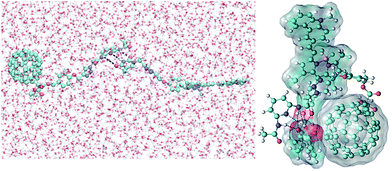 |
| Fig. 9 Right: conformation of the B-3·C-1 complex in aqueous solution obtained from an MD simulation at 6.9 ns, showing the hydrogen bonds (<3 Å as indicated using black dotted lines) between B-3 and the C-1 motif. Left: the starting extended conformation used in the MD simulations. The white surfaces represent the solvent (water molecules) accessible surface areas of B-3 and the fullerene motif of the C-1 structure. | |
2.6 Hydrogen bonded Bodipy–C60 assemblies as singlet oxygen (1O2) photosensitizers
The production of triplet excited states by the hydrogen bonded C60–Bodipy assemblies was also studied using singlet oxygen (1O2) photosensitization (Fig. 10). 1,3-Diphenylbenzofuran (DPBF) was used as 1O2 scavenger. The production of 1O2 could be monitored by following the absorbance changes of DPBF at 414 nm.
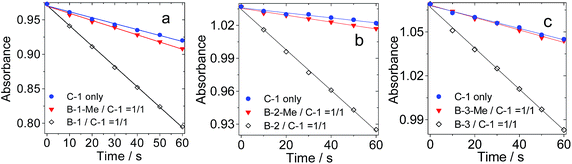 |
| Fig. 10 Singlet oxygen (1O2) generation by photoexcitation of C-1, B-1, B-1-Me, B-2, B-2-Me, B-3 and B-3-Me. Hydrogen bonded pairs produced 1O2 more efficiently. Decrease in absorbance of DPBF vs. photoirradiation time in the presence of photosensitizers: (a) λex = 495 nm, 1.1 mW m−2; (b) λex = 630 nm, 1.3 mW m−2; (c) λex = 588 nm, 1.3 mW m−2; c = 1.0 × 10−5 M in toluene, 20 °C. | |
For all the hydrogen bond assemblies, i.e.B-1·C-1, B-2·C-1 and B-3·C-1, the 1O2 photosensitizing ability is higher than the combinations for which strong hydrogen bonds are unable to form, i.e.B-1-Me·C-1, B-2-Me·C-2, and B-3-Me·C-1. These pairs show similar 1O2 photosensitizing ability to C-1 alone. These results clearly demonstrate the effect of hydrogen bonding on intra-assembly singlet energy transfer.
2.7 Application in triplet–triplet annihilation upconversion
Up until now, no supramolecular triplet photosensitizers for TTA upconversion have been reported. The H-bonding assemblies were used as supramolecular triplet photosensitizers for TTA upconversion, with 1-chloro-9,10-bisphenylethynylanthracene (1-CBPEA) as triplet acceptor/emitter (Fig. 11; note that for B-3 and B-3-Me, the fluorescence emission shows vibrational progression, i.e. there are two emission bands at 616 nm and 657 nm. Actually, there should be no peak at 550 nm; this peak is due to the filter because the transmittance is greatly reduced beyond 570 nm; thus the tailing of the emission band gives a fake ‘peak’ at 550 nm). For B-3·C-1, upconverted emission in the 450–550 nm region was observed with increasing C-1 concentration (Fig. 11a). For B-3-Me, which is unable to form a H-bonded molecular assembly, no TTA upconversion in the region of 450–550 nm was observed. Thus, we confirm that the triplet state production by B-3·C-1 upon photoexcitation is sufficient for TTA upconversion. For the B-3-Me–C-1 mixture, however, no upconversion was observed due to the lack of H-bonding. To the best of our knowledge, this is the first time that a supramolecular triplet photosensitizer was used for TTA upconversion. This result opens a new avenue for studies on TTA upconversion.
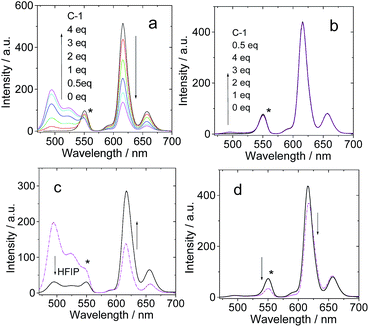 |
| Fig. 11 TTA upconversion of C-1 with B-3 and B-3-Me H-bonding modules. 1-CBPEA was used as triplet acceptor. (a) Emission of B-3 and 1-CBPEA with increasing C-1 concentration; (b) emission of B-3-Me and 1-CBPEA with increasing C-1 concentration; (c) emission observed when 0 μL and 100 μL of hexafluoroisopropanol (HFIP) were added to a mixed solution of B-3, 1-CBPEA and 4 eq. C-1 to disrupt the H-bonding; (d) emission observed when 0 μL and 100 μL of HFIP were added to a mixed solution of B-3-Me, 1-CBPEA and 4 eq. C-1. Excitation was carried out with a 589 nm laser (4.4 mW, power density is 60 mW cm−2), and a band-pass filter (470–570 nm) was used to suppress the scattered laser. The asterisks indicate the residual emission of the antenna. c[photosensitizers] = 1.0 × 10−5 M. c[1-CBPEA] = 1.2 × 10−4 M in deaerated toluene, 20 °C. | |
In order to prove that the production of the triplet excited state and the TTA upconversion are due to the formation of the H-bonded molecular assembly, hexafluoroisopropanol (HFIP) was added to disrupt the H-bonding (Fig. 11c). As a result, the TTA upconversion intensity decreased, and at the same time, the fluorescence of the antenna was enhanced. These changes are in agreement with the fact that the H-bonding was disrupted. Note that the upconversion is weak, but this proof-of-concept approach will be useful for designing supramolecular triplet photosensitizers and for the application of these compounds. For B-3-Me, no such enhancement of the fluorescence of the antenna was observed in the presence of HFIP (Fig. 11d).
