DOI:
10.1039/C4SC02600D
(Edge Article)
Chem. Sci., 2015,
6, 301-307
Core–shell structured phosphorescent nanoparticles for detection of exogenous and endogenous hypochlorite in live cells via ratiometric imaging and photoluminescence lifetime imaging microscopy†
Received
26th August 2014
, Accepted 22nd September 2014
First published on 23rd September 2014
Abstract
We report a ratiometric phosphorescence sensory system for hypochlorite (ClO−) based on core–shell structured silica nanoparticles. Two phosphorescent iridium(III) complexes were immobilised in the inner solid core and outer mesoporous layer of the nanoparticles, respectively. The former is insensitive to ClO− and thus serves as an internal standard to increase the accuracy and precision, while the latter exhibits a specific and significant luminogenic response to ClO−, providing high selectivity and sensitivity. Upon exposure to ClO−, the nanoparticles display a sharp luminescence colour change from blue to red. Additionally, intracellular detection of exogenous and endogenous ClO− has been demonstrated via ratiometric imaging and photoluminescence lifetime imaging microscopy. Compared to intensity-based sensing, ratiometric and lifetime-based measurements are independent of the probe concentration and are thus less affected by external influences, especially in intracellular applications.
Introduction
Hypochlorite (ClO−) is an oxidizing agent that has been widely applied for bleaching, disinfection, and water treatment.1 In biology, ClO−, as one of the important reactive oxygen species (ROS), plays an important role in the destruction of pathogens in the immune system.2 It is produced endogenously in leukocytes by myeloperoxidase (MPO) which catalyzes the oxidation of chloride to ClO− by hydrogen peroxide.3 Unregulated ClO− production may be associated with various cardiovascular diseases, neuron degeneration, arthritis and cancer.4 Owing to the important biological roles of ClO−, the design of sensitive and selective probes for its accurate and precise detection has attracted much attention. Optical probes including organic dyes,5 nanoparticles,6 transition metal complexes7 and lanthanide chelates8 are the most frequently used reagents to detect intracellular ClO−. Most of these probes exhibit emission enhancement upon oxidation by ClO− and offer high sensitivity and excellent spatial and temporal resolution in intracellular and in vivo detection via luminescence imaging techniques.5,6,7b,8
In addition to intensity-based probes, wavelength-ratiometric probes have received much interest.5i,9 The presence of analyte can be reflected by a change in the ratio of intensities at two luminescence wavelengths. Ratiometric probes are self-calibrating and have high accuracy and precision, especially in intracellular and in vivo detection. However, the use of ratiometric probes is relatively rare compared to intensity-based sensors because of the difficulty in the design and synthesis of ratiometric dyes. Here, we describe a general approach for the convenient preparation of luminescent ratiometric probes. Core–shell structured silica nanoparticles are selected to construct ratiometric probes because these particles exhibit excellent hydrophilicity, biocompatibility and stability.10 Importantly, the core and shell allow independent modification to meet their respective requirements. As shown in Fig. 1, the solid core is covalently labelled with an emissive reference compound that is insensitive to the analyte and thus can serve as an internal standard to increase the accuracy and precision of the measurement, while mesoporous silica is employed to form the outer shell in which a regular arrangement of mesopores facilitates fast and efficient adsorption of a responsive compound that shows a specific and significant luminogenic response to the analyte, providing high selectivity and sensitivity. Upon interaction with the analyte, these nanoparticles will display changes in their luminescence profile, from dominance of the reference compound to dominance of the responsive one (Fig. 1).
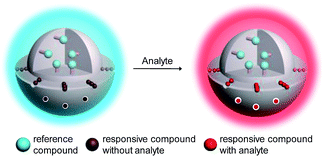 |
| Fig. 1 Design concept of a ratiometric probe based on core–shell structured nanoparticles. | |
Lifetime-based measurements are another powerful method to identify the presence of analyte when a probe exhibits changes in emission lifetime upon interaction with the analyte. The fast development of photoluminescence lifetime imaging microscopy (PLIM) facilitates the lifetime-based detection of intracellular analytes. Similar to the intensity ratio of a ratiometric probe, the lifetime of a probe is independent of the probe concentration or the power of the laser source. Phosphorescent transition metal complexes have been attracting much interest in intracellular sensing owing to their interesting phosphorescence characteristics such as high photostabilities, high phosphorescence quantum yields, large Stokes shifts and tunable phosphorescence colours.11 Additionally, their long and sensitive phosphorescence lifetimes allow lifetime-based measurements in the absence of interference from short-lived scattering and autofluorescence.12
In this work, two phosphorescent iridium(III) complexes 1 and 2 (Scheme 1) have been prepared to serve as ClO− insensitive and sensitive compounds, respectively, to construct the core–shell structured ratiometric sensor SiO2-1@mSiO2-2. Utilisation of SiO2-1@mSiO2-2 to detect intracellular exogenous and endogenous ClO− has been demonstrated via ratiometric imaging and PLIM.
