DOI:
10.1039/C5RA19708B
(Paper)
RSC Adv., 2015,
5, 90466-90472
Bio-complementary supramolecular polymers with effective self-healing functionality†
Received
24th September 2015
, Accepted 15th October 2015
First published on 16th October 2015
Abstract
Complementary nucleobase-functionalized polymers, a combination of base-pair interactions and a blend of two different polymers, can be used for efficient construction of supramolecular polymer networks; these materials exhibit tunable mechanical properties, rapid thermo-responsiveness and self-healing behavior making them highly attractive for a wide range of potential applications. Herein, a multi-uracil functionalized polyhedral oligomeric silsesquioxane (POSS-U) and adenine end-capped three-arm polycaprolactone oligomer (PCL-A) have been successfully developed and show high complementary ability in both solution and the solid state owing to reversible uracil–adenine (U–A)-induced physical cross-linking. The POSS-U/PCL-A blend system demonstrates that physically crosslinked films can be readily tailored to provide the desired mechanical properties and rapidly reprocessed after damage under mild temperature conditions. Importantly, these films can also spontaneously self-heal at room temperature as an autonomously repairing material, without the need for external intervention. Thus, this new system provides a potential route toward the design and fabrication of next-generation self-healing polymers.
Introduction
Self-assembly of supramolecular polymers has recently attracted significant attention due to their ability to form novel structural organizations through highly selective molecular recognition motifs.1–4 As a typical example, non-covalent systems based on complementary nucleobase-pair interactions can regulate the assembly/disassembly process and control hierarchical organization structures;5–7 by doing so, nucleobase-mediated self-assembly allows the rapid formation of distinct polymer-like structures that exhibit unique physical properties, such as a high specificity, directionality, and an interaction strength comparable to multiple hydrogen bonding energies. These features make nucleobase-pairing bonding an indispensable design tool for achieving the required qualities within supramolecular polymers.8–17 For instance, the viscoelastic properties of nucleobase-functionalized materials can be enhanced by introducing complementary hydrogen bonds within a reversible non-covalent matrix to create high molecular weight complexes.11,18,19 However, existing supramolecular polymers still possess several disadvantages, including inefficient thermal stability and the low binding energy of hydrogen-bonded nucleobase pairs, which result in the inherent limitations of these materials and insufficient mechanical properties for certain applications.20–22 Thus, to complement existing methods, it is would be highly beneficial to develop stable, effective synthetic routes for supramolecular polymers that improve their mechanical properties and also achieve rapid reversible binding to promote viscoelastic responses under mild conditions.
To address the challenges described above, supramolecular polymer networks (SPNs) have been demonstrated to form non-covalently crosslinked structures that promote efficient self-assembly of supramolecular polymers, and the mechanical reinforcement and transient hydrogen-bonded networks of SPNs can be directly manipulated to obtain a wide variety of multifunctional materials.23–26 These features also enable supramolecular polymers to undergo immediate responses to stimuli such as heat, light, pH or electricity.27,28 In other words, the introduction of SPNs within synthetic polymers represents a promising approach towards the development of a new generation of stimuli-responsive dynamic systems, which may enable the spontaneous formation of a three-dimensional network to obtain rapid, reversible responses to various environmental conditions and thus enhance the reliability and long-term performance of stimuli-responsive materials. Recently, stimuli-responsive materials have been widely reported to have applications within self-healing systems in different fields, such as surface coatings,29 electrical conductors30–33 and tissue engineering.34,35 Different types of these materials have demonstrated the ability to tune self-healing properties through the introduction of microencapsulated36 or photoactivated repair agents,37,38 reversible covalent39–42 or non-covalent bonding43–50 into the material matrices. However, in terms of sustainability and effectiveness, these approaches still lack the ability to modulate the thermal and mechanical properties of self-healing materials for specific requirements. Stimuli-responsive materials with excellent dynamic and self-healing abilities are urgently required. Based on the above-mentioned desirable properties, SPNs may provide a more suitable approach to promote reversible supramolecular interactions within a network and directly tune the viscoelastic response of the materials to influence their physical properties. Therefore, three-dimensional materials constructed using various SPNs may enable the creation of different types of secondary structure to achieve the required performance within self-healing materials.