3. Conclusion
In summary, hydrogen bond mediated supramolecular assemblies of Bodipy and C60 modules were prepared and acted as supramolecular triplet photosensitizers. Intra-assembly singlet and triplet state energy transfers were observed. The molecular assemblies were used as supramolecular triplet photosensitizers for triplet–triplet annihilation upconversion. Three different thymine-containing visible light-harvesting Bodipy derivatives (B-1, B-2 and B-3, which show absorption at 505 nm, 630 nm and 593 nm, respectively) were used as the H-bonding modules, whereas 1,6-diaminopyridine appended C60 was used as the complementary hydrogen bonding module (C-1). Visible light-harvesting antennae with methylated thymine were prepared as reference compounds (B-1-Me, B-2-Me and B-3-Me), which are unable to form strong H-bonds with C-1. Triple H-bonds are formed between each Bodipy module (B-1, B-2 and B-3 visible light-harvesting antennae) and the bonding module (C-1). The photophysical properties of the assemblies were studied with steady state UV/vis absorption spectroscopy, fluorescence emission spectroscopy, electrochemical characterization, and nanosecond transient absorption spectroscopy. Singlet energy transfer from the Bodipy antenna to the C60 module was confirmed by the fluorescence quenching studies. The methylated modules were unable to form strong H-bonds with C-1, and fluorescence quenching was observed to a lesser extent. The nanosecond transient absorption spectroscopy showed that the triplet state is either localized on the C60 module (assembly B-1·C-1), or on the Bodipy antenna (for assemblies B-2·C-1 and B-3·C-1). For assembly B-1·C-1, the photophysical process involves intra-assembly singlet energy transfer from the antenna to the C60 module, and then intersystem crossing of the latter produces the triplet excited state. The triplet state is localized on the C60 part in assembly B-1·C-1. For B-2·C-1 and B-3·C-1, intra-assembly ping-pong singlet/triplet energy transfer was proposed, and as a result the triplet state is confined on the styryl-Bodipy part in B-2·C-1 and B-3·C-1. No significant triplet state production was observed for the reference mixtures of B-1-Me/C-1, B-2-Me/C-1 and B-3-Me/C-1, for which no strong H-bonds were formed. These supramolecular assemblies were used as triplet photosensitizers for triplet–triplet annihilation upconversion. These studies may offer a new approach for designing organic triplet photosensitizers.
4. Experimental section
4.1 Materials
UV/vis absorption spectra were measured with an Agilent 8453 spectrophotometer. Fluorescence spectra were recorded on a Shimadzu RF-5301 PC spectrofluorometer. Luminescence lifetimes were measured on an OB920 luminescence lifetime spectrometer (Edinburgh Instruments, UK).
4.2 Syntheses
Synthesis of C-1.
8 (0.043 mmol, 42.7 mg), sodium ascorbate (0.0237 mmol, 4.7 mg) and CuSO4 (0.0118 mmol, 2.9 mg) were added successively to a solution of 3 (0.04 mmol, 10 mg) in a mixed CHCl3–EtOH–water (12
:
1
:
1, v/v, 28 mL) solvent. The mixture was stirred at RT for 12 h. Then water was added, and the mixture was extracted with CH2Cl2. The organic phase was dried over Na2SO4. The solvent was evaporated under reduced pressure. The crude product was purified by column chromatography (silica gel, CH2Cl2–CH3OH, 50
:
1, v/v) to afford a gray solid. Yield: 26.5 mg (50.2%). 1H NMR (500 MHz, DMSO-d6): 10.59 (s, 1H), 10.13 (s, 1H), 8.24 (s, 1H), 7.76–7.65 (m, 3H), 7.49 (d, 2H, J = 8.3 Hz), 7.11 (d, 2H, J = 8.3 Hz), 5.50–5.46 (m, 4H), 5.17 (s, 2H), 4.50–4.44 (m, 2H), 2.12 (s, 3H), 1.29 (t, 3H, J = 7.0 Hz). 13C NMR (100 MHz, DMSO-d6): 162.5, 144.2, 144.1, 144.0, 143.8, 143.7, 143.2, 140.0, 138.4, 137.7, 131.1, 114.5, 71.1, 60.7, 52.1, 30.7, 23.9, 13.9. HRMS: m/z calcd for [C75H14O5]+: 994.0841; found: 994.0844.
Synthesis of B-1.