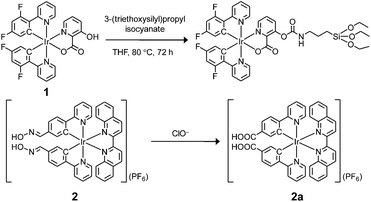 |
| Scheme 1 Preparation of iridium(III) silane analogue from complex 1 and recognition mechanism of complex 2 toward ClO−. | |
Results and discussion
Design and properties of the reference and responsive iridium(III) complexes
Complex 1 was prepared as the reference compound as it is unreactive toward ClO− and the hydroxyl group allows covalent immobilisation onto silica particles (Scheme 1). Complex 2 was designed as the responsive compound because it shows a phosphorogenic response toward ClO− due to elimination of the isomerisation of the aldoxime groups (C
N–OH).5d,7a,c The selection of 2,2’-biquinoline as the diimine ligand was to tune the emission colour of complex 2 into the red region, and thus the emission spectrum was separated from that of the blue-emissive complex 1. Complex 2 was obtained from reaction of the bis(pyridylbenzaldehyde) analogue with hydroxylamine hydrochloride in refluxing methanol/dichloromethane (Scheme S1†). The complexes were characterized by 1H NMR spectroscopy, IR spectroscopy and positive-ion MALDI-TOF-MS (ESI†).
Upon photoexcitation, complex 1 displayed a structured emission band with the maxima at 479 and 500 nm, while complex 2 showed a broad band centred at 609 nm (Fig. 2a). The emissions of complexes 1 and 2 have been assigned to triplet intraligand (3IL) and metal-to-ligand charge-transfer (3MLCT) excited states, respectively. It is noteworthy that complexes 1 and 2 could be simultaneously excited at 405 nm, which is one of the most commonly used laser sources in confocal microscopy, and that their respective blue and red phosphorescences were spectrally well separated (Δλ > 100 nm). Additionally, both complexes retained their phosphorescence intensities when mixed with each other, indicating limited energy or electron transfer between these two complexes.
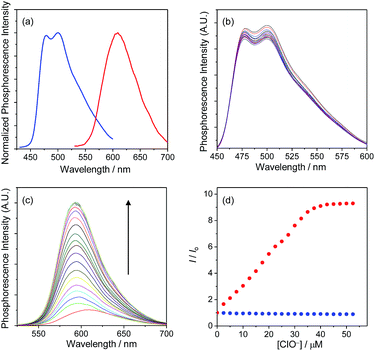 |
| Fig. 2 (a) Normalized phosphorescence spectra of complexes 1 (blue) and 2 (red) in MeOH at 298 K. (b) Phosphorescence spectral traces of complex 1 (1.4 μM) in MeOH at 298 K in the presence of 0–50 μM NaClO. (c) Phosphorescence spectral traces of complex 2 (1.5 μM) in MeOH at 298 K in the presence of 0–50 μM NaClO. (d) Phosphorescence titration curves of complexes 1 (blue) and 2 (red). The excitation wavelength was 405 nm. The emission intensities of complexes 1 and 2 were monitored at 500 and 598 nm, respectively. | |
The phosphorescence responses of complexes 1 and 2 toward ClO− were studied via emission titrations in MeOH due to their limited water solubility. Results showed that addition of ClO− to complex 1 did not cause any notable change in the phosphorescence spectrum or lifetime (about 69 and 72 ns in the absence and presence of ClO−, respectively), whereas complex 2 displayed a phosphorescence enhancement of about 9.2 fold at 598 nm and lifetime elongation from 265 to 410 ns upon addition of ClO− (Fig. 2b–d). The significant phosphorescence change of complex 2 has been ascribed to the formation of the bis-carboxyl analogue 2a upon oxidation by ClO− (Scheme 1), eliminating the isomerisation of the aldoxime groups,5d,7a,c which is an effective nonradiative decay process and quenches the phosphorescence of complex 2. The generation of complex 2a has also been confirmed by the positive-ion MALDI-TOF mass spectrometry, which revealed a peak at m/z = 845, corresponding to the bis-carboxyl complex 2a cation. All these photophysical properties of complexes 1 and 2 and their sensory behaviours fulfilled the requirements of cooperation in a hybridised ratiometric sensory system.