Our previous studies demonstrated that the incorporation of complementary nucleobase pairs into synthetic polymers resulted in remarkable dynamic behavior and mechanical properties in conjunction with a substantial increase in thermal stability and a noticeably higher glass transition temperature (Tg).10,14,51–53 Recently, we also reported that combination of a low-molecular weight polyhedral oligomeric silsesquioxane (POSS) macromer with diaminopyridine (DAP) quadruple hydrogen bonding interactions resulted in formation of a non-covalently crosslinked polymer-like membrane with good mechanical properties.54 The DAP complex plays a critical role in the processes of proton exchange, and can be fabricated into proton-conducting devices to provide a high proton-transporting ability.55 Based on these findings, we speculated that introduction of nucleobase-functionalization into a commercial oligomer and inorganic POSS to fabricate uniform supramolecular membranes may considerably affect the mechanical properties of the resulting SPN and enable its use in a range of promising applications such as autonomous self-healing materials. To the best of our knowledge, supramolecular polymers based on complementary interactions between nucleobase pairs with the aim of tuning mechanical properties and achieving self-healing ability have not been previously reported. In this study, a novel POSS macromer containing eleven uracil groups (POSS-U) was successfully synthesized. POSS-U is expected to interact with its complementary adenine end-capped three-arm polycaprolactone oligomer (PCL-A) to form physically cross-linked structures via complementary uracil–adenine (U–A) pairing (Scheme 1).52–55 As the mechanical performance of thin films is largely dependent on the crosslink density of the material matrix, the POSS-U/PCL-A complex could be easily used to prepare the desired thin films using various POSS-U/PCL-A blend ratios to improve the thermal stimuli-responsive properties and reprocessability of these systems. In addition, we also show that fracture-induced mechanochemical activation is rapidly achieved in these materials without the assistance of an external source, which provides excellent self-healing capability to restore the mechanical properties of the fractured materials at room temperature within 24 hours. Thus, this work demonstrates a simple and efficient route for creating polymer-like thin films that act as a new class of supramolecular polymers for developing self-healing materials for a wide range of potential applications.
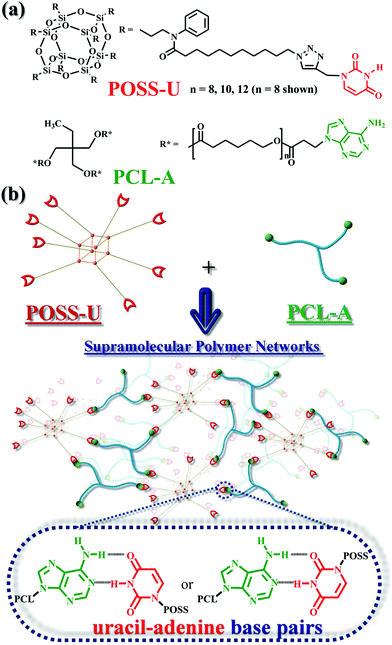 |
| Scheme 1 (a) Chemical structures of nucleobase-functionalized supramolecular materials. (b) Structural representation of the functional supramolecular polymer network and complementary interactions between POSS-U and PCL-A. | |
Experimental section
POSS-U and PCL-A were prepared by a combination of Michael addition and azide–alkyne click reaction. The detailed synthetic procedures, structural characterizations and analytic methods are described in more detail in the ESI.†
Results and discussion
Synthesis of nucleobase-functionalized materials and molecular recognition in complementary POSS-U/PCL-A complexes
Scheme 1 depicts the chemical structures of the complementary nucleobase-functionalized materials. The novel star-like uracil-based POSS derivative, POSS-U, was synthesized via a copper-catalyzed click reaction between azide-functionalized POSS (POSS-N3) with propargyl uracil as a hydrogen bonding segment (Scheme S1†). POSS-U was recovered at high yield (92%) and precisely controlled molecular weights up to a massive 5482 g mol−1 (POSS-U containing ten uracil functional groups forms a major structural component), consistent with the structure of the molecule presented in Fig. S3.† A complementary partner, oligomeric adenine-functionalized three-arm PCL (PCL-A), was obtained by Michael addition of PCL triacrylate reacted with adenine. The resulting PCL-A exhibited a low molecular weight (Mw = 1680 g mol−1) and narrow polydispersity index (PDI = 1.196), as determined by gel permeation chromatography (Fig. S5†). Due to the nature of the molecular recognition between uracil and adenine, POSS-U is able to form complexes with PCL-A via complementary U–A hydrogen bonding pairs, which affords supramolecular organization capabilities to obtain stable physically crosslinked structures via bottom-up assembly of POSS-U/PCL-A complexes. Thus, we investigated the bonding strength and dynamic reversibility of these complexes using variable-temperature proton nuclear magnetic resonance (VT-NMR). The POSS-U/PCL-A mixture was dissolved in tetrachloroethane-d2 at 25 °C to determine the association constant (Ka) of the U–A interaction. The chemical shift of the amide proton of POSS-U gradually shifted downfield from 9.94 ppm to 11.76 ppm with increasing PCL-A content (Fig. S6†) and the Ka was calculated to be 576 M−1 using the Benesi–Hildebrand equation (the detailed calculation is shown in Fig. S7†),56 which is consistent with the previously reported Ka value for the U–A interaction.5,10 When a 50/50 solution of the POSS-U/PCL-A complex was heated from 25 to 100 °C, the characteristic protons of the U–A interactions at H1 and H2 shifted significantly upfield to 9.48 and 6.01 ppm, respectively (Fig. S8†); these changes can be attributed to gradual destruction of complementary hydrogen bonding as temperature increased. However, as soon as the sample was cooled down to 25 °C, these protons spontaneously returned to their initial positions, suggesting that POSS-U and PCL-A form completely thermoreversible hydrogen-bonded complexes in solution.