18 (0.18 mmol, 30 mg), sodium ascorbate (0.0237 mmol, 4.7 mg) and CuSO4 (0.0118 mmol, 2.9 mg) were added to a solution of 11 (0.18 mmol, 75 mg) in CHCl3–EtOH–water (12
:
1
:
1, v/v, 28 mL) and the mixture was stirred at RT for 12 h. In a typical work-up, water was added and the mixture was extracted with CH2Cl2. The organic phase was dried over sodium sulfate. After removal of the solvent, the product was purified by column chromatography (silica gel, CH2Cl2–CH3OH, 25
:
1, v/v) to afford an orange solid. Yield: 33.6 mg (32.6%). 1H NMR (500 MHz, DMSO-d6): 11.3 (s, 1H), 8.18 (s, 1H), 7.64 (s, 1H), 7.26 (d, 2H, J = 8.4 Hz), 7.09 (d, 2H, J = 8.5 Hz), 6.17 (s, 2H), 4.91 (s, 2H), 4.79 (t, 2H, J = 4.7 Hz), 4.46 (d, 2H, J = 4.6 Hz), 2.24 (s, 6H), 1.74 (s, 3H), 1.36 (s, 6H). 13C NMR (100 MHz, CDCl3): 163.8, 155.5, 150.6, 140.2, 128.2, 121.2, 114.9, 111.2, 66.3, 49.9, 42.9, 29.7, 14.6, 12.3. HRMS (MALDI): m/z calcd for [C29H30N7O3F2B]+: 573.2741; found: 573.2504.
Synthesis of B-1-Me.
19 (0.15 mmol, 26 mg), sodium ascorbate (0.0237 mmol, 4.7 mg) and CuSO4 (0.0118 mmol, 2.9 mg) were added to a solution of 11 (0.15 mmol, 65 mg) in CHCl3–EtOH–water (12
:
1
:
1, v/v, 14 mL) and the mixture was stirred at RT for 12 h. After the typical work-up, the crude product was purified by column chromatography (silica gel, CH2Cl2–CH3OH, 40
:
1, v/v) to afford an orange solid. Yield: 35.6 mg (40.2%). 1H NMR (500 MHz, CDCl3): 8.00 (s, 1H), 7.41 (s, 1H), 7.20 (d, 2H, J = 6.5 Hz), 6.99 (d, 2H, J = 8.1 Hz), 5.98 (s, 2H), 5.03 (s, 2H), 4.82 (s, 2H), 4.43 (s, 2H), 3.33 (s, 3H), 2.54 (s, 6H), 1.93 (s, 3H), 1.39 (s, 6H). 13C NMR (100 MHz, CDCl3): 164.1, 158.8, 155.6, 151.8, 140.8, 131.9, 128.5, 121.4, 115.3, 110.5, 66.2, 50.4, 44.1, 28.1, 13.2. HRMS (MALDI): m/z calcd for [C30H32N7O3F2B]+: 587.2628; found: 587.2614.
Synthesis of B-2.
18 (0.07 mmol, 11.2 mg), sodium ascorbate (0.047 mmol, 9.4 mg) and CuSO4 (0.0236 mmol, 5.8 mg) were added to a solution of 12 (0.07 mmol, 40 mg) in CHCl3–EtOH–water (12
:
1
:
1, v/v, 14 mL) and the mixture was stirred at RT for 12 h. After the work-up, the crude product was purified by column chromatography (silica gel, CH2Cl2–CH3OH, 25
:
1, v/v) to afford a blue solid. Yield: 33 mg (62.9%). 1H NMR (500 MHz, CDCl3): 8.64 (s, 1H), 7.94 (s, 1H), 7.75–7.84 (m, 6H), 7.41–7.26 (m, 11H), 7.00 (s, 2H), 6.64 (s, 2H), 4.96 (s, 2H), 4.80 (s, 2H), 4.41 (s, 2H), 1.88 (s, 3H), 1.46 (s, 6H). 13C NMR (100 MHz, CDCl3): 169.3, 163.5, 157.4, 156.0, 147.8, 145.4, 143.6, 141.2, 138.6, 134.9, 132.4, 124.1, 122.7, 120.3, 115.7, 71.5, 54.9, 48.0, 34.7, 19.7, 17.0. HRMS (MALDI): m/z calcd for [C43H38N7O3F2B]+: 749.3097; found: 749.3092.
Synthesis of B-2-Me.
19 (0.05 mmol, 8.5 mg), sodium ascorbate (0.047 mmol, 9.4 mg) and CuSO4 (0.0236 mmol, 5.8 mg) were added to a solution of 12 (0.05 mmol, 28 mg) in CHCl3–EtOH–water (12
:
1
:
1, v/v, 14 mL) and the mixture was stirred at RT for 12 h. After the work-up, the crude product was purified by column chromatography (silica gel, CH2Cl2–CH3OH, 40
:
1, v/v) to afford a blue solid. Yield: 33 mg (68.1%). 1H NMR (400 MHz, CDCl3): 7.91 (s, 1H), 7.77–7.63 (m, 6H), 7.39–7.68 (m, 13H), 7.64 (s, 2H), 6.64 (s, 2H), 5.00 (s, 2H), 4.77 (s, 2H), 4.40 (s, 2H), 3.34 (s, 3H), 1.93 (s, 3H), 1.46 (s, 6H). 13C NMR (100 MHz, CDCl3): 164.1, 158.5, 152.8, 152.0, 151.7, 148.0, 142.2, 138.7, 137.7, 136.4, 133.8, 130.1, 129.0, 127.7, 125.0, 119.4, 118.0, 115.2, 110.4, 66.4, 50.0, 44.1, 28.1, 15.1, 13.2. HRMS (MALDI): m/z calcd for [C44H40N7O3F2B]+: 763.3254; found: 763.3294.