Preparation of core–shell structured nanoparticles
In the preparation of core–shell structured nanoparticles,13 complex 1 first reacted with 3-(triethoxysilyl)propyl isocyanate to give the iridium(III) silane analogue (Scheme 1), which was then used to prepare the solid silica core with tetraethyl orthosilicate (TEOS) as a crosslinking reagent. After that, the core was coated with a mesoporous silica layer in the presence of TEOS and surfactant cetyltrimethylammonium bromide (CTAB). Incubation of these nanoparticles with complex 2 (1 mg ml−1) in ethanol allows efficient adsorption, giving the target ratiometric probe SiO2-1@mSiO2-2. The transmission electron microscopy (TEM) images showed that the nanoparticles were well dispersed in solution and spherical in shape with a core diameter of about 80 nm and a shell thickness of 18 nm (Fig. 3a), which was consistent with the dynamic light scattering (DLS) measurements which revealed an average diameter of 123 nm for SiO2-1@mSiO2-2 dispersed in PBS solution. The small-angle X-ray diffraction (XRD) patterns indicated a typical hexagonally packed mesoporous structure (Fig. 3b). The nitrogen adsorption/desorption isotherm measurements for the SiO2-1@mSiO2-2 nanoparticles (Fig. 3c) revealed a surface area of 710.9 m2 g−1, and a well-defined Barrett–Joyner–Halenda (BJH) pore size of about 3.1 nm was observed (Fig. 3d). The Fourier transform infrared (FTIR) spectrum indicated that complex 1 was covalently immobilised through a carbamate linker (Fig. S1†). The zeta potentials of the nanoparticles changed from −32 to +42 mV upon adsorption of the cationic complex 2. The loading amounts of complexes 1 and 2 were calculated to be about 1.0 and 1.5 μg per 1 mg of nanoparticles, respectively, based on their absorption spectra (Fig. S2†). Additionally, the absorption spectrum of SiO2-1@mSiO2-2 in H2O did not show any remarkable change when the solution was left to stand for more than four weeks, indicative of the high stability of the nanoparticles.
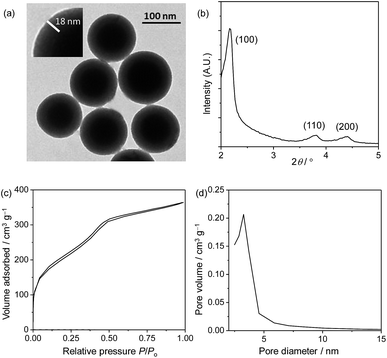 |
| Fig. 3 TEM image (a), XRD pattern (b), nitrogen adsorption/desorption isotherms (c) and pore diameter distribution (d) of SiO2-1@mSiO2-2. | |
Phosphorescence response to ClO−
The phosphorescence response of the core–shell structured nanoparticles SiO2-1@mSiO2-2 to ClO− was investigated in phosphate buffered saline (PBS, pH = 7.4) solution owing to the good solubility of SiO2-1@mSiO2-2 in aqueous solutions. In the absence of ClO−, SiO2-1@mSiO2-2 retained the phosphorescence of both complexes 1 and 2 and displayed intense blue phosphorescence (τ = 83 ns) with a weak emission shoulder in the red region (τ = 171 ns). Additionally, the emission spectrum of SiO2-1@mSiO2-2 is quite similar to that of the mixture containing SiO2-1@mSiO2 and complex 2, suggestive of negligible energy transfer or emission–reabsorption. Compared to the lifetimes of complexes 1 (τ = 69 ns) and 2 (τ = 265 ns) in MeOH, the blue phosphorescence showed a similar lifetime in PBS solution, while the decay of the red phosphorescence was much faster. This is in accordance with the fact that an MLCT emitter displays a shorter lifetime in a more polar solvent, while the lifetime of an IL emitter is less affected by the polarity of solvents.14 It may also be associated with the fact that complex 1 is protected by the inner solid core, while complex 2 is just adsorbed on the outer layer of the nanoparticles. Upon addition of ClO−, the intensity of the red phosphorescence was increased by 5.8 fold with a lifetime elongation to 406 ns, whereas the blue phosphorescence did not show any remarkable difference in either intensity or lifetime (τ = 82 ns) (Fig. 4a). These results are in line with the phosphorescence responses of complexes 1 and 2 toward ClO− (Fig. 2b–d). SiO2-1@mSiO2-2 showed a smaller enhancement factor of the red phosphorescence compared to the response of complex 2 to ClO−, which is due to steric hindrance caused by the silica particles, limiting the full interaction between complex 2 and ClO−. The intensity ratio of the red phosphorescence over the blue one was enhanced from 0.42 to 2.40 when the concentration of ClO− was increased from 0 to 50 μM, and a good linearity was obtained (Fig. 4b). The ratiometric response was specific toward ClO− over other ROS and reactive nitrogen species (RNS) including H2O2, ˙OH, 1O2, NO, NO2−, and NO3− (Fig. 4c). Fig. 4d illustrates the resistance of ratiometric sensing to probe concentration. Addition of ClO− to SiO2-1@mSiO2-2 resulted in a change in the phosphorescence colour from blue to red. The red phosphorescence of SiO2-1@mSiO2-2 in the presence of ClO− was also in sharp contrast to the blue emission of a concentrated SiO2-1@mSiO2-2 solution (Fig. 4d left). However, it was very difficult to distinguish whether the luminogenic probe complex 2 reacted with ClO− or was just at a high concentration (Fig. 4d right), even though it displayed a larger phosphorescence enhancement factor (9.2 fold) compared to SiO2-1@mSiO2-2 (5.8 fold). These results suggested that ratiometric probes are more suitable in intracellular and in vivo applications where the concentrations of the probes are difficult to measure or control.