Polymer-like behavior and self-assembly properties of POSS-U/PCL-A in the bulk state
To investigate the formation of physically crosslinked polymer-like structures in the solid state, the blend film could be easily prepared by casting the tetrachloroethane solution of POSS-U/PCL-A onto a smooth polytetrafluoroethylene (PTFE) substrate, followed by air-drying and thermal annealing at 80 °C. In terms of appearance, POSS-U and PCL-A behaved as solid powders and the POSS-U/PCL-A blend appeared as a black-colored, elastic film (Fig. 1a). This implies that the U–A interaction plays a crucial role as a physical cross-linkage that drastically affects the macroscale behavior of supramolecular materials, prompting us to further explore the mechanical properties of POSS-U/PCL-A films in more detail through universal testing machine measurements.
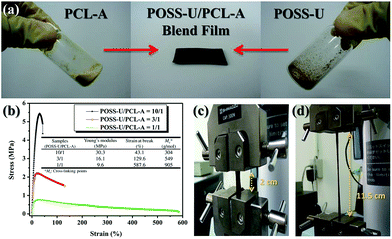 |
| Fig. 1 (a) Photographic images of polymer-like film formed from POSS-U/PCL-A blends. (b) Tensile stress–strain curves for various compositions of POSS-U/PCL-A blends. The insert table in (b) shows a summary of the mechanical properties of all samples. Images of the 1/1 POSS-U/PCL-A film photographed before (c) and after (d) the mechanical test. | |
Cross-linking density is a key parameter that controls the viscoelastic and mechanical properties of thin films. Thus, blending films were prepared by mixing POSS-U and PCL-A at different molar ratios of 10/1, 3/1 and 1/1. Fig. 1b displays the stress–strain diagram for various POSS-U/PCL-A films. As summarized in the inset table in Fig. 1b, the Young's modulus of the 10/1 POSS-U/PCL-A film was determined as 30.3 MPa with an elongation at break of 43.1%, indicating that a high POSS-U content tends to promote plastic behavior. Further increasing the PCL-A content to a 1/1 ratio resulted in a substantial increase in the elongation at break up to 587.6%, whereas the Young's modulus significantly decreased to 9.6 MPa (Fig. 1c and d). This data suggests that elastic behavior dominates plastic behavior in the presence of a large amount of flexible PCL-A segment within the polymer matrix. In other words, these complexes self-assemble into different extents of physically crosslinked structures; manipulation of crosslinking density at various POSS-U/PCL-A blend ratios reveals that the mechanical properties of thin films can be easily tailored. To further understand the physical cross-linking density, we examined the average molecular weight between cross-linking points (Mc) to estimate the extent of complementary hydrogen bonding interactions within the POSS-U/PCL-A films. On the basis of the Young's modulus data and using the Charlesby–Hancock equation (Fig. S9†),57 the Mc of the 1/1 POSS-U/PCL-A film was 905 g mol−1, which was much higher than the values for the 10/1 and 3/1 POSS-U/PCL-A films (Mc = 304 and 549 g mol−1 for the 10/1 and 3/1 complexes, respectively). This implies that increasing the fraction of PCL-A within the blend increases the Mc and results in a higher probability of collisions between the U and A groups due to the progressive equilibrium between the numbers of base pairs (POSS-U contains ten uracil arms and PCL-A has three adenine groups). Thus, the effect of these complementary interactions on the mechanical properties of supramolecular polymers is intriguing, yet has been rarely reported in the past.45,58,59 This led us to further investigate the phase behavior of these complexes in film state via differential scanning calorimetry (DSC) and synchrotron wide-angle X-ray diffraction (WAXD).