Synthesis of B-3.
18 (0.05 mmol, 8.2 mg), sodium ascorbate (0.047 mmol, 9.4 mg) and CuSO4 (0.0236 mmol, 5.8 mg) were added to a solution of 16 (0.055 mmol, 35 mg) in CHCl3–EtOH–water (12
:
1
:
1, v/v, 14 mL) and the mixture was stirred at RT for 24 h. After the work-up, the crude product was purified by column chromatography (silica gel, CH2Cl2–CH3OH, 25
:
1, v/v) to afford a blue solid. Yield: 22.3 mg (50.2%). 1H NMR (400 MHz, CDCl3): 8.99 (s, 1H), 8.28 (s, 1H), 8.17 (d, 2H, J = 7.6 Hz), 7.79–7.69 (m, 2H), 7.50–7.38 (m, 6H), 7.24–7.22 (m, 3H), 7.00 (s, 2H), 6.66 (s, 1H), 5.99 (s, 1H), 5.00 (s, 2H), 4.81 (s, 2H), 4.41 (s, 3H), 4.31 (t, 2H, J = 6.7 Hz), 2.62 (s, 3H), 1.88 (s, 3H), 1.44–1.40 (m, 8H), 0.96 (t, 3H, J = 6.7 Hz). 13C NMR (100 MHz, CDCl3): 164.4, 154.1, 151.2, 141.6, 140.8, 138.2, 133.3, 130.0, 123.4, 120.8, 119.5, 117.6, 116.2, 115.1, 111.3, 109.2, 66.1, 43.1, 31.1, 30.1, 20.6, 13.9, 12.3. HRMS (MALDI): m/z calcd for [C46H45N8O3F2B]+: 806.3676; found: 806.3685.
Synthesis of B-3-Me.
19 (0.04 mmol, 7.1 mg), sodium ascorbate (0.047 mmol, 9.4 mg), CuSO4 (0.0236 mmol, 5.8 mg) were added to a solution of 16 (0.04 mmol, 25.6 mg) in a mixture of CHCl3–EtOH–water (14 mL, 12
:
1
:
1, v/v) and the mixture was stirred at RT for 24 h. After the typical work-up, the crude product was purified by column chromatography (silica gel, CH2Cl2–CH3OH, 40
:
1, v/v) to afford a blue solid. Yield: 19.6 mg (59.7%). 1H NMR (400 MHz, CDCl3): 8.28 (s, 1H), 8.17 (d, 2H, J = 9.5 Hz), 7.78–7.69 (m, 2H), 7.50–7.38 (m, 5H), 7.25–7.22 (m, 4H), 7.00 (d, 2H, J = 7.4 Hz), 6.67 (s, 1H), 5.99 (s, 1H), 5.02 (s, 2H), 4.80 (s, 2H), 4.42 (s, 2H), 4.31 (t, 2H, J = 7.5 Hz), 3.34 (s, 3H), 2.62 (s, 3H), 1.93 (s, 3H), 1.47–1.38 (m, 8H), 0.96 (t, 3H, J = 6.4 Hz). 13C NMR (100 MHz, CDCl3): 164.1, 158.1, 154.3, 152.0, 143.0, 141.1, 138.3, 133.4, 128.8, 125.8, 123.5, 119.5, 117.6, 114.5, 110.5, 109.5, 66.2, 43.2, 29.5, 28.1, 20.92, 14.1, 12.9. HRMS (MALDI): m/z calcd for [C47H47N8O3F2B]+: 820.3832; found: 820.3850.
4.3 Cyclic voltammetry
Cyclic voltammetry was performed using a CHI610D electrochemical workstation (Shanghai, China). Cyclic voltammograms were recorded at scan rates of 0.05 V s−1. The electrolytic cell used was a three electrode cell. Electrochemical measurements were performed at RT using 0.1 M tetrabutylammonium hexafluorophosphate (TBAP) as supporting electrolyte, after purging with N2. The working electrode was a glassy carbon electrode, and the counter electrode was a platinum electrode. A non-aqueous Ag/AgNO3 (0.1 M in acetonitrile) reference electrode was contained in a separate compartment connected to the solution via a semipermeable membrane. DCM was used as the solvent. Ferrocene was added as the internal reference.
4.4 Nanosecond transient absorption spectra
The spectra were measured on an LP920 laser flash photolysis spectrometer (Edinburgh Instruments, UK). The lifetimes (obtained by monitoring the decay traces) were calculated with the LP900 software. All the solutions were purged with N2 for 15 min before measurement and the gas flow was maintained during the measurements. The samples were excited with a nanosecond pulsed laser (Opolette™ 355II + UV nanosecond pulsed laser, typical pulse length: 7 ns. Pulse repetition: 20 Hz. Peak OPO energy: 4 mJ. The wavelength is tunable in the range of 410–2200 nm. OPOTEK, USA) and the transient signals were recorded on a Tektronix TDS 3012B oscilloscope.