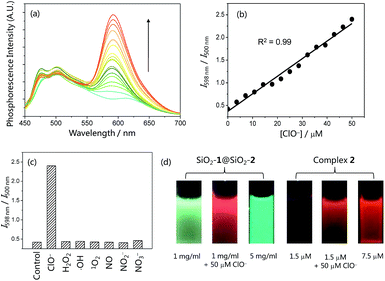 |
| Fig. 4 (a) Phosphorescence spectral traces of SiO2-1@mSiO2-2 (1 mg ml−1) in PBS buffer (pH = 7.4) at 298 K in the presence of 0–50 μM NaClO. (b) Plots of I598 nm/I500 nm as a function of ClO− concentration. (c) Selective ratiometric response of SiO2-1@mSiO2-2 (1 mg ml−1) toward ClO− (50 μM) over other ROS and RNS (200 μM) in PBS buffer at 298 K. (d) Photographs of SiO2-1@mSiO2-2 in PBS solution and complex 2 in MeOH under excitation at 365 nm. | |
Ratiometric imaging of intracellular ClO−
Before investigating the intracellular sensing behaviour of SiO2-1@mSiO2-2 toward ClO−, the cytotoxicity of the nanoparticles toward RAW 264.7 cells was evaluated by the 3-(4,5-dimethyl-2-thiazolyl)-2,5-diphenyltetrazolium bromide (MTT) assay.15 At doses in the range 10–300 μg ml−1, cell viability was greater than 95% after 12 h of incubation (Fig. 5), indicating the good biocompatibility of SiO2-1@mSiO2-2. The use of SiO2-1@mSiO2-2 to visualise intracellular ClO− was demonstrated via confocal laser-scanning microscopy. The excitation wavelength was 405 nm and phosphorescence signals in the blue (480 ± 20 nm) and red (600 ± 20 nm) windows were collected. As shown in Fig. 6a, RAW 264.7 cells treated with SiO2-1@mSiO2-2 (120 μg ml−1) for 3 h displayed intense blue phosphorescence and the red phosphorescence was hardly observed. Further incubation of the cells with NaClO (50 μM) for 1 h selectively and significantly enhanced the red phosphorescence. The overlaid images of the blue and red windows clearly showed that the dominance of the intracellular phosphorescence was shifted from the blue to the red window upon incubation with ClO− (Fig. 6a). The intensity ratio of the red window over the blue window, Ired/Iblue, was determined to increase from 0.16 to 1.6 upon ClO− oxidation of complex 2 to yield complex 2a, which is consistent with the results of the phosphorescence titration (Fig. 4a). Smaller Ired/Iblue values were observed in both the absence and presence of ClO− compared to the results of the extracellular titration, probably due to nonspecific quenching of complexes 2/2a that were localised on the outer layer of the nanoparticles in an intracellular environment. To demonstrate the resistance of the ratiometric detection to external influences, we repeated the intracellular sensing experiments with the following experimental changes: (1) the dose of SiO2-1@mSiO2-2 was increased to 180 μg ml−1 or decreased to 60 μg ml−1; (2) the incubation duration of SiO2-1@mSiO2-2 was increased to 6 h; (3) the incubation temperature of SiO2-1@mSiO2-2 was reduced to 25 °C; (4) the 405 nm laser power was randomly varied. In experiments (1)–(3) the intracellular amount of SiO2-1@mSiO2-2 was influenced, and in experiment (4) the amount of excited SiO2-1@mSiO2-2 was affected randomly. The cell images are shown in Fig. S3.† The luminescence intensity recorded from the red window showed that the intensity-based detection of ClO− was disturbed by the external influences (Fig. 6b red). In contrast, with the signals from the blue window as an internal standard (Fig. 6b blue), the Ired/Iblue values were almost unaffected (Fig. 6b green), demonstrating the strong resistance of the ratiometric detection toward external influences. Therefore, ratiometric sensors are more useful compared to luminescence turn-on or turn-off probes when used in a complex environment such as in intracellular and in vivo applications.