Fig. 2a displays the DSC traces for various POSS-U/PCL-A films. PCL-A exhibited a melting point (Tm) of 80 °C and clear glass transition temperature (Tg) of −10 °C, whereas POSS-U had a Tg of only 62 °C. For all formed complexes, incorporation of PCL-A into POSS-U led to an apparent single Tg and disappearance of the Tm, indicating that POSS-U and PCL-A form highly complementary hydrogen-bonded complexes in the bulk state. In addition, the Tgs of the POSS-U/PCL-A films increased gradually from 13 to 44 °C as the content of POSS-U increased. This indicates the presence of different “molecular motions” which is consistent with the observations of mechanical properties (Fig. 1b). To further confirm the DSC results, synchrotron WAXD experiments were carried out at room temperature to analyze the microstructural evolution of these materials. As shown in Fig. 2b, the WAXD profile of PCL-A contained three reflection peaks at 11° (d = 0.69 nm), 17° (d = 0.45 nm) and 24° (d = 0.32 nm). The interlayer distances of 0.32 nm and 0.69 nm suggest that the stacks of dimerized adenine are held together via π–π interactions in these interaction domains,10,20,60 as a result of combining regular structures with a specific arrangement of molecules. The d-spacing distance of 0.45 nm indicates a broad halo corresponding to the intermolecular distance in the amorphous region. The WAXD pattern of pure POSS-U possesses two amorphous halos centered at 4.14° (d = 1.84 nm) and 17° (d = 0.45 nm), respectively (Fig. 2b). The low-angle peak at 4.14° comes from the distance between two neighboring POSS blocks, reflecting the formation of the self-complementary uracil–uracil interactions on the d-spacing of POSS-U film,54,55,61 and another at 0.45 nm that is also attributed to the intramolecular amorphous region. As expected, each of the POSS-U/PCL-A films exhibited a long period peak at 1.76°, corresponding to a d-spacing of 4.33 nm, indicating the occurrence of longer-distance intermolecular connections between nearest-neighbor POSS units. Additionally, the intermolecular π–π interactions of PCL-A corresponding to d-spacings of 0.32 nm and 0.69 nm disappeared, implying that the U–A interactions were occurring. Moreover, these observations also indicate that the POSS-U/PCL-A system exhibits rapid molecular-recognition capabilities, resulting in a stable and tunable SPN within a supramolecular film.
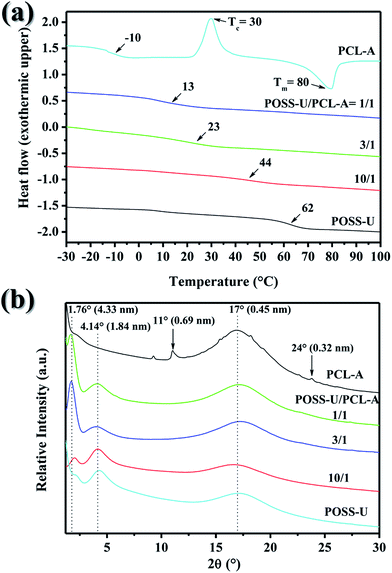 |
| Fig. 2 (a) DSC curves and (b) WAXD data for POSS-U/PCL-A complexes at various molar ratios. | |
Controlling self-healing ability by mild-heat treatment and complementary interactions
To gain further insight into the dynamic behavior of hydrogen bonding-induced phase transitions, we subjected the 3/1 POSS-U/PCL-A film to variable-temperature viscosity measurements. Upon heating from 40 to 120 °C, the viscosity of POSS-U/PCL-A reduced substantially from 1.4 × 104 Pa s at 40 °C to 12.5 Pa s at 120 °C (Fig. 3a). This result suggests that a temperature-dependent association between complementary base pairs occurs as an interesting solid–gel transition from an elastomeric solid to a viscous liquid state when temperature increases, even though POSS-U/PCL-A has a high physical crosslink density at room temperature. These results also agree well with the VT-NMR data (Fig. S8†). After multiple heating and cooling cycles to 120 °C and 40 °C, POSS-U/PCL-A displayed an obvious thermosensitive response and dynamic viscosity returned back to the original high viscosity for each state as temperature decreased; these results demonstrate a strong connection between reconstructive phase transitions and mechanical properties. Thus, we speculated that POSS-U/PCL-A film should be capable of directly remodeling under mild temperature conditions. The chopped 3/1 POSS-U/PCL-A blend sample was placed into a PTFE mold and subsequently heated to 100 °C for 20 min (Fig. S10†), providing a simple and rapid process for producing the desired films. In theory, the remolded supramolecular film should attain the same mechanical performance as the original film. Fig. 3b depicts a typical stress–strain diagram for the original and remolded films. During repeated stress–strain tests, all samples displayed similar mechanical properties with a tensile strength and failure strain of 2.05 ± 0.1 MPa and 114 ± 6%, respectively. These data demonstrate that thermocycled POSS-U/PCL-A film maintains excellent reproduction of mechanical performance and can be rapidly reprocessed under mild temperature conditions (100 °C) using a melt film-forming method. To date, there are no reported examples of complementary multiple hydrogen-bonded systems that exhibit rapid recyclability in this manner.