The quantum yield of the triplet formation (ΦT) was determined using the sensitizing method, which was described in detail previously.16b,c,54 The method is based on the use of a triplet energy acceptor (β-carotene) and a standard compound (Ru(bpy)3Cl2, bpy = 2,2′-bipyridine, ΦT = 1). Using optically matched solutions of the reference and the C-1·B-1 hydrogen bonding assembly upon excitation at the same wavelength, the ΔO.D. value, the triplet–triplet energy transfer to β-carotene (KTTET, monitored for the T1 → Tn absorption at 515 nm), and the decay of the triplet energy donor in the absence of β-carotene (k0) were used for calculation of the ΦT values.
4.5 Computational simulations of the B-1 and C-1 complex
Force field parameters of B-1 and C-1 structures.
The structures of the B-1 and C-1 motifs without fullerene were first energy minimized using density functional theory at the B3LYP/6-31G* level. Frequency analyses were performed on the optimized structures in order to obtain the Hessian matrix, from which the corresponding force constants for all the bonds were calculated, as well as the equilibrium bond lengths. The electrostatic potential was calculated based on the same theory, using Mulliken population analysis. The restrained electrostatic potential (RESP) method was used to fit the electrostatic potential in order to obtain the partial atomic charges.55 All quantum chemical calculations were carried out with the Gaussian 09 software package.56 The PARATOOL plug-in of VMD was used to derive the corresponding force constants.57 For the fullerene motif of C-1, the partial charges of all carbon atoms were set to zero, as reported for previous molecular dynamics simulations of fullerene derivatives.58,59 Force constants obtained from the CHARMM force field parameters60 were applied for the bonding and non-bonding interactions within the fullerene motif of C-1.
Molecular dynamics simulations.
The starting complex of B-1 and C-1 is extended, with three pairs of hydrogen bonds between B-1 and C-1. This initial structure was solvated in a TIP3P water box (85 Å × 55 Å × 48 Å), resulting in a system containing 6970 water molecules. The whole system was minimized first, and the B-1·C-1 complex was fixed for 5000 steps to remove unfavorable van der Waals contacts between solvent and solute molecules. Then the system was gradually heated to 300 K, while the B-1·C-1 complex was constrained with harmonic restraints. After a 50 ps equilibration at the desired temperature, the whole system was simulated for another 30 ns without restraints under the NPT ensemble (constant number of atoms, pressure and temperature). The temperature was maintained at 310 K using Langevin dynamics, and the pressure was kept at 1 atm using the Langevin piston method. An integration time step of 2 fs was used. The long-range electrostatic interactions were treated using the PME method. The trajectory was saved every 500 steps (1 ps). A hydrogen bond is formed if the donor atoms (H atoms) are within 3 Å of the acceptor atoms (N or O atoms). All molecular dynamics simulations were performed using the NAMD 2.9 software.61 The non-bonded interaction parameters were taken from the CHARMM 27 force field parameters.60
Acknowledgements
We thank the NSFC (21273028, 21473020 and 21421005), the Royal Society (U.K.) and NSFC (China-UK Cost-Share Science Networks, 21011130154), the Program for Changjiang Scholars and Innovative Research Team in University [IRT_13R06], the State Key Laboratory of Fine Chemicals (KF1203), the Fundamental Research Funds for the Central Universities (DUT14ZD226) and Dalian University of Technology (DUT2013TB07) for financial support.
Notes and references
- J. Xuan and W. Xiao, Angew. Chem., Int. Ed., 2012, 51, 6828–6838 CrossRef CAS PubMed.
- D. P. Hari and B. König, Angew. Chem., Int. Ed., 2013, 52, 4734–4743 CrossRef CAS PubMed.
- J. M. R. Narayanama and C. R. J. Stephenson, Chem. Soc. Rev., 2011, 40, 102–113 RSC.
- L. Shi and W. Xia, Chem. Soc. Rev., 2012, 41, 7687–7697 RSC.
- D. Ravelli, M. Fagnoni and A. Albini, Chem. Soc. Rev., 2013, 42, 97–113 RSC.
- Y. Cakmak, S. Kolemen, S. Duman, Y. Dede, Y. Dolen, B. Kilic, Z. Kostereli, L. T. Yildirim, A. L. Dogan, D. Guc and E. U. Akkaya, Angew. Chem., Int. Ed., 2011, 50, 11937–11941 CrossRef CAS PubMed.
-
(a) A. Kamkaew, S. H. Lim, H. B. Lee, L. V. Kiew, L. Y. Chung and K. Burgess, Chem. Soc. Rev., 2013, 42, 77–88 RSC;
(b) O. J. Stacey and S. J. A. Pope, RSC Adv., 2013, 3, 25550–25564 RSC;
(c) F. Schmitt, J. Freudenreich, N. P. E. Barry, L. Juillerat-Jeanneret, G. Süss-Fink and B. Therrien, J. Am. Chem. Soc., 2012, 134, 754–757 CrossRef CAS PubMed;
(d) A. Gorman, J. Killoran, C. O'Shea, T. Kenna, W. Gallagher and D. O'Shea, J. Am. Chem. Soc., 2004, 126, 10619–10631 CrossRef CAS PubMed.