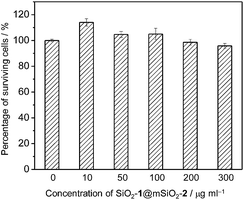 |
| Fig. 5 Percentage of surviving RAW 264.7 cells after exposure to SiO2-1@mSiO2-2 at 37 °C for 12 h. | |
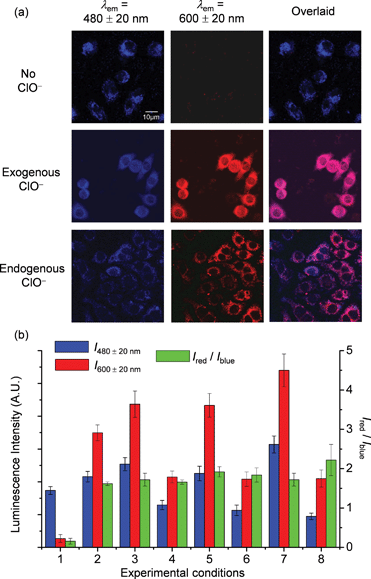 |
| Fig. 6 (a) Luminescence images of RAW 264.7 cells treated with SiO2-1@mSiO2-2 (120 μg ml−1) for 3 h at 37 °C (top), followed by incubation with NaClO (50 μM) for 1 h (middle), and RAW 264.7 cells stimulated with LPS and PMA, and incubated with SiO2-1@mSiO2-2 (120 μg ml−1) for 2 h at 37 °C (bottom). (b) Luminescence intensity of RAW 264.7 cells recorded from the blue window (blue) and the red window (red) and the intensity ratio Ired/Iblue (green). Experimental conditions: RAW 264.7 cells were treated with SiO2-1@mSiO2-2 (120 μg ml−1) for 3 h at 37 °C without (1) and with (2) further incubation with NaClO (50 μM) for 1 h; the concentration of SiO2-1@mSiO2-2 was increased to 180 μg ml−1 (3) and decreased to 60 μg ml−1 (4); the incubation duration of SiO2-1@mSiO2-2 was increased to 6 h (5); the incubation temperature of SiO2-1@mSiO2-2 was reduced to 25 °C (6); the 405 nm laser power was randomly increased (7); and detection of endogenous ClO− was recorded (8). | |
As RAW 264.7 cells are known to produce endogenous ClO− when stimulated with lipopolysaccharide (LPS) and phorbol myristate acetate (PMA),5e,7b,8,16 the feasibility of utilizing SiO2-1@mSiO2-2 to detect endogenous ClO− was tested. The stimulated RAW 264.7 cells showed intense red luminescence after incubation with SiO2-1@mSiO2-2 for 2 h, with an Ired/Iblue value of 2.2 ± 0.4 (Fig. 6). This ratio was larger than those obtained in the detection of exogenous ClO−. This might be because SiO2-1@mSiO2-2 was more ready to react with endogenous ClO−, since internalisation of ClO− into cells was not required. Interestingly, the overlaid images showed that the red and blue phosphorescence signals were well colocalised in the measurement of exogenous ClO−. However, they only partially overlapped in the detection of endogenous ClO−, indicating that the distribution of exogenous ClO− was not even inside the cells. As SiO2-1@mSiO2-2 reacted with ClO− irreversibly, it is important to point out that the measured Ired/Iblue value cannot be used to determine the average concentration of intracellular ClO−. However, the data can serve as an approximate indicator for intracellular ClO− generation.
Photoluminescence lifetime imaging of intracellular ClO−
The significant ClO−-induced lifetime elongation of the red phosphorescence of SiO2-1@mSiO2-2 encouraged us to investigate the intracellular sensing behaviour of the nanoparticles via PLIM. RAW 264.7 cells treated with SiO2-1@mSiO2-2 (120 μg ml−1) for 2 h at 37 °C showed an average luminescence lifetime of about 31 ns (Fig. 7). Further incubation with NaClO (50 μM) for 1 h elongated this value to about 91 ns due to the oxidation of complex 2 to complex 2a. When the cells were stimulated with LPS and PMA, SiO2-1@mSiO2-2 displayed a longer lifetime of about 116 ns without NaClO treatment to the cells, which was consistent with the results of the ratiometric detections, that endogenous ClO− led to a higher degree of conversion of complex 2 to complex 2a compared to exogenous ClO−. All these results indicated that SiO2-1@mSiO2-2 can be used to detect both exogenous and endogenous ClO−via PLIM with high sensitivity.