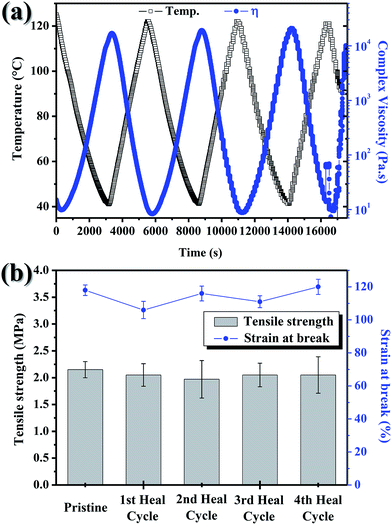 |
| Fig. 3 (a) Temperature cycling viscosity experiments for 3/1 POSS-U/PCL-A: viscosity (solid symbols) and temperature (open symbol) versus time. (b) Stress–strain curves for original and healed 3/1 POSS-U/PCL-A subjected to successive breakage and reprocessing/reshaping procedures. | |
In addition to the thermo-responsive self-healing ability observed for the reversible SPN system, we further investigated the spontaneous self-healing behavior of the fractured POSS-U/PCL-A film under ambient conditions without any external source or stimulus (Scheme S2†). Similarly to previous procedures,43,49 flexible 3/1 POSS-U/PCL-A film was cut into two pieces, and then the pieces were gently connected together for 1 min and subsequently left to heal in a drying oven at 25 °C at a relative humidity of 30% for different healing times. Fig. 4 shows the time-dependent mechanical properties of the self-healing process: after healing for 12 h, approximately 32% of the original breaking strain was recovered. A maximum elongation at break of 121 ± 6% was observed after 24 h, corresponding to a healing efficiency of up to 92% recovery compared to the original film, indicating that almost complete fracture healing was achieved within 24 h. However, further extending the healing time to 48 h did not further increase elongation rate of the healed sample. This is possibly due to restriction of intermolecular hydrogen bonds exposed on the healing surface becoming increasingly likely to locate the nearest interaction partners over time, thus inhibiting continued growth of self-healing efficiency, while longer healing times are required to increase mechanical properties.49 To further verify these results and explore the surface morphology during the autonomous healing of fractures in complementary materials, the relationship between self-healing efficiency and evolution of surface microstructure was observed using a scanning electron microscope (SEM) following the routine sample preparation method described above. As illustrated in Fig. 5, the SEM images indicated that the contact area between the two surfaces of the open cracks was completely fused after healing at room temperature for 24 h. These results further confirm the autonomic self-healing ability of POSS-U/PCL-A blend films, which occurs as a result of the presence of U–A base pairs and their stable interactions, which promote a rapid dynamic response to restore function after damage.