- A. M. Bugaj, Photochem. Photobiol. Sci., 2011, 10, 1097–1109 CAS.
- Y. C. Simon and C. Weder, J. Mater. Chem., 2012, 22, 20817–20830 RSC.
- T. N. Singh-Rachford and F. N. Castellano, Coord. Chem. Rev., 2010, 254, 2560–2573 CrossRef CAS PubMed.
- J. Zhao, S. Ji and H. Guo, RSC Adv., 2011, 1, 937–950 RSC.
- P. Ceroni, Chem. – Eur. J., 2011, 17, 9560–9564 CrossRef CAS PubMed.
-
(a) M. T. Whited, P. I. Djurovich, S. T. Roberts, A. C. Durrell, C. W. Schlenker, S. E. Bradforth and M. E. Thompson, J. Am. Chem. Soc., 2011, 133, 88–96 CrossRef CAS PubMed;
(b) D. N. Kozhevnikov, V. N. Kozhevnikov, M. Z. Shafikov, A. M. Prokhorov, D. W. Bruce and J. A. Gareth Williams, Inorg. Chem., 2011, 50, 3804–3815 CrossRef CAS PubMed;
(c) S. M. Borisov, R. Saf, R. Fischer and I. Klimant, Inorg. Chem., 2013, 52, 1206–1216 CrossRef CAS PubMed;
(d) Q. Zhao, C. Huang and F. Li, Chem. Soc. Rev., 2011, 40, 2508–2524 RSC.
- J. Zhao, W. Wu, J. Sun and S. Guo, Chem. Soc. Rev., 2013, 42, 5323–5351 RSC.
- J. Zhao, S. Ji, W. Wu, W. Wu, H. Guo, J. Sun, H. Sun, Y. Liu, Q. Li and L. Huang, RSC Adv., 2012, 2, 1712–1728 RSC.
-
(a) S. G. Awuahab and Y. You, RSC Adv., 2012, 2, 11169–11183 RSC;
(b) N. Adarsh, R. Avirah and D. Ramaiah, Org. Lett., 2010, 12, 5720–5723 CrossRef CAS PubMed;
(c) N. Adarsh, M. Shanmugasundaram, R. R. Avirah and D. Ramaiah, Chem. – Eur. J., 2012, 18, 12655–12662 CrossRef CAS PubMed.
- C. Zhang, J. Zhao, S. Wu, Z. Wang, W. Wu, J. Ma, S. Guo and L. Huang, J. Am. Chem. Soc., 2013, 135, 10566–10578 CrossRef CAS PubMed.
- S. Guo, L. Ma, J. Zhao, B. Küçüköz, A. Karatay, M. Hayvali, H. G. Yaglioglub and A. Elmalib, Chem. Sci., 2014, 5, 489–500 RSC.
- J. Ma, X. Yuan, B. Küçüköz, S. Li, C. Zhang, P. Majumdar, A. Karatay, X. H. Li, H. G. Yaglioglu, A. Elmali, J. Zhao and M. Hayvali, J. Mater. Chem. C, 2014, 2, 3900–3913 RSC.
- W. Shi, R. Menting, E. A. Ermilov, P.-C. Lo, B. Röderb and D. P. Ng, Chem. Commun., 2013, 49, 5277–5279 RSC.
-
(a) T. Lazarides, G. Charalambidis, A. Vuillamy, M. Réglier, E. Klontzas, G. Froudakis, S. Kuhri, D. M. Guldi and A. G. Coutsolelos, Inorg. Chem., 2011, 50, 8926–8936 CrossRef CAS PubMed;
(b) F. Hu, J. Huang, M. Cao, Z. Chen, Y. Yang, S. Liu and J. Yin, Org. Biomol. Chem., 2014, 12, 7712–7720 RSC;
(c) K. Wang, C. Wang, Y. Zhang, S. Zhang, B. Yang and Y. Yang, Chem. Commun., 2014, 50, 9458–9461 RSC;
(d) X. Hu, Z. Chen, L. Chen, L. Zhang, J. Hou and Z. Li, Chem. Commun., 2012, 48, 10999–11001 RSC;
(e) X. Xiao, N. Sun, D. Qi and J. Jiang, Polym. Chem., 2014, 5, 5211–5217 RSC;
(f) J. P. Byrne, J. A. Kitchen and T. Gunnlaugsson, Chem. Soc. Rev., 2014, 43, 5302–5325 RSC.
-
(a) K. Feng, M. Yu, S. Wang, G. Wang, C. Tung and L. Wu, ChemPhysChem, 2013, 14, 198–203 CrossRef CAS PubMed;
(b) J. Yao, H. Li, Y. Xu, Q. Wang and D. Qu, Chem. – Asian J., 2014, 9, 3482–3490 CrossRef CAS PubMed.
- Á. J. Jiménez, R. M. K. Calderón, M. S. Rodríguez-Morgade, D. M. Guldi and T. Torres, Chem. Sci., 2013, 4, 1064–1074 RSC.