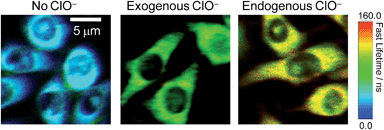 |
| Fig. 7 PLIM images of RAW 264.7 cells treated with SiO2-1@mSiO2-2 (120 μg ml−1) for 2 h at 37 °C (left), followed by treatment with NaClO (50 μM) for 1 h (middle), and RAW 264.7 cells stimulated with LPS and PMA and incubated with SiO2-1@mSiO2-2 (120 μg ml−1) for 2 h at 37 °C (right). | |
Conclusions
In summary, we have described a new design strategy to construct a ratiometric sensing system based on core–shell structured silica nanoparticles, and prepared a phosphorescent ratiometric probe for ClO− by incorporation of two iridium(III) complexes into the nanoparticles. The utilisation of this probe to detect intracellular exogenous and endogenous ClO− has been demonstrated via ratiometric imaging and PLIM. Compared to intensity-based sensing and imaging, both ratiometric and lifetime-based detections are less affected by probe concentration and laser source power. Additionally, in PLIM, the phosphorescence signals are distinguishable from short-lived background interference. Importantly, the design strategy for ratiometric and lifetime-based sensors in this work is not limited to hypochlorite sensing; the incorporation of responsive compounds as reporters and insensitive compounds as internal standards into the core–shell structured nanoparticles will lead to new ratiometric sensors for specific analytes. The utilisation of long-lived luminescent compounds will enable lifetime-based measurements in intracellular applications.
Acknowledgements
The financial support from the National Basic Research Program of China (2012CB933301), National Natural Science Foundation of China (21171098), Program for New Century Excellent Talents in University (NCET-12-0740), the Ministry of Education of China (IRT1148 and 20133223110006), Natural Science Foundation of Jiangsu Province of China (BK20130038) and Priority Academic Program Development of Jiangsu Higher Education Institutions is acknowledged.
Notes and references
- M. Deborde and U. von Gunten, Water Res., 2008, 42, 13–51 CrossRef CAS PubMed.
-
(a) T. Ozben, J. Pharm. Sci., 2007, 96, 2181–2196 CrossRef CAS PubMed;
(b) C. C. Winterbourn, Nat. Chem. Biol., 2008, 4, 278–286 CrossRef CAS PubMed;
(c) Z. M. Prokopowicz, F. Arce, R. Biedroń, C. L. Chiang, M. Ciszek, D. R. Katz, M. Nowakowska, S. Zapotoczny, J. Marcinkiewicz and B. M. Chain, J. Immunol., 2010, 184, 824–835 CrossRef CAS PubMed.
-
(a) J. E. Harrison and J. Shultz, J. Biol. Chem., 1976, 251, 1371–1374 CAS;
(b) D. I. Pattison and M. J. Davies, Biochemistry, 2006, 45, 8152–8162 CrossRef CAS PubMed;
(c) S. E. Gomez-Mejiba, Z. Zhai, M. S. Gimenez, M. T. Ashby, J. Chilakapati, K. Kitchin, R. P. Mason and D. C. Ramirez, J. Biol. Chem., 2010, 285, 20062–20071 CrossRef CAS PubMed.
- R. A. Roberts, D. L. Laskin, C. V. Smith, F. M. Robertson, E. M. Allen, J. A. Doorn and W. Slikker, Toxicol. Sci., 2009, 112, 4–16 CrossRef CAS PubMed.
-
(a) S. Kenmoku, Y. Urano, H. Kojima and T. Nagano, J. Am. Chem. Soc., 2007, 129, 7313–7318 CrossRef CAS PubMed;
(b) Y.-K. Yang, H. J. Cho, J. Lee, I. Shin and J. Tae, Org. Lett., 2009, 11, 859–861 CrossRef CAS PubMed;
(c) T.-I. Kim, S. Park, Y. Choi and Y. Kim, Chem.–Asian J., 2011, 6, 1358–1361 CrossRef CAS PubMed;
(d) X. Cheng, H. Jia, T. Long, J. Feng, J. Qin and Z. Li, Chem. Commun., 2011, 47, 11978–11980 RSC;
(e) Y. Koide, Y. Urano, K. Hanaoka, T. Terai and T. Nagano, J. Am. Chem. Soc., 2011, 133, 5680–5682 CrossRef CAS PubMed;
(f) Y. Zhou, J.-Y. Li, K.-H. Chu, K. Liu, C. Yao and J.-Y. Li, Chem. Commun., 2012, 48, 4677–4679 RSC;
(g) X. Jin, L. Hao, Y. Hu, M. She, Y. Shi, M. Obstb, J. Li and Z. Shi, Sens. Actuators, B, 2013, 186, 56–60 CrossRef CAS PubMed;
(h) Q. Xu, K.-A. Lee, S. Lee, K. M. Lee, W.-J. Lee and J. Yoon, J. Am. Chem. Soc., 2013, 135, 9944–9949 CrossRef CAS PubMed;
(i) X. Wu, Z. Li, L. Yang, J. Han and S. Han, Chem. Sci., 2013, 4, 460–467 RSC;
(j) M. Sun, H. Yu, H. Zhu, F. Ma, S. Zhang, D. Huang and S. Wang, Anal. Chem., 2014, 86, 671–677 CrossRef CAS PubMed.