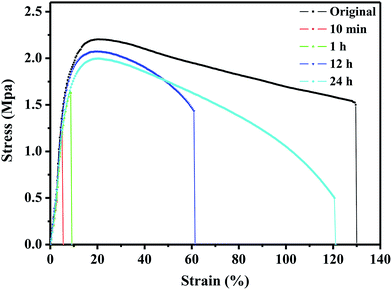 |
| Fig. 4 Stress–strain experiments for both original and healed 3/1 POSS-U/PCL-A films. The blend films were cut into two completely separate pieces and then the pieces were gently contacted together and allowed to heal for different periods of time at 25 °C. | |
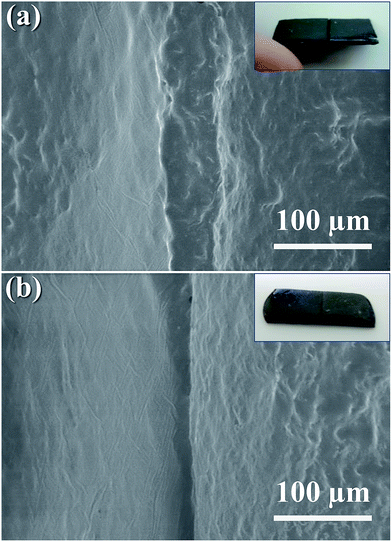 |
| Fig. 5 SEM images showing the changes on the incision interface of 3/1 POSS-U/PCL-A films after (a) 2 h and (b) 24 h healing periods at room temperature; the inserts are photographic images of the mended samples after different healing times. | |
Conclusions
To conclude, this study demonstrates a simple and promising strategy for preparing low-molecular weight supramolecular films that exhibit unusual physical properties including excellent polymer-like features as well as rapid, dynamic and thermo-responsive properties. Incorporation of complementary U–A base-pairs into an oligomer matrix provides a simple self-assembly route to form a stable physically crosslinked polymer network. Due to the ability to tune the U–A content and the presence of a reversible network, these blending films can be readily tailored to obtain the desired mechanical properties. The films can also be reprocessed via a melt film-forming method under mild temperature conditions – as a result of manipulating the reversible hydrogen bonding behavior to efficiently fine-tune molecular recognition. In addition, this newly-developed material can also undergo autonomous repair at ambient temperature in the absence of external stimuli, as evidenced by restoration of the mechanical performance of the damaged materials within the short healing period of 24 h, making this material particularly appealing for the development of self-assembled supramolecular films with autonomic self-repair functionality. Thus, this complementary base-pairing strategy for self-healing materials could be widely applied as a means of promoting wound healing within tissue regenerating materials as hydrogen bonding may provide such materials with the desired functions; these studies are currently are being conducted in our laboratory.
Acknowledgements
This study was supported financially by “Aim for the Top University Plan” of the National Taiwan University of Science and Technology, and the Ministry of Science and Technology, Taiwan (contract no. MOST 104-2221-E-011-153).
Notes and references
- L. Brunsveld, B. J. B. Folmer, E. W. Meijer and R. P. Sijbesma, Chem. Rev., 2001, 101, 4071–4097 CrossRef CAS PubMed.
- J.-M. Lehn, Chem. Soc. Rev., 2007, 36, 151–160 RSC.
- G. Chen and M. Jiang, Chem. Soc. Rev., 2011, 40, 2254–2266 RSC.
- E. Busseron, Y. Ruff, E. Moulina and N. Giuseppone, Nanoscale, 2013, 5, 7098–7140 RSC.
- S. Sivakova and S. J. Rowan, Chem. Soc. Rev., 2005, 34, 9–21 RSC.
- W. H. Binder and R. Zirbs, Adv. Polym. Sci., 2007, 207, 1–78 CrossRef CAS.
- J. L. Sessler, C. M. Lawrence and J. Jayawickramarajah, Chem. Soc. Rev., 2007, 36, 314–325 RSC.
- J. Ziauddin and D. M. Sabatini, Nature, 2001, 411, 107–110 CrossRef CAS PubMed.
- S. Sivakova, J. Wu, C. J. Campo, P. T. Mather and S. J. Rowan, Chem. –Eur. J., 2006, 12, 446–456 CrossRef PubMed.
- C. C. Cheng, C. F. Huang, Y. C. Yen and F. C. Chang, J. Polym. Sci., Part A: Polym. Chem., 2008, 46, 6416–6424 CrossRef CAS PubMed.
- M. Fathalla, C. M. Lawrence, N. Zhang, J. L. Sessler and J. Jayawickramarajah, Chem. Soc. Rev., 2009, 38, 1608–1620 RSC.
- Y. Ura, J. M. Beierle, L. J. Leman, L. E. Orgel and M. R. Ghadiri, Science, 2009, 325, 73–77 CrossRef CAS PubMed.
- P. K. Lo and H. F. Sleiman, J. Am. Chem. Soc., 2009, 131, 4182–4183 CrossRef CAS PubMed.
- I. H. Lin, C. C. Cheng, Y. C. Yen and F. C. Chang, Macromolecules, 2010, 43, 1245–1252 CrossRef CAS.
- S. K. Yang, A. V. Ambade and M. Weck, Chem. Soc. Rev., 2011, 40, 129–137 RSC.
- R. McHale and R. K. O'Reilly, Macromolecules, 2012, 45, 7665–7675 CrossRef CAS.
-
(a) Y. C. Wu and S. W. Kuo, J. Mater. Chem., 2012, 22, 2982–2991 RSC;
(b) J. H. Wang, O. Altukhov, C. C. Cheng, F. C. Chang and S.-W. Kuo, Soft Matter, 2013, 9, 5196–5206 RSC;
(c) A. E. Way, A. B. Korpusik, T. B. Dorsey, L. E. Buerkle, H. A. von Recum and S. J. Rowan, Macromolecules, 2014, 47, 1810–1818 CrossRef CAS.