- S. Gadde, D.-M. S. Islam, C. A. Wijesinghe, N. K. Subbaiyan, M. E. Zandler, Y. Araki, O. Ito and F. D'Souza, J. Phys. Chem. C, 2007, 111, 12500–12503 CAS.
- M. Segura, L. Sánchez, J. Mendoza, N. Martín and D. M. Guldi, J. Am. Chem. Soc., 2003, 125, 15093–15100 CrossRef CAS PubMed.
- L. Sánchez, N. Martín and D. M. Guldi, Angew. Chem., Int. Ed., 2005, 44, 5374–5382 CrossRef PubMed.
- V. Nandwana, L. A. Serrano, K. M. Solntsev, B. Ebenhoch, Q. Liu, G. Y. Tonga, I. D. W. Samuel, G. Cooke and V. M. Rotello, Langmuir, 2013, 29, 7534–7537 CrossRef CAS PubMed.
- H. Fang, S. Wang, S. Xiao, J. Yang, Y. Li, Z. Shi, H. Li, H. Liu, S. Xiao and D. Zhu, Chem. Mater., 2003, 15, 1593–1597 CrossRef CAS.
- Y. Liu, S. Xiao, H. Li, Y. Li, H. Liu, F. Lu, J. Zhuang and D. Zhu, J. Phys. Chem. B, 2004, 108, 6256–6260 CrossRef CAS PubMed.
- J. W. Arbogast, A. P. Darmanyan, C. S. Foote, F. N. Diederich, R. L. Whetten, Y. Rubin, M. M. Alvarez and S. J. Anz, J. Phys. Chem., 1991, 95, 11–12 CrossRef CAS.
- C. Villegas, J. L. Delgado, P.-A. Bouit, B. Grimm, W. Seitz, N. Martín and D. M. Guldi, Chem. Sci., 2011, 2, 1677–1681 RSC.
- A. N. Amin, M. E. El-Khouly, N. K. Subbaiyan, M. E. Zandler, S. C. Fukuzumi and F. D'Souza, Chem. Commun., 2012, 48, 206–208 RSC.
- Y. Liu and J. Zhao, Chem. Commun., 2012, 48, 3751–3753 RSC.
- R. Ziessel, B. D. Allen, D. B. Rewinska and A. Harriman, Chem. – Eur. J., 2009, 15, 7382–7393 CrossRef CAS PubMed.
- W. Wu, J. Zhao, J. Sun and S. Guo, J. Org. Chem., 2012, 77, 5305–5312 CrossRef CAS PubMed.
- L. Huang, X. Yu, W. Wu and J. Zhao, Org. Lett., 2012, 14, 2594–2597 CrossRef CAS PubMed.
- L. Huang, X. Cui, B. Therrien and J. Zhao, Chem. – Eur. J., 2013, 19, 17472–17482 CrossRef CAS PubMed.
- G. Ulrich, R. Ziessel and A. Harriman, Angew. Chem., Int. Ed., 2008, 47, 1184–1201 CrossRef CAS PubMed.
-
(a) H. Lu, J. Mack, Y. Yang and Z. Shen, Chem. Soc. Rev., 2014, 43, 4778–4823 RSC;
(b) X. Yu, X. Jia, X. Yang, W. Liu and W. Qin, RSC Adv., 2014, 4, 23571–23579 RSC;
(c) Y. Chen, H. Wang, L. Wan, Y. Bian and J. Jiang, J. Org. Chem., 2011, 76, 3774–3781 CrossRef CAS PubMed.
- A. Loudet and K. Burgess, Chem. Rev., 2007, 107, 4891–4932 CrossRef CAS PubMed.
- S. Erbas-Cakmak, O. A. Bozdemir, Y. Cakmak and E. U. Akkaya, Chem. Sci., 2013, 4, 858–862 RSC.
-
(a) O. A. Bozdemir, S. Erbas-Cakmak, O. O. Ekiz, A. Dana and E. U. Akkaya, Angew. Chem., Int. Ed., 2011, 50, 10907–10912 CrossRef PubMed;
(b) C. Wan, A. Burghart, J. Chen, F. Bergström, L. Johansson, M. Wolford, T. GyumKim, M. Topp, R. Hochstrasser and K. Burgess, Chem. – Eur. J., 2003, 9, 4430–4441 CrossRef CAS PubMed.
- D. Zhang, V. Martín, I. García-Moreno, A. Costela, M. E. Pérez-Ojedab and Y. Xiao, Phys. Chem. Chem. Phys., 2011, 13, 13026–13033 RSC.
-
J. R. Lakowicz, Principles of Fluorescence Spectroscopy, Kluwer Academic/Plenum Publishers: New York, 2nd edn, 1999 Search PubMed.
-
B. Valeur, Molecular Fluorescence: Principles and Applications, Wiley-VCH Verlag, GmbH, 2001 Search PubMed.
- A. Ghosh, A. Mandoli, D. K. Kumar, N. S. Yadav, T. Ghosh, B. Jha, J. A. Thomas and A. Das, Dalton Trans., 2009, 9312–9321 RSC.