- P. Panizzi, M. Nahrendorf, M. Wildgruber, P. Waterman, J.-L. Figueiredo, E. Aikawa, J. McCarthy, R. Weissleder and S. A. Hilderbrand, J. Am. Chem. Soc., 2009, 131, 15739–15744 CrossRef CAS PubMed.
-
(a) N. Zhao, Y.-H. Wu, R.-M. Wang, L.-X. Shi and Z.-N. Chen, Analyst, 2011, 136, 2277–2282 RSC;
(b) R. Zhang, Z. Ye, B. Song, Z. Dai, X. An and J. Yuan, Inorg. Chem., 2013, 52, 10325–10331 CrossRef CAS PubMed;
(c) K. Chen, J. W. Bats and M. Schmittel, Inorg. Chem., 2013, 52, 12863–12865 CrossRef CAS PubMed.
- Y. Xiao, R. Zhang, Z. Ye, Z. Dai, H. An and J. Yuan, Anal. Chem., 2012, 84, 10785–10792 CrossRef CAS PubMed.
-
(a) K. K.-W. Lo, K. Y. Zhang, S.-K. Leung and M.-C. Tang, Angew. Chem., Int. Ed., 2008, 47, 2213–2216 CrossRef CAS PubMed;
(b) J. Liu, Y. Liu, Q. Liu, C. Li, L. Sun and F. Li, J. Am. Chem. Soc., 2011, 133, 15276–15279 CrossRef CAS PubMed;
(c) T. Yoshihara, Y. Yamaguchi, M. Hosaka, T. Takeuchi and S. Tobita, Angew. Chem., Int. Ed., 2012, 51, 4148–4151 CrossRef CAS PubMed;
(d) S. Gehrig, M. A. Mall and C. Schultz, Angew. Chem., Int. Ed., 2012, 51, 6258–6261 CrossRef CAS PubMed;
(e) W. Shi, X. Li and H. Ma, Angew. Chem., Int. Ed., 2012, 51, 6432–6435 CrossRef CAS PubMed;
(f) Y. Chen, C. Zhu, Z. Yang, J. Chen, Y. He, Y. Jiao, W. He, L. Qiu, J. Cen and Z. Guo, Angew. Chem., Int. Ed., 2013, 52, 1688–1691 CrossRef CAS PubMed;
(g) X. Wang, J. Sun, W. Zhang, X. Ma, J. Lv and B. Tang, Chem. Sci., 2013, 4, 2551–2556 RSC;
(h) D. Meng, S. Yang, D. Sun, Y. Zeng, J. Sun, Y. Li, S. Yan, Y. Huang, C. W. Bielawski and J. Geng, Chem. Sci., 2014, 5, 3130–3134 RSC.
-
(a) R. G. Chaudhuri and S. Paria, Chem. Rev., 2012, 112, 2373–2433 CrossRef PubMed;
(b) K. E. Sapsford, W. R. Algar, L. Berti, K. B. Gemmill, B. J. Casey, E. Oh, M. H. Stewart and I. L. Medintz, Chem. Rev., 2013, 113, 1904–2074 CrossRef CAS PubMed;
(c) V. Valtchev and L. Tosheva, Chem. Rev., 2013, 113, 6734–6760 CrossRef CAS PubMed;
(d) X. Wu, S. Chang, X. Sun, Z. Guo, Y. Li, J. Tang, Y. Shen, J. Shi, H. Tian and W. Zhu, Chem. Sci., 2013, 4, 1221–1228 RSC;
(e) X. Wu and W. Zhu, Chem. Soc. Rev., 2014 10.1039/c4cs00152d.