- J. L. Sessler and J. Jayawickramarajah, Chem. Commun., 2005, 1939–1949 RSC.
- F. Herbst, D. Döhler, P. Michael and W. H. Binder, Macromol. Rapid Commun., 2013, 34, 203–220 CrossRef CAS PubMed.
- S. Sivakova, D. A. Bohnsack, M. E. Mackay, P. Suwanmala and S. J. Rowan, J. Am. Chem. Soc., 2005, 127, 18202–18211 CrossRef CAS PubMed.
- A. J. Wilson, Soft Matter, 2007, 3, 409–425 RSC.
- C. Heinzmann, C. Weder and L. M. de Espinosa, Chem. Soc. Rev., 2015 10.1039/C5CS00477B.
- R. F. M. Lange, M. van Gurp and E. W. Meijer, J. Polym. Sci., Part A: Polym. Chem., 1999, 37, 3657–3670 CrossRef CAS.
- S. Seiffert and J. Sprakelc, Chem. Soc. Rev., 2012, 41, 909–930 RSC.
- T. Aida, E. W. Meijer and S. I. Stupp, Science, 2012, 335, 813–817 CrossRef CAS PubMed.
- P. Wei, X. Yan and F. Huang, Chem. Soc. Rev., 2015, 44, 815–832 RSC.
- M. A. C. Stuart, Nat. Mater., 2010, 9, 101–113 CrossRef PubMed.
-
(a) X. Yan, F. Wang, B. Zheng and F. Huang, Chem. Soc. Rev., 2012, 41, 6042–6065 RSC;
(b) X. Ma and H. Tian, Acc. Chem. Res., 2014, 47, 1971–1981 CrossRef CAS PubMed;
(c) H. Chen, X. Ma, S. Wu and H. Tian, Angew. Chem., Int. Ed., 2014, 53, 14149–14152 CrossRef CAS PubMed;
(d) L. Chen, H. Chen, X. Yao, X. Ma and H. Tian, Chem.–Asian J., 2015 DOI:10.1002/asia.201500704.
- S. H. Cho, S. R. White and P. V. Braun, Adv. Mater., 2009, 21, 645–649 CrossRef CAS PubMed.
- B. J. Blaiszik, S. L. Kramer, M. E. Grady, D. A. McIlroy, J. S. Moore, N. R. Sottos and S. R. White, Adv. Mater., 2012, 24, 398–401 CrossRef CAS PubMed.
- E. Palleau, S. Reece, S. C. Desai, M. E. Smith and M. D. Dickey, Adv. Mater., 2013, 25, 1589–1592 CrossRef CAS PubMed.
- C. Wang, H. Wu, Z. Chen, M. T. McDowell, Y. Cui and Z. Bao, Nat. Chem., 2013, 5, 1042–1048 CrossRef CAS PubMed.
- Z. Zhao and E. M. Arruda, Science, 2014, 344, 591–592 CrossRef CAS PubMed.
- A. Phadke, C. Zhang, B. Arman, C. C. Hsu, R. A. Mashelkar, A. K. Lele, M. J. Tauber, G. Arya and S. Varghese, Proc. Natl. Acad. Sci. U. S. A., 2012, 109, 4383–4388 CrossRef CAS PubMed.
- X. Yu, L. Chen, M. Zhang and T. Yi, Chem. Soc. Rev., 2014, 43, 5346–5371 RSC.
- S. R. White, N. R. Sottos, P. H. Geubelle, J. S. Moore, M. R. Kessler, S. R. Sriram, E. N. Brown and S. Viswanathan, Nature, 2001, 409, 794–797 CrossRef CAS PubMed.
- B. Ghosh and M. W. Urban, Science, 2009, 323, 1458–1460 CrossRef CAS PubMed.
- Y. Amamoto, J. Kamada, H. Otsuka, A. Takahara and K. Matyjaszewski, Angew. Chem., Int. Ed., 2011, 123, 1698–1701 CrossRef PubMed.
- X. Chen, M. A. Dam, K. Ono, A. Mal, H. Shen, S. R. Nutt, K. Sheran and F. Wudl, Science, 2002, 295, 1698–1702 CrossRef CAS PubMed.
- P. Zheng and T. J. McCarthy, J. Am. Chem. Soc., 2012, 134, 2024–2027 CrossRef CAS PubMed.
- K. Imato, M. Nishihara, T. Kanehara, Y. Amamoto, A. Takahara and H. Otsuka, Angew. Chem., Int. Ed., 2012, 124, 1164–1168 CrossRef PubMed.