- Y. Chen, W. Lei, G. Jiang, Q. Zhou, Y. Hou, C. Li, B. Zhang and X. Wang, Dalton Trans., 2013, 42, 5924–5931 RSC.
- M. Urbani1, K. Ohkubo, D. M. S. Islam, S. Fukuzumi and F. Langa1, Chem. – Eur. J., 2012, 18, 7473–7485 CrossRef PubMed.
- J. Liu, M. El-Khouly, S. Fukuzumi and D. K. P. Ng, Chem. – Asian J., 2011, 6, 174–179 CrossRef CAS PubMed.
- M. E. El-Khouly and S. Fukuzumi, J. Porphyrins Phthalocyanines, 2011, 15, 111–117 CrossRef CAS.
- C. C. Hofmann, S. M. Lindner, M. Ruppert, A. Hirsch, S. A. Haque, M. Thelakkat and J. Köhler, J. Phys. Chem. B, 2010, 114, 9148–9156 CrossRef CAS PubMed.
- L. Zhang, B. Chen, L. Wu, C. Tung, H. Cao and Y. Tanimoto, Chem. – Eur. J., 2003, 9, 2763–2769 CrossRef CAS PubMed.
- T. S. Balaban, N. Berova, C. M. Drain, R. Hauschild, X. F. Huang, H. Kalt, S. Lebedkin, J.-M. Lehn, F. Nifaitis, G. Pescitelli, V. I. Prokhorenko, G. Riedel, G. Smeureanu and J. Zeller, Chem. – Eur. J., 2007, 13, 8411–8427 CrossRef CAS PubMed.
- C. V. Kumar, L. Qin and P. K. Das, J. Chem. Soc., Faraday Trans. 2, 1984, 80, 783–793 RSC.
- J. Wang, P. Cieplak and P. A. Kollman, J. Comput. Chem., 2000, 21, 1049–1074 CrossRef CAS.
-
M. J. Frisch, G. W. Trucks, H. B. Schlegel, G. E. Scuseria, M. A. Robb, J. R. Cheeseman, G. Scalmani, V. Barone, B. Mennucci, G. A. Petersson, H. Nakatsuji, M. Caricato, X. Li, H. P. Hratchian, A. F. Izmaylov, J. Bloino, G. Zheng, J. L. Sonnenberg, M. Hada, M. Ehara, K. Toyota, R. Fukuda, J. Hasegawa, M. Ishida, T. Nakajima, Y. Honda, O. Kitao, H. Nakai, T. Vreven, J. A. Montgomery, Jr, J. E. Peralta, F. Ogliaro, M. Bearpark, J. J. Heyd, E. Brothers, K. N. Kudin, V. N. Staroverov, R. Kobayashi, J. Normand, K. Raghavachari, A. Rendell, J. C. Burant, S. S. Iyengar, J. Tomasi, M. Cossi, N. Rega, J. M. Millam, M. Klene, J. E. Knox, J. B. Cross, V. Bakken, C. Adamo, J. Jaramillo, R. Gomperts, R. E. Stratmann, O. Yazyev, A. J. Austin, R. Cammi, C. Pomelli, J. W. Ochterski, R.
L. Martin, K. Morokuma, V. G. Zakrzewski, G. A. Voth, P. Salvador, J. J. Dannenberg, S. Dapprich, A. D. Daniels, O. Farkas, J. B. Foresman, J. V. Ortiz, J. Cioslowski and D. J. Fox, Gaussian 09, Revision A.01, Gaussian, Inc., Wallingford CT, 2009 Search PubMed.
- W. Humphrey, A. Dalke and K. Schulten, J. Mol. Graphics, 1996, 14, 33–38 CrossRef CAS.
- Z. Cao, Y. Peng, S. Li, L. Liu and T. Yan, J. Phys. Chem. C, 2009, 113, 3096–3104 CAS.
- L. Monticelli, J. Chem. Theory Comput., 2012, 8, 1370–1378 CrossRef CAS.
- A. D. MacKerell, Jr, D. Bashford, M. Bellott, R. L. Dunbrack, Jr, J. D. Evanseck, M. J. Field, S. Fischer, J. Gao, H. Guo, S. Ha, D. Joseph-McCarthy, L. Kuchnir, K. Kuczera, F. T. K. Lau, C. Mattos, S. Michnick, T. Ngo, D. T. Nguyen, B. Prodhom, W. E. Reiher, B. Roux, M. Schlenkrich, J. C. Smith, R. Stote, J. Straub, M. Watanabe, J. Wiórkiewicz-Kuczera, D. Yin and M. Karplus, J. Phys. Chem. B, 1998, 102, 3586–3616 CrossRef PubMed.
- J. C. Phillips, R. Braun, W. Wang, J. Gumbart, E. Tajkhorshid, E. Villa1, C. Chipot, R. D. Skeel, L. Kalé and K. Schulten, J. Comput. Chem., 2005, 26, 1781–1802 CrossRef CAS PubMed.
Footnote |
† Electronic supplementary information (ESI) available: Syntheses, structure characterization data, and UV/vis absorption and emission spectra. See DOI: 10.1039/c4sc03865g |
|
This journal is © The Royal Society of Chemistry 2015 |
Click here to see how this site uses Cookies. View our privacy policy here.