-
(a) Q. Zhao, L. Li, F. Li, M. Yu, Z. Liu, T. Yi and C. Huang, Chem. Commun., 2008, 685–687 RSC;
(b) M. Yu, Q. Zhao, L. Shi, F. Li, Z. Zhou, H. Yang, T. Yi and C. Huang, Chem. Commun., 2008, 2115–2117 RSC;
(c) Q. Zhao, F. Li and C. Huang, Chem. Soc. Rev., 2010, 39, 3007–3030 RSC;
(d) C. Li, M. Yu, Y. Sun, Y. Wu, C. Huang and F. Li, J. Am. Chem. Soc., 2011, 133, 11231–11239 CrossRef CAS PubMed;
(e) Q. Zhao, C. Huang and F. Li, Chem. Soc. Rev., 2011, 40, 2508–2524 RSC;
(f) Q. Liu, T. Yang, W. Feng and F. Li, J. Am. Chem. Soc., 2012, 134, 5390–5397 CrossRef CAS PubMed;
(g) K. K.-W. Lo, A. W.-T. Choi and W. H.-T. Law, Dalton Trans., 2012, 41, 6021–6047 RSC;
(h)
K. Y. Zhang and K. K.-W. Lo, in Comprehensive Inorganic Chemistry II, ed. J. Reedijk and K. Poeppelmeier, Elsevier, Oxford, 2013, vol. 8, pp. 657–732 Search PubMed;
(i) Q. Liu, B. Yin, T. Yang, Y. Yang, Z. Shen, P. Yao and F. Li, J. Am. Chem. Soc., 2013, 135, 5029–5037 CrossRef CAS PubMed;
(j)
K. Y. Zhang and K. K.-W. Lo, in Inorganic Chemical Biology: Principles, Techniques and Applications, ed. G. Gasser, John Wiley & Sons, Ltd, Chichester, UK, 2014, pp. 99–147 Search PubMed.
-
(a) Y. You, S. Lee, T. Kim, K. Ohkubo, W.-S. Chae, S. Fukuzumi, G.-J. Jhon, W. Nam and S. J. Lippard, J. Am. Chem. Soc., 2011, 133, 18328–18342 CrossRef CAS PubMed;
(b) E. Baggaley, J. A. Weinstein and J. A. G. Williams, Coord. Chem. Rev., 2012, 256, 1762–1785 CrossRef CAS PubMed;
(c) H. Woo, S. Cho, Y. Han, W.-S. Chae, D.-R. Ahn, Y. You and W. Nam, J. Am. Chem. Soc., 2013, 135, 4771–4787 CrossRef CAS PubMed;
(d) C. Shi, H. Sun, X. Tang, W. Lv, H. Yan, Q. Zhao, J. Wang and W. Huang, Angew. Chem., Int. Ed., 2013, 52, 13434–13438 CrossRef CAS PubMed;
(e) H. Shi, H. Sun, H. Yang, S. Liu, G. Jenkins, W. Feng, F. Li, Q. Zhao, B. Liu and W. Huang, Adv. Funct. Mater., 2013, 23, 3268–3276 CrossRef CAS;
(f) Y. Ma, S. Liu, H. Yang, Y. Wu, H. Sun, J. Wang, Q. Zhao, F. Li and W. Huang, J. Mater. Chem. B, 2013, 1, 319–329 RSC;
(g) W. Xu, X. Zhao, W. Lv, H. Yang, S. Liu, H. Liang, Z. Tu, H. Xu, W. Qiao, Q. Zhao and W. Huang, Adv. Healthcare Mater., 2014, 3, 658–669 CrossRef CAS PubMed.
- S.-H. Wu, C.-Y. Mou and H.-P. Lin, Chem. Soc. Rev., 2013, 42, 3862–3875 RSC.
-
(a) S.-W. Li, Y.-M. Cheng, Y.-S. Yeh, C.-C. Hsu, P.-T. Chou, S.-M. Peng, G.-H. Lee, Y.-L. Tung, P.-C. Wu, Y. Chi, F.-I. Wu and C.-F. Shu, Chem.–Eur. J., 2005, 11, 6347–6357 CrossRef CAS PubMed;
(b) K. K.-W. Lo, K. Y. Zhang, C.-K. Chung and K. Y. Kwok, Chem.–Eur. J., 2007, 13, 7110–7120 CrossRef CAS PubMed.
- T. Mosmann, J. Immunol. Methods, 1983, 65, 55–63 CrossRef CAS.
- R. Wippel, M. Rehn, A. C. F. Gorren, K. Schmidt and B. Mayer, Biochem. Pharmacol., 2004, 67, 1285–1295 CrossRef CAS PubMed.
Footnotes |
† Electronic supplementary information (ESI) available: Experimental procedures, characterisation data, Scheme S1 and Fig. S1–S3. See DOI: 10.1039/c4sc02600d |
‡ K. Y. Zhang and J. Zhang contributed equally to this work. |
|
This journal is © The Royal Society of Chemistry 2015 |
Click here to see how this site uses Cookies. View our privacy policy here.