- K. K. Oehlenschlaeger, J. O. Mueller, J. Brandt, S. Hilf, A. Lederer, M. Wilhelm, R. Graf, M. L. Coote, F. G. Schmidt and C. Barner-Kowollik, Adv. Mater., 2014, 26, 3561–3566 CrossRef CAS PubMed.
- P. Cordier, F. Tournilhac, C. Soulie-Ziakovic and L. Leibler, Nature, 2008, 451, 977–980 CrossRef CAS PubMed.
- A. B. South and L. A. Lyon, Angew. Chem., Int. Ed., 2010, 122, 779–783 (Angew. Chem., Int. Ed., 2010, 49, 767–771) CrossRef PubMed.
- S. Burattini, B. W. Greenland, D. H. Merino, W. Weng, J. Seppala, H. M. Colquhoun, W. Hayes, M. E. Mackay, I. W. Hamley and S. J. Rowan, J. Am. Chem. Soc., 2010, 132, 12051–12058 CrossRef CAS PubMed.
- Q. Wang, J. L. Mynar, M. Yoshida, E. Lee, M. Lee, K. Okuro, K. Kinbara and T. Aida, Nature, 2010, 463, 339–343 CrossRef CAS PubMed.
- M. Burnworth, L. Tang, J. R. Kumpfer, A. J. Duncan, F. L. Beyer, G. L. Fiore, S. J. Rowan and C. Weder, Nature, 2011, 472, 334–337 CrossRef CAS PubMed.
- N. Holten-Andersen, M. J. Harrington, H. Birkedal, B. P. Lee, P. B. Messersmith, K. Y. C. Lee and J. H. Waite, Proc. Natl. Acad. Sci. U. S. A, 2011, 108, 2651–2655 CrossRef CAS PubMed.
- Y. Chen, A. M. Kushner, G. A. Williams and Z. Guan, Nat. Chem., 2012, 4, 467–472 CrossRef CAS PubMed.
- M. Zhang, D. Xu, X. Yan, J. Chen, S. Dong, B. Zheng and F. Huang, Angew. Chem., Int. Ed., 2012, 124, 7117–7121 CrossRef PubMed.
- C. C. Cheng, Y. C. Yen, Y. S. Ye and F. C. Chang, J. Polym. Sci., Part A: Polym. Chem., 2009, 47, 6388–6395 CrossRef CAS PubMed.
- I. H. Lin, C. C. Cheng, C. W. Huang, M. C. Liang, J. K. Chen, F. H. Ko, C. W. Chu, C. F. Huang and F. C. Chang, RSC Adv., 2013, 3, 12598–12603 RSC.
- I. H. Lin, C. C. Cheng, W. T. Chuang, J. K. Chen, U. S. Jeng, F. H. Ko, C. W. Chu, C. F. Huang and F. C. Chang, Soft Matter, 2013, 9, 9608–9614 RSC.
- C. C. Cheng, Y. C. Yen and F. C. Chang, Macromol. Rapid Commun., 2011, 32, 927–932 CrossRef CAS PubMed.
- C. C. Cheng, Y. C. Yen, F. H. Ko, C. W. Chu, S. K. Fan and F. C. Chang, J. Mater. Chem., 2012, 22, 731–734 RSC.
- L. Fielding, Tetraherdon, 2000, 56, 6151–6170 CrossRef CAS.
- A. Charlesby and N. H. Hancock, Proc. R. Soc. London, Ser. A, 1953, 218, 245–255 CrossRef CAS.
- J. Hentschel, A. M. Kushner, J. Ziller and Z. Guan, Angew. Chem., Int. Ed., 2012, 51, 10561–10565 CrossRef CAS PubMed.
- L. R. Hart, J. H. Hunter, N. A. Nguyen, J. L. Harries, B. W. Greenland, M. E. Mackay, H. M. Colquhoun and W. Hayes, Polym. Chem., 2014, 5, 3680–3688 RSC.
- S. J. George and A. Ajayaghosh, Chem.–Eur. J., 2005, 11, 3217–3227 CrossRef CAS PubMed.
- P. P. Hou, K. H. Gu, Y. F. Zhu, Z. Y. Zhang, Q. Wang, H. B. Pan, S. Yang, Z. Shen and X. H. Fan, RSC Adv., 2015, 5, 70163–70171 RSC.
Footnote |
† Electronic supplementary information (ESI) available: Experimental part including synthesis procedures, structural characterizations, variable-temperature NMR, and analytic methods. See DOI: 10.1039/c5ra19708b |
|
This journal is © The Royal Society of Chemistry 2015 |